DOI:
10.1039/D1SC01220G
(Edge Article)
Chem. Sci., 2021,
12, 9500-9505
Boosting cancer therapy efficiency via photoinduced radical production†
Received
2nd March 2021
, Accepted 14th June 2021
First published on 15th June 2021
Abstract
Current cancer therapy has been restricted by the hypoxic microenvironment of tumors, especially for strongly oxygen-dependent photodynamic therapy. To defeat tumor hypoxia, an oxygen-irrelevant radical nanogenerator, PI/FBC, is developed by the co-assembly of iodized polymer PI and NIR photosensitizer FBC, and further evaluated as a remote controllable free radical generation platform for enhancing antitumor efficiency. The PI/FBC radical nanogenerator can be excited by NIR light to produce ROS through transfer of energy to oxygen and induce the formation of toxic iodine radicals via electron transfer to PI. Notably, unlike conventional tumor treatments, such a radical nanogenerator is controllable and insusceptible to oxygen concentration. Moreover, benefiting from the strong NIR emission of FBC, the distribution of the PI/FBC radical nanogenerator can be monitored without incorporating other imaging agents. This PI/FBC radical nanogenerator treatment will no doubt broaden the family of antitumor strategies by using non-oxygen radicals, which is significant for reference in the development of promising anticancer agents.
Introduction
Hypoxia is a typical microenvironment feature in most solid tumors, resulting from the imbalance between oxygen supply and demand.1,2 A hypoxic microenvironment makes cancer cells resistant to cancer treatments, especially for oxygen-consuming therapies, for instance, photodynamic therapy.3–6 To enhance the therapeutic efficiency, great efforts have been dedicated to overcoming the bottleneck arising from hypoxia,7 such as increasing the oxygen concentration,8–10 reducing the oxygen consumption,11,12 or combining multiple therapeutic methods.13–20 Nano-delivery systems provide a promising potency in overcoming hypoxia of tumors, but face challenges such as poor repeatability and potentially adverse toxicity.21,22 Recently, hypoxia-tolerant photosensitizers have provided a powerful tool for hypoxic tumor treatment.23–26 These photosensitizers could produce toxic reactive oxygen species (ROS) such as superoxide (O2˙−), hydroxyl (⋅OH) and hydroperoxyl
through a photo-induced electron transfer mechanism. However, the produced toxic species are related to oxygen, indicating that the photodynamic therapeutic efficiency of these photosensitizers still relies on oxygen concentration although they require much lower oxygen concentration than traditional photosensitizers.27 To defeat tumor hypoxia, it would be promising to develop an entirely oxygen-independent platform with remote controllable ability.
Oxygen-irrelevant free radicals are also highly reactive species, which have been extensively employed to synthesize compounds and polymers.28–31 Benefiting from their efficient generation even under a hypoxic environment, radical nanogenerators based on free radical initiators have been developed to overcome tumor hypoxia for amplifying antitumor efficiency.32–36 Aside from these radical nanogenerators which focus mainly on thermolabile radical generators, production of free radicals via a photo-redox reaction may be another promising approach for hypoxic tumor treatment. Photo-induced radical reactions with excellent controllability have been an appealing option for catalytic organic synthesis and controllable polymerization.37–40 For cancer therapy, Bu and his co-workers developed a ⋅Cl nano-generator by decorating a SiO2-coated upconversion nanoparticle (UCNP) with Ag0/AgCl hetero-dots outside, in which ⋅Cl generation can be triggered by the short wavelength emission of the UCNP upon NIR illumination.41 In consideration of the energy conversion efficiency, it is a great advantage for efficient oxygen-irrelevant radical formation directly via a NIR photosensitizer upon illumination.
Herein, we develop a biocompatible free radical nanogenerator (PI/FBC) by the co-assembly of iodized block copolymer POEGMA-b-PIEMA (PI) and tetrafluorophenyl bacteriochlorin (FBC) photosensitizer with strong NIR absorbance for hypoxia-irrelevant phototherapy against tumor hypoxia. As shown in Scheme 1, FBC as a NIR photosensitizer can not only produce ROS under NIR illumination, but also induce the production of highly cytotoxic iodine free radicals, endowing the PI/FBC nanogenerator with superior remote controllability. Moreover, iodide as the radical initiator was installed on the polymer chain by reversible addition–fragmentation chain transfer (RAFT) polymerization, avoiding unpredictable side effects suffered from the leakage of initiators. Besides, POEGMA as the hydrophilic block can form a hydrophilic shell on the surface of the PI/FBC nanogenerator, effectively improving the biocompatibility of the PI/FBC radical nanogenerator and extending the circulation time. More importantly, the strong NIR absorption of FBC is beneficial to realize deep penetration against skin tissue without severe side effects. Thus, the PI/FBC radical nanogenerator may lead to the new trend of free radical-induced tumor therapy, providing a reference for the development of non-oxygen free radicals for future hypoxia antitumor therapy.
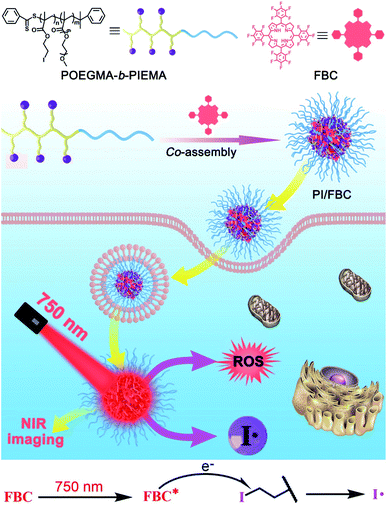 |
| Scheme 1 Preparation of the PI/FBC radical nanogenerator and its antitumor mechanism upon NIR irradiation. Iodine radicals were produced by electron transfer from FBC to PI under NIR irradiation. | |
Results and discussion
Synthesis of PI/FBC nanoparticles
NIR absorption photosensitizer FBC was firstly synthesized according to our previous work (Fig. S1, ESI†).26 Then, iodine-containing monomer 2-iodoethyl methacrylate was prepared via an esterification reaction between methacryloyl chloride and 2-iodoethanol (Fig. S2, ESI†).42,43 Before POEGMA-b-PIEMA was synthesized through RAFT polymerization, a POEGMA macro-RAFT agent was prepared by the homopolymerization of OEGMA according to the route as illustrated in Scheme S1 (ESI†). The successful synthesis of POEGMA and POEGMA-b-PIEMA was confirmed by 1H NMR spectroscopy (Fig. S3 and S4, ESI†) and gel permeation chromatography (GPC, Fig. S5, ESI†), respectively. Afterwards, PI/FBC nanoparticles were fabricated by the co-assembly of POEGMA-b-PIEMA and FBC. The transmission electron microscopy (TEM) image shown in Fig. 1a reveals the well-defined spherical morphology of PI/FBC nanoparticles. The hydrodynamic size distribution of PI/FBC nanoparticles was determined by dynamic lighting scattering (DLS, Fig. 1b). Compared with PI nanoparticles (directly formed by the self-assembly of POEGMA-b-PIEMA block polymers), the size of the PI/FBC nanoparticles did not increase but decreased slightly after FBC was incorporated, which may have resulted from the more concentrated hydrophobic moieties of FBC. The zeta potential value of PI/FBC nanoparticles was below −10 mV in comparison to that of PI nanoparticles, which not only suggested the successful encapsulation of FBC, but also showed good colloidal stability as displayed in Fig. 1c. The photophysical properties of PI/FBC nanoparticles were evaluated by utilizing UV-vis and fluorescence spectrophotometers (Fig. 1d). The UV-vis spectra of PI/FBC nanoparticles indicated that FBC was successfully encapsulated in the nanoparticles, in agreement with the DLS and zeta potential results. Besides, owing to the efficient encapsulation of FBC, the strong NIR emission of FBC can also be observed in the fluorescence spectrum of PI/FBC nanoparticles, endowing PI/FBC nanoparticles with excellent imaging performance. Additionally, iodine-free PC/FBC nanoparticles as the control were prepared by encapsulating FBC with a block copolymer, poly(ethylene glycol)-block-poly(lactide acid) (PEG-b-PLA), and the detailed characterization is shown in Fig. S6–S8 (ESI†).
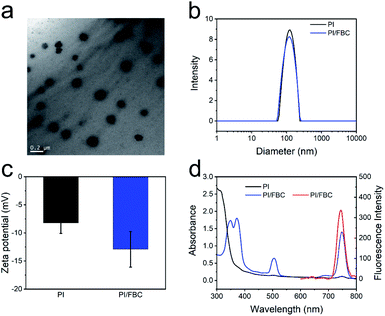 |
| Fig. 1 Characterization of the prepared nanoparticles. (a) Representative TEM image of PI/FBC nanoparticles. (b) Size distribution of nanoparticles determined by dynamic light scattering. (c) Zeta potential of PI and PI/FBC nanoparticles. (d) UV-vis absorption and fluorescence spectra of PI/FBC nanoparticles. | |
Reactive species production of PI/FBC nanoparticles
PI/FBC nanoparticles were expected to produce both reactive oxygen species and iodine free radicals upon NIR illumination (Fig. 2a). And then we determined the extracellular reactive species (RS) production of PI/FBC nanoparticles before in vitro antitumor performance. DPBF as a total ROS probe was employed to measure the ROS production of PI/FBC nanoparticles under NIR irradiation.44 As shown in Fig. 2b, S9 and S10 (ESI†), PI/FBC and PC/FBC nanoparticles can both cause the absorbance decrease of DPBF with the irradiation time extension, indicating that a significant amount of ROS was generated by the nanoparticles. It is noteworthy that the absorbance decrease of DPBF of PI/FBC nanoparticles was modest compared to that of PC/FBC nanoparticles, which may be induced by the iodine free radical production of PI/FBC nanoparticles. The iodine free radical production competes with the ROS generation to consume excited photosensitizers, resulting in the decrease of ROS formation. To verify the hypothesis, 2,2′-amino-di(2-ethyl-benzothiazoline sulphonic acid-6) ammonium salt (ABTS) as a radical probe was adopted to determine the production of iodine free radicals.35 We can observe that the UV-vis spectrum of ABTS of PC/FBC nanoparticles changed slightly after NIR illumination (Fig. 2c); however, a significant increase was found in that of PI/FBC nanoparticles, indicating that a mass of iodine radicals was produced by PI/FBC nanoparticles (Fig. 2d). To further confirm the production of free radicals, we also used a radical trapping agent, TEMPO, to capture the produced iodine free radical under NIR illumination (Fig. S11, ESI†). The peak at 2.8 ppm increased with irradiation time extension, suggesting the successful formation of iodine radicals.
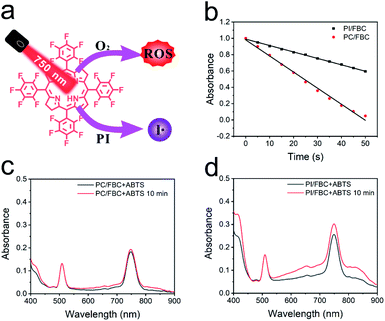 |
| Fig. 2
In vitro reactive species production. (a) Illustration of the generation mechanism of reactive species. (b) Changes in the absorption of DPBF at 425 nm induced by PC/FBC and PI/FBC nanoparticles under illumination. Free radical production of (c) PC/FBC and (d) PI/FBC nanoparticles determined by using ABTS as a radical probe, respectively. | |
Intracellular uptake and photoactivity
Encouraged by the exciting extracellular consequence, in vitro antitumor performance was evaluated on 4T1 cells. A confocal laser scanning microscope (CLSM) was employed to determine the cellular internalization and cytotoxic species production of nanoparticles. As shown in Fig. 3a, bright red fluorescence can be observed around the cell nucleus (blue fluorescence), indicating that nanoparticles can efficiently enter cancer cells. Subsequently, 2,7-dichlorofluorescein diacetate (DCFH-DA), a fluorescent probe, was used to determine the production of oxidizing toxic species as shown in Fig. 3b. Compared to PI and PC/FBC nanoparticles, strong green fluorescence of DCF generated via oxidation of reactive species to DCFH-DA was detected after treatment with NIR irradiation in the presence of PI/FBC nanoparticles, indicating that the DCF formation resulted from the toxic species produced by PI/FBC nanoparticles after NIR irradiation. There was almost no green fluorescence in PI nanoparticle treated cells with NIR illumination, which may reveal that PI nanoparticles are biocompatible.
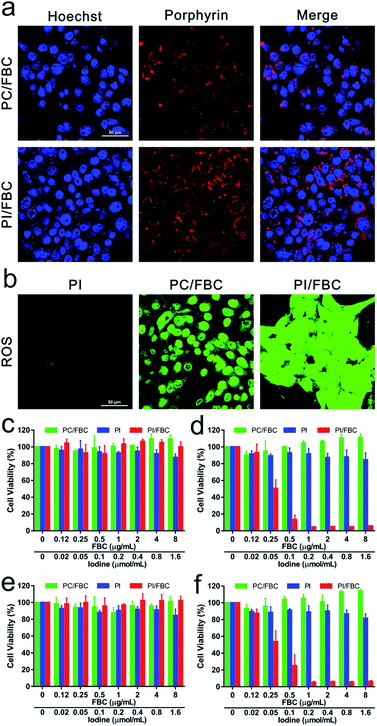 |
| Fig. 3
In vitro antitumor performance. (a) Cellular uptake of PI/FBC and PC/FBC nanoparticles measured using a confocal laser scanning microscope (scale bar: 50 μm). (b) CLSM images of intracellular reactive species (RS) induced by PI/FBC and PC/FBC nanoparticles (scale bar: 50 μm). (c–f) Relative viabilities of 4T1 cells incubated with a series of concentrations of PI/FBC and PC/FBC nanoparticles. (c) Dark toxicity and (d) phototoxicity under normoxic conditions. (e) Dark toxicity and (f) phototoxicity under hypoxic conditions (750 nm, 50 mW cm−2, 10 min). | |
MTT assay was employed to further evaluate the antitumor efficiency under normal and hypoxic conditions (FBC concentration: 0–8 μg mL−1) (Fig. 3c–f). From Fig. 3c and e, it can be observed that there is no significant cytotoxicity against cells for PI, PC/FBC and PI/FBC nanoparticles, indicating that all the nanoparticles have good biocompatibility. It is noteworthy that, with NIR irradiation (750 nm, 50 mW cm−2, 10 min), PI/FBC nanoparticles could efficiently induce cell death in a concentration-dependent manner, even in a hypoxic environment (Fig. 3d and f). However, the PC/FBC and PI nanoparticle treated cells did not end their days obviously. These results may be associated with the modest or disabled production of toxic species of PC/FBC or PI nanoparticles, respectively, which is in line with the foregoing consequence of intracellular DCF fluorescence detected by CLSM. In addition, we also determined the cytotoxicity of PC/FBC nanoparticles at a higher FBC concentration (0–32 μg mL−1) under an elevated laser power density (100 mW cm−2, 15 min) (Fig. S12, ESI†). The results revealed that the cell survival decreased with the increase of PC/FBC concentration, which further confirmed that the high cell survival of PC/FBC in the previous experiment resulted from the limited ROS generation.
In vivo imaging and biodistribution, photodynamic therapy and bio-safety
Based on the remarkable in vitro photo-induced toxicity against cancer cells, an in vivo performance study was carried out on nude mice bearing tumors. 4T1 tumor-bearing mice were obtained by subcutaneously injecting 200 μL of 5 × 106 tumor cells. For in vivo distribution monitoring via fluorescence imaging, PI/FBC nanoparticles were injected into mice via the tail vein after the xenograft tumor volume reached 100 mm3. The fluorescence imaging was monitored in real time via detecting the emission at 750 nm after FBC was excited with 510 nm light. As shown in Fig. 4a and S13 (ESI†), the fluorescence signal at the tumor gradually increased with time extension, suggesting that PI/FBC could be efficiently enriched in tumors by the enhanced permeability and retention (EPR) effect of tumors.45 The biodistribution results also distinctly demonstrated that PI/FBC nanoparticles were primarily accumulated in the tumor site 24 h post-injection.
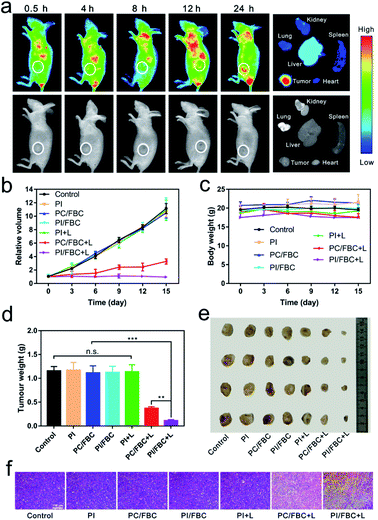 |
| Fig. 4
In vivo photo-induced inhibition test against the 4T1 tumor. (a) Changes of in vivo distribution of PI/FBC nanoparticles with the time extension monitored by fluorescence imaging. (b) Relative tumor volume and (c) the mice body weight during treatment. (d) Tumor weights and (e) photograph of isolated tumors after 15 days of treatment (n = 4). (f) Images of a tumor section stained with H&E (scale bar: 150 μm, *P < 0.05, **P < 0.01, and ***P < 0.001). | |
To further verify the antitumor efficiency of PI/FBC nanoparticles, mice bearing 4T1 tumors were randomly divided into seven groups (control, PI, PC/FBC, PI/FBC, PI + L, PC/FBC + L and PI/FBC + L) and separately administered with the corresponding nanoparticles through the tail vein when the tumor volume reached 100 mm3. Tumors in PI + L, PC/FBC + L and PI/FBC + L groups were irradiated with a 750 nm laser for 15 min at a power density of 200 mW cm−2. Tumor volumes and body weights were recorded to evaluate the tumor suppression effect and biocompatibility of nanoparticles, respectively (Fig. 4b and c). We found that PC/FBC and PI/FBC nanoparticles could significantly inhibit the tumor growth after illumination with a 750 nm laser. It is noteworthy that PI/FBC exhibited a superior inhibition efficiency than PC/FBC, which could be attributed to the oxygen-irrelevant iodine free radicals generated by PI/FBC nanoparticles under illumination. However, PI nanoparticles did not show any antitumor effect, indicating that the oxygen-irrelevant free radical production was triggered by FBC under 750 nm laser irradiation. Without laser illumination, PI, PC/FBC and PI/FBC nanoparticles all showed no obvious difference compared to the control, revealing that they had a good biocompatibility. No significant weight loss was observed in Fig. 4c, suggesting minimal side effects of the nanoparticles. On 15th day, the mice were sacrificed to harvest tumors and primary organs (heart, liver, spleen, lungs and kidneys) for further evaluation of antitumor efficiency. Isolated tumors were weighed and photographed as depicted in Fig. 4d and e. PI/FBC + L treated tumors had the lightest weight and smallest volume, indicating that PI/FBC nanoparticles could efficiently inhibit the tumor growth after illumination with a 750 nm laser. H&E staining on tissue sections (Fig. 4f and S14, ESI†) also revealed that PI/FBC nanoparticles are highly biocompatible with major organs and could cause significant cell necrosis after being triggered by 750 nm laser irradiation.
Conclusions
In conclusion, a biocompatible hypoxia-tolerant nano-system, PI/FBC, was fabricated by co-assembling an iodized polymer with a NIR photosensitiser, FBC. PI/FBC nanoparticles could efficiently accumulate in tumor sites through the EPR effect, which can be monitored in real time via NIR fluorescence imaging. Upon being excited with an NIR laser, PI/FBC nanoparticles could not only produce cytotoxic ROS by transferring energy to oxygen, but also cause highly toxic iodine free radical production through an oxygen-irrelevant mechanism. Therefore, PI/FBC nanoparticles could facilitate a significantly enhanced antitumor efficiency by conquering the hypoxic tumor environment in a controllable manner. This PI/FBC radical nanogenerator treatment will no doubt broaden the family of antitumor strategies by using nonoxygen free radicals, which is significant for reference in the development of prospective anticancer agents.
Data availability
The electronic supplementary information include experimental detail, NMR data, spectra data and histological data.
Author contributions
Z. Liu conceived the project and designed the experiments. Z. liu and M. Wu performed the sample preparation, characterization and biological experiment. Z. Liu and M. Wu wrote the paper and contributed equally. All authors discussed the results and commented on the manuscript.
Ethical statement
All animal studies were conducted on male Balb/c nude mice (four to five weeks) in compliance with the National Institutes of Health Guide for the Care and Use of Laboratory Animals approved by the Animal Care and Use Committee of East China University of Science and Technology.
Conflicts of interest
There are no conflicts to declare.
Acknowledgements
This work was financially supported by the National Natural Science Foundation of China (No. 22075079 and 21875063) and the Science and Technology Commission of Shanghai Municipality for the Shanghai International Cooperation Program (19440710600).
References
- W. R. Wilson and M. P. Hay, Nat. Rev. Cancer, 2011, 11, 393–410 CrossRef CAS PubMed.
- J. M. Brown and W. R. Wilson, Nat. Rev. Cancer, 2004, 4, 437–447 CrossRef CAS PubMed.
- T. M. Ashton, E. Fokas, L. A. Kunz-Schughart, L. K. Folkes, S. Anbalagan, M. Huether, C. J. Kelly, G. Pirovano, F. M. Buffa, E. M. Hammond, M. Stratford, R. J. Muschel, G. S. Higgins and W. G. McKenna, Nat. Commun., 2016, 7, 12308 CrossRef CAS PubMed.
- K. Graham and E. Unger, Int. J. Nanomed., 2018, 13, 6049–6058 CrossRef CAS PubMed.
- X. Jing, F. Yang, C. Shao, K. Wei, M. Xie, H. Shen and Y. Shu, Mol. Cancer, 2019, 18, 157 CrossRef PubMed.
- A. Sahu, I. Kwon and G. Tae, Biomaterials, 2020, 228, 119578 CrossRef CAS PubMed.
- C. D. Phung, T. H. Tran, L. M. Pham, H. T. Nguyen, J. H. Jeong, C. S. Yong and J. O. Kim, J. Controlled Release, 2020, 324, 413–429 CrossRef CAS PubMed.
- S. C. Zhang, Q. Z. Li, N. Yang, Y. H. Shi, W. Ge, W. J. Wang, W. Huang, X. J. Song and X. C. Dong, Adv. Funct. Mater., 2019, 29, 1906805 CrossRef CAS.
- L. Xing, J. H. Gong, Y. Wang, Y. Zhu, Z. J. Huang, J. Zhao, F. Li, J. H. Wang, H. Wen and H. L. Jiang, Biomaterials, 2019, 206, 170–182 CrossRef CAS PubMed.
- Z. Liu, Y. Xue, M. Wu, G. Yang, M. Lan and W. Zhang, Biomacromolecules, 2019, 20, 4563–4573 CrossRef CAS PubMed.
- W. Lv, Z. Zhang, K. Y. Zhang, H. Yang, S. Liu, A. Xu, S. Guo, Q. Zhao and W. Huang, Angew. Chem., Int. Ed., 2016, 55, 9947–9951 CrossRef CAS PubMed.
- M. Li, Y. Shao, J. H. Kim, Z. Pu, X. Zhao, H. Huang, T. Xiong, Y. Kang, G. Li, K. Shao, J. Fan, J. W. Foley, J. S. Kim and X. Peng, J. Am. Chem. Soc., 2020, 142, 5380–5388 CrossRef CAS PubMed.
- L. H. Fu, C. Qi, Y. R. Hu, J. Lin and P. Huang, Adv. Mater., 2019, 31, 1808325 CrossRef PubMed.
- D. Cui, J. Huang, X. Zhen, J. Li, Y. Jiang and K. Pu, Angew. Chem., Int. Ed., 2019, 58, 5920–5924 CrossRef CAS PubMed.
- X. Zhao, S. Long, M. Li, J. Cao, Y. Li, L. Guo, W. Sun, J. Du, J. Fan and X. Peng, J. Am. Chem. Soc., 2020, 142, 1510–1517 CrossRef CAS PubMed.
- Y. Jiang, X. Zhao, J. Huang, J. Li, P. K. Upputuri, H. Sun, X. Han, M. Pramanik, Y. Miao, H. Duan, K. Pu and R. Zhang, Nat. Commun., 2020, 11, 1857 CrossRef CAS PubMed.
- Y. Jiang, J. Li, Z. Zeng, C. Xie, Y. Lyu and K. Pu, Angew. Chem., Int. Ed., 2019, 58, 8161–8165 CrossRef CAS PubMed.
- J. Li, D. Cui, Y. Jiang, J. Huang, P. Cheng and K. Pu, Adv. Mater., 2019, 31, 1905091 CrossRef CAS PubMed.
- Z. Zeng, C. Zhang, J. Li, D. Cui, Y. Jiang and K. Pu, Adv. Mater., 2021, 33, 2007247 CrossRef CAS PubMed.
- S. He, Y. Jiang, J. Li and K. Pu, Angew. Chem., Int. Ed., 2020, 59, 10633–10638 CrossRef CAS PubMed.
- K. Cho, X. Wang, S. Nie, Z. G. Chen and D. M. Shin, Clin. Cancer Res., 2008, 14, 1310–1316 CrossRef CAS PubMed.
- D. Sun, S. Zhou and W. Gao, ACS Nano, 2020, 14, 12281–12290 CrossRef CAS PubMed.
- T. Luo, K. Ni, A. Culbert, G. Lan, Z. Li, X. Jiang, M. Kaufmann and W. Lin, J. Am. Chem. Soc., 2020, 142, 7334–7339 CrossRef CAS PubMed.
- K. Zhang, Z. Yu, X. Meng, W. Zhao, Z. Shi, Z. Yang, H. Dong and X. Zhang, Adv. Sci., 2019, 6, 1900530 CrossRef PubMed.
- L. Li, C. Shao, T. Liu, Z. Chao, H. Chen, F. Xiao, H. He, Z. Wei, Y. Zhu, H. Wang, X. Zhang, Y. Wen, B. Yang, F. He and L. Tian, Adv. Mater., 2020, 32, 2003471 CrossRef CAS PubMed.
- M. Wu, Z. Liu and W. Zhang, Chem. Sci., 2021, 12, 1295–1301 RSC.
- S. Kuang, L. Sun, X. Zhang, X. Liao, T. W. Rees, L. Zeng, Y. Chen, X. Zhang, L. Ji and H. Chao, Angew. Chem., Int. Ed., 2020, 59, 20697–20703 CrossRef CAS PubMed.
- M. J. Black, K. F. Biegasiewicz, A. J. Meichan, D. G. Oblinsky, B. Kudisch, G. D. Scholes and T. K. Hyster, Nat. Chem., 2020, 12, 71–75 CrossRef PubMed.
- J. Sinha, B. D. Fairbanks, H. B. Song and C. N. Bowman, ACS Macro Lett., 2019, 8, 213–217 CrossRef CAS.
- P. Li, J. Zhao, L. Shi, J. Wang, X. Shi and F. Li, Nat. Commun., 2018, 9, 1972 CrossRef PubMed.
- J. Han and J. Xie, Chem, 2020, 6, 1053–1055 CAS.
- X. Q. Wang, F. Gao and X. Z. Zhang, Angew. Chem., Int. Ed., 2017, 56, 9029–9033 CrossRef CAS PubMed.
- S. Shen, C. Zhu, D. Huo, M. Yang, J. Xue and Y. Xia, Angew. Chem., Int. Ed., 2017, 56, 8801–8804 CrossRef CAS PubMed.
- X. M. Li, Y. Liu, F. Fu, M. B. Cheng, Y. T. Liu, L. C. Yu, W. Wang, Y. D. Wan and Z. Yuan, Nano-Micro Lett., 2019, 11, 68 CrossRef CAS PubMed.
- H. Xiang, H. Lin, L. Yu and Y. Chen, ACS Nano, 2019, 13, 2223–2235 CAS.
- Y. P. Wan, G. H. Lu, J. F. Zhang, Z. Y. Wang, X. Z. Li, R. Chen, X. Cui, Z. M. Huang, Y. F. Xiao, J. Chelora, W. J. Zhang, Y. H. Liu, M. Li, H. Y. Xie and C. S. Lee, Adv. Funct. Mater., 2019, 29, 1903436 CrossRef.
- L. Wang, J. M. Lear, S. M. Rafferty, S. C. Fosu and D. A. Nagib, Science, 2018, 362, 225–229 CrossRef CAS PubMed.
- M. C. Fu, R. Shang, B. Zhao, B. Wang and Y. Fu, Science, 2019, 363, 1429–1434 CrossRef CAS PubMed.
- A. E. Bosnidou and K. Muniz, Angew. Chem., Int. Ed., 2019, 58, 7485–7489 CrossRef CAS PubMed.
- H. L. Cao, G. C. Wang, Y. D. Xue, G. L. Yang, J. Tian, F. Liu and W. A. Zhang, ACS Macro Lett., 2019, 8, 616–622 CrossRef CAS.
- R. Song, H. Wang, M. Zhang, Y. Liu, X. Meng, S. Zhai, C. C. Wang, T. Gong, Y. Wu, X. Jiang and W. Bu, Angew. Chem., Int. Ed., 2020, 59, 21032–21040 CrossRef CAS PubMed.
- Y. H. Chang, P. Y. Lin, M. S. Wu and K. F. Lin, Polymer, 2012, 53, 2008–2014 CrossRef CAS.
- G. Amitai, H. Murata, J. D. Andersen, R. R. Koepsel and A. J. Russell, Biomaterials, 2010, 31, 4417–4425 CrossRef CAS PubMed.
- T. Entradas, S. Waldron and M. Volk, J. Photochem. Photobiol., B, 2020, 204, 111787 CrossRef CAS PubMed.
- D. Peer, J. M. Karp, S. Hong, O. C. Farokhzad, R. Margalit and R. Langer, Nat. Nanotechnol., 2007, 2, 751–760 CrossRef CAS PubMed.
Footnotes |
† Electronic supplementary information (ESI) available. See DOI: 10.1039/d1sc01220g |
‡ These authors contributed equally. |
|
This journal is © The Royal Society of Chemistry 2021 |
Click here to see how this site uses Cookies. View our privacy policy here.