DOI:
10.1039/D1SC00846C
(Edge Article)
Chem. Sci., 2021,
12, 7401-7410
Bright luminescent lithium and magnesium carbene complexes†‡
Received
10th February 2021
, Accepted 15th April 2021
First published on 15th April 2021
Abstract
We report on the convenient synthesis of a CNC pincer ligand composed of carbazole and two mesoionic carbenes, as well as the corresponding lithium- and magnesium complexes. Mono-deprotonation affords a rare “naked” amide anion. In contrast to the proligand and its mono-deprotonated form, tri-deprotonated s-block complexes show bright luminescence, and their photophysical properties were therefore investigated by absorption- and luminescence spectroscopy. They reveal a quantum yield of 16% in solution at ambient temperature. Detailed quantum-chemical calculations assist in rationalizing the emissive properties based on an Intra-Ligand-Charge-Transfer (ILCT) between the carbazolido- and mesoionic carbene ligands. (Earth-)alkali metals prevent the distortion of the ligand following excitation and, thus, by avoiding non-radiative deactivation support bright luminescence.
Introduction
Carbazole-based dyes have a rich history as photo-sensitizers,1–3 photo-initiators and -catalysts,4–7 host materials for Organic Light-Emitting Diodes (OLEDs),8,9 triplet emitters,10,11 and Thermally Activated Delayed Fluorescence (TADF)12–18 materials. Recent remarkable achievements comprise the emissive properties of two-coordinate coinage metal complexes.19–32 There, embedding copper(I) in a push–pull electronic environment provided by a π-acidic carbene33 and a π-donating carbazolido ligand (Fig. 1, I), resulted in a 100% quantum yield of the 474 nm luminescence and an excited state lifetime of 2.8 μs.22 The surprising efficiency of these “carbene-metal-amido” complexes relies on a prompt Reverse Inter System Crossing (RISC) mechanism.34–36 Furthermore, the donor–bridge–acceptor substitution pattern, as it is known from organic dyes, enhances the transition probability, and thus favors fluorescence over non-radiative decay channels.37 We became interested in carbazolyl bridged pincer-type NHC ligands38–41 as promising candidates to stabilize multiple bonded late transition metal complexes.42–44 Similar ligands, which had been studied mainly as NNN pincers (Fig. 1, II),45–57 have been pioneered by Bezuidenhout58–62 (CNC pincer, C: mesoionic carbene MIC; III and IV) and Kunz63–68 (CNC pincer, C: N-heterocyclic carbene NHC; V). Mesoionic carbenes,69–75 in general, and 1,2,3-triazolinylidenes, in particular, excel through their donating properties76,77 and, as such, are expected to stabilize high-valent transition metals.
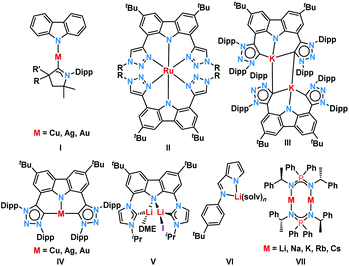 |
| Fig. 1 Previously studied related complexes. | |
Despite the fact that they are much less popular than conventional NHCs42 and less explored in photochemistry, they increasingly attract attention.52,78–89 During our initial coordination experiments with the 1,2,3-triazolinylidene decorated carbazolide obtained by deprotonation of 3, we noticed strong luminescence upon deprotonation (vide infra). Intriguingly, Kunz had already noticed luminescence for lithium carbazolyl bridged dicarbenes, however, these systems have not been studied spectroscopically yet (Fig. 1, III and V).59,63,64,90 Bezuidenhout and co-workers reported the photochemical properties of T-shaped and linear coinage metal complexes (Fig. 1, IV).62 They found that the luminescence wavelength is tunable by the judicious choice of the metal. Thereby, the copper(I) complex emitted in the blue- (quantum yield Φem = 0.8%), the silver(I) in the orange- (Φem = 2.4%), and the gold(I) complex in the green- (Φem = 0.6%) regions of the spectrum. The protonated proligand showed emissive properties as well (green, Φem = 2.0%). Heinze et al.91 described, for example, “alkali-blue” emissive pyrrolates (Fig. 1, VI). A quantum yield of 1% was achieved through an efficient ILCT (Intra-Ligand-Charge-Transfer) thanks to the templating effect of the alkali metals.92 Blue luminescence was also observed by Roesky et al. in the case of iminophosphonamide alkali metal complexes (Fig. 1, VII) with a solid-state quantum yield of up to 36%.93 Agapie and co-workers introduced lithium bridged dipyridyl dipentacene pyrrolates as efficient singlet fission molecules.94
Inspired by these reports and the surge of interest in photochemistry with complexes of earth-abundant metals,95 we report herein a carbazolide bridged mesoionic biscarbene pincer ligand and a detailed investigation on the luminescent properties of its (earth-)alkali complexes. These complexes show excellent quantum yields of up to 16% at ambient temperatures in solution. Using quantum chemical calculations, we elucidate the effects of rigidity and planarity on the luminescence quantum yield and the excitation/luminescence wavelengths.
Results and discussion
Proligand synthesis
Searching for an alternate route to design tridentate carbazole-triazolylidene ligands, avoiding the use of potentially hazardous and explosive tert-butylhypochloride as suggested by Bezuidenhout and co-workers,59 we initially examined the alkylation of classical triazoles, as has been reported by Limberg, Hecht and Brooker.45,51 This strategy proved also successful in case of carbenaporphyrins.90 In the present case, neither the use of methyl iodide nor Meerwein's salt (triethyloxonium tetrafluoroborate) or methyl triflate yielded the desired carbazole-bistriazolium salts in reasonable yields. Instead, mixtures with predominantly N-carbazole-methylation were observed.
To avoid the undesired N-carbazole alkylation, we consequently adopted an intramolecular alkylation strategy (Scheme 1).96 The synthesis of 1 was achieved following nitration, reduction, and azotation of commercially available 3,6-di-tert-butylcarbazole.51 Using standard CuAAC (Cu-catalyzed Azide–Alkyne Cycloaddition) conditions with 6-chlorohex-1-yne led to clean 2. The formation of the product was apparent from the disappearance of the characteristic azide stretching resonance at 2099 cm−1 (Fig. S1†) and by the characteristic low-field 1H NMR resonance at δ = 7.98 ppm indicative for a triazole heterocycle (Fig. S2†). Adding excess of potassium iodide and heating the mixture in acetonitrile to reflux for two days gave the N-fused triazolium salt 3 in essentially quantitative yields. The cyclization was evident by several features in the NMR spectra, namely (i) the low-field shift of the triazolium-5H resonance in the 1H NMR spectrum from δ = 7.98 ppm (2) to δ = 8.91 ppm (3), (ii) the low-field shift of the methylene protons' resonance of the former –CH2Cl group from δ = 3.64 ppm (2) to δ = 4.79 ppm (3), and (iii) the coupling of this methylene group to one of the triazolin nitrogen atoms according to two-dimensional 1H–15N HMBC spectra (Fig. S6 and S11†). X-ray quality crystals97 of 3 were obtained by slow evaporation of a saturated chloroform solution (Fig. 2). The triazolium salt 3 crystallized with four strongly disordered molecules of chloroform in the lattice in the orthorhombic space group Pbcm with half a molecule of 3 in the asymmetric unit.
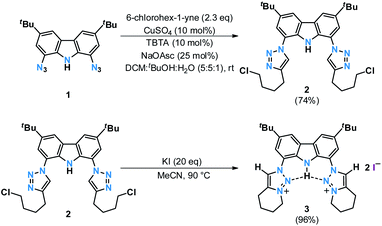 |
| Scheme 1 Proligand synthesis via intramolecular cyclization. TBTA = tris[(1-benzyl-1H-1,2,3-triazol-4-yl)methyl]amine, NaOAsc = sodium ascorbate. | |
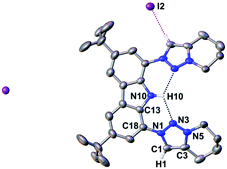 |
| Fig. 2 Compound 3 forms intramolecular hydrogen bonds in the solid-state. Thermal ellipsoids are shown at the 50% probability level; hydrogen atoms (except the ones bonded to the carbazole's nitrogen atom and the triazolium heterocycles) are omitted for clarity. Selected bond lengths [Å], angles [°], and dihedral angle [°]: N10–H10 0.88(2), N10–C13 1.391(6), C18–N1 1.433(7), N1–N3 1.326(6), N3–N5 1.311(6), C1–C3 1.353(8), N3–H10 2.351(19), C13–N10–C13 107.0(6), N1–C1–C3 105.8(5), C13–C18–N1–N3 29.2(7). | |
The structural metrics of the dication in 3 (Table S3†) resemble those of previously reported triazolium salts.98,99 In the solid-state structure of 3, strong hydrogen bonding interactions between the central triazolium nitrogen atoms N3 and the carbazole proton H10 were observed. Due to steric bulk, these interactions are not feasible for the other reported MIC-CNC pincer ligands (Fig. 1III and IV), but have been observed in a bis(pyrazolyl)carbazole derivative.100 Additionally, weak hydrogen bonding between H10–I2 and H1–I2 were observed in the solid-state structure of 3 (Fig. S29†).
Complex synthesis
The reaction of proligand 3 with one equivalent of lithium hexamethyl disilazide [LiHMDS; LiN(SiMe3)2] led to deprotonation of the carbazole (4, Scheme 2). Evidence for the latter came from the disappearance of the resonance for the carbazole N–H group in the 1H NMR spectrum (Fig. S12†). X-ray quality crystals of deep-orange and air-stable 4, which crystallized in the P
space group, were obtained by diffusion of hexane into a THF/benzene solution (Fig. 3). Notably, the carbazolide in 4 does not coordinate a lithium cation. Instead, the latter precipitated from the reaction mixture in the form of lithium iodide. Compound 4, therefore, contains a rare “naked” amide anion, as was also corroborated by calculations (Fig. S31†).101–103 The structure in the solid-state reveals a weak hydrogen bond [2.397(2) Å] between N10 and H2, which might be the reason for the surprising stability of 4 towards moisture. Proligand 3 was also deprotonated thrice by three equivalents of LiHMDS, as was confirmed by the 1H NMR spectroscopic analysis of Li5 (Fig. S14†). The 7Li NMR of Li5 showed a signal at δ = −1.43 ppm, which corroborates the presence of lithium cations (Fig. S16†). Immediately upon deprotonation of 3, strong blue luminescence was observed (vide infra) even in dim light. X-ray quality crystals of Li5 could be obtained by slow diffusion of pentane into a diethyl ether solution of the complex (Fig. 4A). Li5 crystallized in the monoclinic space group P21/n. It has a dimeric structure in the solid-state with bridging μ-iodo ligands and includes lithium iodide adducts. Thereby, the Li2–I1 and Li3–I2 units could be understood as lithium iodide molecules.
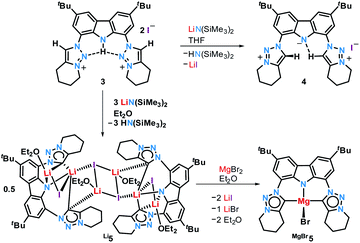 |
| Scheme 2 Deprotonation of 3 to 4 and Li5 and subsequent transmetalation to MgBr5. | |
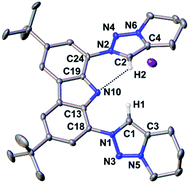 |
| Fig. 3 Compound 4 is devoid of N-coordinated lithium in the solid-state. Thermal ellipsoids are shown at the 50% probability level; hydrogen atoms (except the ones bonded to the triazolium heterocycles) are omitted for clarity. Selected bond lengths [Å], angles [°], and dihedral angles [°]: N10–C13 1.373(4), N10–C19 1.368(6), C24–N2 1.434(4), N2–C2 1.351(4), C2–C4 1.361(5), C4–N6 1.354(4), N6–N4 1.322(4), N4–N2 1.335(4), N10–H2 2.397(2), N10–H1 2.909(4), C18–N1 1.437(4), N1–N3 1.324(4), N5–N3 1.324(4), N5–C3 1.351(5), C3–C1 1.361(5), C13–N10–C19 103.1(3), N2–C2–C4 106.0(3), N1–C1–C3 105.7(3), C13–C18–N1–N3 −137.7(4), C19–C24–N2–N4 162.9(4). | |
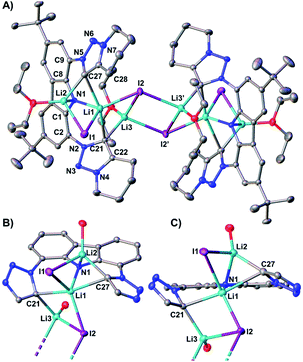 |
| Fig. 4 (A) The molecular structure of dimeric Li5 contains six lithium atoms and four bridging iodo ligands. Thermal ellipsoids are shown at the 50% probability level; hydrogen atoms are omitted for clarity. The monomers are shown from different views in (B) and (C), for which the annulated six-membered rings, tBu groups, ethyl fragments of the diethyl ether and all hydrogen atoms have been omitted for clarity. Selected bond lengths [Å], angles [°], and dihedral angles [°]: N1–C1 1.3837(19), N1–C8 1.3899(19), C9–N5 1.4302(19), N5–N6 1.3396(18), N6–N7 1.3251(19), N7–C28 1.356(2), C28–C27 1.392(2), C27–N5 1.373(2), C27–Li2 2.136(3), Li2–I1 2.776(3), I1–Li1 2.911(3), Li1–I2 2.748(3), Li1–I1 2.911(3), C27–Li1 2.633(3), Li3–I2′ 2.850(3), Li3–I2 2.970(3), C21–Li1 2.391(3), C21–Li3 2.216(3), C21–N2 1.3771(19), N2–N3 1.3414(17), N3–N4 1.3249(18), N4–C22 1.357(2), C22–C21 1.398(2), C2–N2 1.4348(19), N1–Li1 2.052(3), N1–Li2 2.188(3), C1–N1–C8 103.18(12), N2–C21–C22 100.22(12), N5–C27–C28 100.25(13), C1–C2–N2–N3 137.4(1), C8–C9–N5–N6 151.3(1). | |
The Li2–I1 bond length [2.776(3) Å] is comparable with that observed in lithium iodide adducts (2.70–2.80 Å). Also the larger Li3–I2 distance [2.970(3) Å] is in the range of previously reported lithium iodide clusters (2.98 Å).104–108 The N1 nitrogen atom of the carbazolido- (Fig. 4B and C), as well as the MIC-ligands, thus coordinate one lithium atom (Li1) and formally another molecule of lithium iodide (Li2–I1 and Li3–I2, respectively). Overall, this arrangement locks the central Li1 atom in place. Eventually, the lability of the lithium iodide was corroborated by elemental analysis (ESI†). Repeated re-crystallization lowered the equivalents of lithium iodide from two, as present in the solid-state structure shown in Fig. 4, to 0.3 equivalents.
Complex MgBr5 was prepared by transmetalation of Li5 with MgBr2, but may be as well prepared directly from 3 and the Grignard reagent MeMgBr.109 We were not able to obtain single crystals of sufficient quality for elucidation of the structure in the solid-state.110 However, a diffusion NMR experiment (DOSY) revealed that solutions of 4 and MgBr5 are mononuclear in deuterated benzene, whereas Li5 remains a dimer (page S17). Like blue-green luminescent Li5 (and in contrast to non-emissive 3 and weakly emissive 4) complex MgBr5 showed intense, lime-green luminescence in solution, whereas all investigated compounds were essentially non-luminescent in the solid state.
Luminescent properties
The bright luminescence motivated more detailed photophysical studies of all compounds. In benzene solutions, very strong luminescence is seen for Li5, strong luminescence for MgBr5, and undetectable to low luminescence for 3 and 4Fig. 5. Pertinent spectroscopic features are summarized in Table 1, normalized steady-state absorption and luminescence spectra are shown in Fig. 6.
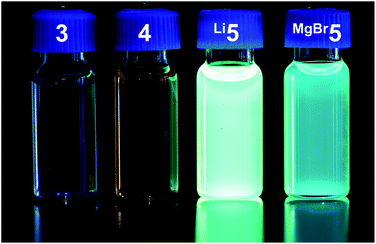 |
| Fig. 5
Li5 and MgBr5 are luminescent, whereas 3 and 4 are undetectable to low luminescent. The picture was obtained upon irradiation of 1 mM benzene solutions with a common laboratory UV lamp (360 nm). | |
Table 1 Key spectroscopic data of 3, 4, Li5, and MgBr5a
Compound |
λ
abs,max/nm |
λ
em/nm |
Φ
em/% |
E
Stokes/eV (cm−1) |
Spectroscopic data were obtained at room-temperature for 1 × 10−5 M benzene solutions of 3, 4, Li5, and MgBr5. The emission wavelengths and quantum yields were obtained after excitation at 390 nm.
The plateau is assigned to vibronic transitions as discussed below.
|
3
|
360, (492)b |
— |
— |
— |
4
|
352, 445, 487 |
565 |
2 |
0.35 (2835) |
Li5
|
325, 402, 465 |
506 |
16 |
0.23 (2016) |
MgBr5
|
339, 380, 431 |
482 |
14 |
0.30 (2455) |
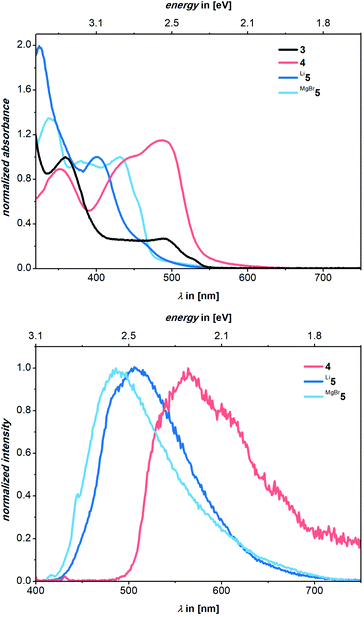 |
| Fig. 6 Normalized absorption (top) and luminescence (bottom) spectra of 3 (black), 4 (red), Li5 (dark blue), and MgBr5 (light blue) in benzene. The luminescence was recorded upon photoexcitation at 390 nm. Absorbance spectra are normalized at their 360, 445, 402, and 431 nm maxima, respectively, and luminescence spectra to the highest feature. | |
Although the biscationic proligand 3 is poorly soluble in organic solvents, maxima evolved at 297 and 360 nm. It features a plateau-like shoulder peaking at 492 nm and reaching up to approximately 570 nm. Upon photoexcitation in benzene, 3 is found to be non-luminescent. In contrast, in the absorption spectra of 4, we find maxima at 352, 445, and 487 nm. Photoexcitation of 4 at, for example, 390 nm leads to a broad and undefined luminescence with a maximum at 565 nm and a 2% Φem (Fig. 6). Turning to the absorption spectrum of Li5, two maxima are discernable at 325 and 402 nm. A tail is superimposed onto the latter all the way to 600 nm including a minor shoulder centered at around 465 nm. Excitation spectra for Li5 and MgBr5 reveal that the lowest absorption wavelength which leads to luminescence is 465 nm for Li5 and 434 nm for MgBr5. In both cases, this is in line with their absorption maxima (Fig. S21†). We determined Stokes shifts of 0.35, 0.30, and 0.23 eV for 4, MgBr5 and Li5, respectively.
Excitation of Li5 at 390 nm gives a luminescence maximum at 506 nm and a luminescence quantum yield of 16%. The luminescence spectrum of Li5 also shows a tail up to 725 nm. Turning finally to MgBr5, we note absorption maxima at 339, 380, and 431 nm. Once again, the latter features a tail up to approximately 560 nm.
Photoexcitation of MgBr5 at 390 nm leads to a luminescence that reaches a maximum at 487 nm with a quantum yield of 14%. Again, a tail up to 730 nm is noted. Upon exposure of solutions of Li5 and MgBr5 to air, the spectroscopic signatures of mono-deprotonated 4 were regenerated (Fig. S21 and S22†). Time-Correlated Single-Photon Counting (TCSPC) experiments on Li5 and MgBr5 revealed a luminescence decay with lifetimes of (11.8 ± 1.6 × 10−2) and (10.7 ± 4.8 × 10−2) ns, respectively (Fig. S25 and S26†). By virtue of lifetime components which are in the typical range for singlet excited states, we rule out the involvement of triplet excited state species as is the case for TADF or phosphorescence. In other words, the luminescent deactivation is fluorescence.
Computational analysis
Puzzled by the optical properties, we investigated the electronic and structural properties of all compounds in their excited states. Exploratory TD-DFT calculations revealed that the transitions to the S1 state of 3 and 4 are ILCT (approximated HOMO→LUMO, Fig. 7A and C) processes.
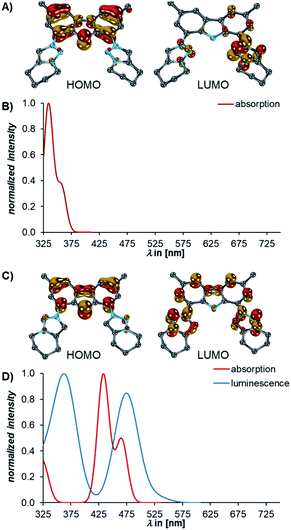 |
| Fig. 7 The dominant absorptions and concomitant excitations to the S1 states of 3 and 4 belong to the HOMO → LUMO transitions (B and D, red). For both 3 and 4, the HOMOs (left) are located at the carbazole framework, whereas the LUMOs (right) are mainly localized on one of the triazolium groups (3, A) or both MIC units (4, C). While 3 is non-luminescent, 4 shows a weak luminescence from the S1 state (D, blue). Orbitals were obtained at the TD-DFT(SMD=C6H6)/def2-TZVPP//B3LYP-D3(BJ)/def2-SVP level of theory, whereas the luminescence spectra were obtained at the STEOM-CCSD(SMD=C6H6)/def2-TZVPP//B3LYP-D3(BJ)/def2-SVP level of theory. Hydrogen atoms are omitted for clarity. | |
The HOMO is located at the carbazolido ligand, whereas the LUMO is associated with the MICs (cf. Fig. S32†). For an accurate description of the charge-transfer states, which is challenging for TD-DFT111–113 (Fig. S33–S35†), absorption spectra were computed with the more suitable ab initio method “Similarly Transformed Equation of Motion Coupled Cluster Singles and Doubles” (STEOM-CCSD)114–116 using the “Domain-based Local Pair Natural Orbital (DLPNO)” approximation.117,118 Indeed, this method reproduced the absorption spectra including the transitions to the S1 states best (3, fosc = 0.13, calcdλabs = 357 nm, expλabs = 360 nm, Fig. 7B; 4, fosc = 0.19, calcdλabs = 466 nm, expλabs = 487 nm, Fig. 7D).
The luminescence from the S1 state of 4 is predicted to be weak (fosc = 0.01, calcdλem = 528 nm, expλem = 565 nm, Fig. 7D) which is in agreement with the observation that 4 is weakly luminescent with a 2% quantum yield. For 3, neither TD-DFT nor STEOM-CCSD reproduced the plateau between 425 and 525 nm. However, the calculation of the vibronically resolved absorption spectrum using excited state dynamics (Fig. S36†) shows that this shoulder arises from molecular vibrations.
Subsequently, we investigated the electronic structures of the complexes 5. The calculations revealed that the dimeric structure of Li5 could be well modelled by calculating the electronic structure of the truncated monomer, thereby omitting the diethyl ether molecules as well (Fig. S32†).
A bright transition to the S1 state Li5truncated (fosc = 0.17, calcdλabs = 338 nm, expλabs = 402 nm) was also predicted for Li5 (Fig. 8B). This transition originates also from the ILCT from the carbazolido- to the MIC ligand (Fig. 8). The same is true for MgBr5 (fosc = 0.17, calcdλabs = 407 nm, expλabs = 431 nm (Fig. 8D). Luminescence from the S1 state of the complexes 5 is bright (Li5, fosc = 0.10, calcdλem = 408 nm, expλem = 506 nm, MgBr5fosc = 0.20, calcdλem = 435 nm, expλem = 482 nm, Fig. 8B and D, respectively) and in agreement with 5 being luminescent with quantum yields of 16% for Li5 and 14% for MgBr5. The STEOM-CCSD calculations also reproduce the experimental Stokes shifts (4calcd = 0.31, 4exp = 0.35; Li5truncated calcd = 0.40, Li5exp = 0.25; and MgBr5calcd = 0.20, MgBr5exp = 0.30 eV).
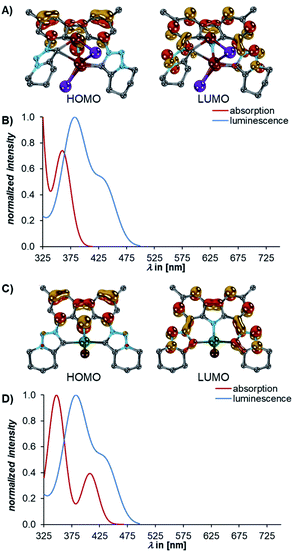 |
| Fig. 8 The dominant absorptions and concomitant excitations to the S1 states of Li5 and MgBr5 belong to the HOMO → LUMO transitions (B and D, red). For both Li5 and MgBr5, the HOMOs (left) are located at the carbazole, whereas the LUMOs (right) are mainly localized on both MIC units (A and C). For both Li5 and MgBr5, the luminescence from the S1 states is bright (Li5, B and MgBr5, D, blue). Orbitals were obtained at the TD-DFT(SMD=C6H6)/def2-TZVPP//B3LYP-D3(BJ)/def2-SVP level of theory, whereas the luminescence spectra were obtained at the STEOM-CCSD(SMD=C6H6)/def2-TZVPP//B3LYP-D3(BJ)/def2-SVP level of theory. Hydrogen atoms are omitted for clarity. | |
Considering the vastly differing luminescent properties of 3, 4, and complexes 5, we evaluated the geometries of the first S1 excited states. The proligand 3 is distorted in the relaxed S1 state, in which a triazolium group bends out of plane (Fig. 9, left). This means that the excitation is followed by a change of the mean value of the C–C–N–N dihedral angles before and after excitation (Δ∠C–C–N–N) between the carbazole and the triazolium unit of 90° (S0: ∠C–C–N–N = 10° and S1: ∠C–C–N–N = 100°). A comparable degree of distortion was also derived from the root-mean-square deviation for the change of the positions of all atoms (RMSDS0→S1; Fig. S38†).
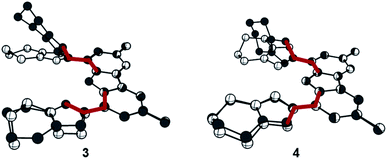 |
| Fig. 9 The distortions in the excited states of the proligand 3 (left) and 4 (right) visualized by superposition of the S0 (white) and S1 (grey) states. tBu groups are truncated to methyl groups, and hydrogen atoms are omitted for clarity. Dihedral angles (∠C–C–N–N) are highlighted in red. | |
Upon the photoexcitation of 4, a MIC group bends out of plane as well (Fig. 9, right) with Δ∠C–C–N–N = 42° (S0: ∠C–C–N–N = 177° and S1: ∠C–C–N–N = 135°). Li5 essentially retains the ground-state geometry in the relaxed S1 state, due to the fact that the coordinated metal ions lock the MIC ligands in place (Fig. 10, left). Throughout the excitation, the Δ∠C–C–N–N changes only by 7° (S0: ∠C–C–N–N = 139° and S1: ∠C–C–N–N = 146°).
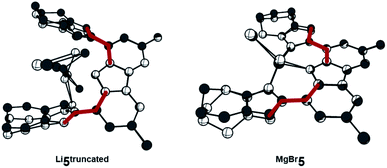 |
| Fig. 10 The metal prevents the distortions due to excitation in case of Li5truncated (left) and MgBr5 (right), as visualized by the superposition of the S0 (white) and S1 (grey) states. tBu groups are truncated to methyl groups, and hydrogen atoms are omitted for clarity. Dihedral angles (∠C–C–N–N) are highlighted in red. | |
The anchoring effect of the metal is also evident for MgBr5, which retains a pseudo-square-planar coordination around the magnesium ion (Fig. 10, right). Here, the Δ∠C–C–N–N is 11° (S0: ∠C–C–N–N = 177° and S1 ∠C–C–N–N = 166°). Intriguingly, the computationally determined degree of distortion in the excited S1 state correlates well with the experimental Stokes shifts and quantum yields (Fig. 11). For instance, 4 shows a large Stokes shift of ΔE = 0.35 eV and Δ∠C–C–N–N = 42°, while Li5 exhibits a Stokes shift of ΔE = 0.25 eV and Δ∠C–C–N–N = 7°.
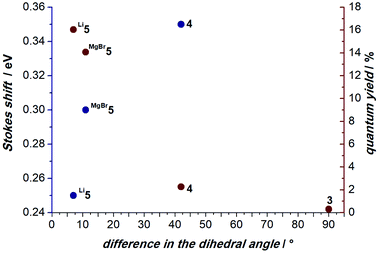 |
| Fig. 11 The excited state distortions, as expressed by the change of the mean value of the Δ∠C–C–N–N dihedral angles between the carbazole and the MICs, correlate with the Stokes shifts (blue circles) and quantum yields (red circles) of compounds 3, 4, Li5 and MgBr5. No Stokes shift is available for non-emissive 3. | |
Berlman suggested that chromophores, in which the ground and first excited states are planar, show high quantum yields,119,120 because molecular distortions and rotations in the relaxed excited state often lead to non-radiative relaxation.121–123 This rigidification or Restriction of Intramolecular Motions (RIM) principle is of course crucial for organic fluorophores including ubiquitous BODIPY.124,125 It has also been exploited in sensing and coordination chemistry as the Chelation-Enhanced Fluorescence (CHEF) effect, in which the ground and excited geometries of a molecule are locked into a planar conformation by coordinating metals.126–132
Accordingly, complexes with comparably small structural distortions in the excited state are more efficient emitters (Li5 Δ∠C–C–N–N = 7°, Φem = 16%; MgBr5 Δ∠C–C–N–N = 11°, Φem = 14%; 4 Δ∠C–C–N–N = 42°, Φem = 2%; 3 Δ∠C–C–N–N = 90°, non-emissive). Therefore, we conclude that the structural relaxation of the excited S1 state quenches the luminescence. In contrast, the alkali- and earth-alkali metals lock the conformation and, hence, allow for bright luminescence with comparably small Stokes shifts.
Conclusions
We report on the synthesis of an N-fused CNC pincer proligand composed of carbazole and two triazolium units. The synthetic approach, scalable to multigram quantities, avoids the use of hazardous tert-butylhypochloride, which had found use for related ligand systems. The proligand undergoes single deprotonation to afford a rare “naked” amide, which is air-stable due to intramolecular hydrogen-type bonding interactions. Triple deprotonation by a lithium base affords a chelated, binuclear lithium complex, which undergoes transmetalation with magnesium. Photophysical investigations show that the s-block complexes excel with luminescence quantum yields of up to 16% at ambient temperature and in solution, whereas the pro- and mono-deprotonated ligands are essentially non-luminescent. Detailed quantum-chemical calculations helped to rationalize the luminescent properties with an Intra-Ligand- Charge-Transfer (ILCT) from the carbazolide to the mesoionic carbenes. (Earth-)alkali metals prevent the distortion of the ligand following excitation and, in turn, enable bright luminescence in the blue to green region of the spectrum.
Author contributions
PP and SH prepared the compound 4, PP prepared as well complexes Li5, MgBr5 and ran the computations, CMS supported by TU performed the photochemical studies, FAW and ND synthesized the compounds 1, 2 and 3, RHI performed the XRD analysis of 3, SH performed the XRD analysis of 4, BM performed the XRD analysis of Li5, AG contributed to the synthesis of Li5 and MgBr5, MZ performed the DOSY experiments, DMG, SH and DM coordinated the project, DM conceived the idea. The manuscript was proof-read and approved by all authors.
Conflicts of interest
There are no conflicts to declare.
Acknowledgements
We thank K. Meyer and the Boehringer-Ingelheim Foundation (Exploration Grant) for their support. We also acknowledge the RRZ Erlangen for the generous allocation of computing time. DMG acknowledges the Emerging Fields Initiative “Singlet Fission” supported by FAU Erlangen-Nürnberg and funding from the Bavarian State Government as part of the Solar Technologies go Hybrid initiative. SH thanks the Daimler and Benz Foundation, the Fonds der Chemischen Industrie, Paderborn University and the University of Innsbruck for financial support. Instrumentation and technical assistance for the XRD analysis of Li5 were provided by the Service Center X-ray Diffraction, with financial support from Saarland University and the German Science Foundation DFG (project number INST 256/349-1).
Notes and references
- V. Promarak, M. Ichikawa, T. Sudyoadsuk, S. Saengsuwan, S. Jungsuttiwong and T. Keawin, Thin Solid Films, 2008, 516, 2881 CrossRef CAS.
- Z. S. Wang, N. Koumura, Y. Cui, M. Takahashi, H. Sekiguchi, A. Mori, T. Kubo, A. Furube and K. Hara, Chem. Mater., 2008, 20, 3993 CrossRef CAS.
- J. Tang, J. L. Hua, W. J. Wu, J. Li, Z. G. Jin, Y. T. Long and H. Tian, Energy Environ. Sci., 2010, 3, 1736 RSC.
- J. Luo and J. Zhang, ACS Catal., 2016, 6, 873 CrossRef CAS.
- A. Al Mousawi, F. Dumur, P. Garra, J. Toufaily, T. Hamieh, B. Graff, D. Gigmes, J. P. Fouassier and J. Lalevée, Macromolecules, 2017, 50, 2747 CrossRef CAS.
- Z. Huang, Y. Gu, X. Liu, L. Zhang, Z. Cheng and X. Zhu, Macromol. Rapid Commun., 2017, 38, 1600461 CrossRef PubMed.
- F. Dumur, Eur. Polym. J., 2020, 125, 109503 CrossRef.
- K. Brunner, A. van Dijken, H. Börner, J. J. A. M. Bastiaansen, N. M. M. Kiggen and B. M. W. Langeveld, J. Am. Chem. Soc., 2004, 126, 6035 CrossRef CAS PubMed.
- B. Wex and B. R. Kaafarani, J. Mater. Chem., 2017, 5, 8622 CAS.
- T. Fleetham, G. Li, L. Wen and J. Li, Adv. Mater., 2014, 26, 7116 CrossRef CAS PubMed.
- T. Fleetham, G. Li and J. Li, Adv. Mater., 2017, 29, 1601861 CrossRef PubMed.
- H. Uoyama, K. Goushi, K. Shizu, H. Nomura and C. Adachi, Nature, 2012, 492, 234 CrossRef CAS PubMed.
- P. Kotchapradist, N. Prachumrak, R. Tarsang, S. Jungsuttiwong, T. Keawin, T. Sudyoadsuk and V. Promarak, J. Mater. Chem., 2013, 1, 4916 RSC.
- Z. Gao, Z. M. Wang, T. Shan, Y. L. Liu, F. Z. Shen, Y. Y. Pan, H. H. Zhang, X. He, P. Lu, B. Yang and Y. G. Ma, Org. Electron., 2014, 15, 2667 CrossRef CAS.
- K. Albrecht, K. Matsuoka, K. Fujita and K. Yamamoto, Angew. Chem., Int. Ed., 2015, 54, 5677 CrossRef CAS PubMed.
- Q. Zhang, J. Li, K. Shizu, S. Huang, S. Hirata, H. Miyazaki and C. Adachi, J. Am. Chem. Soc., 2012, 134, 14706 CrossRef CAS PubMed.
- T. Furukawa, H. Nakanotani, M. Inoue and C. Adachi, Sci. Rep., 2015, 5, 8429 CrossRef CAS PubMed.
- H. Kaji, H. Suzuki, T. Fukushima, K. Shizu, K. Suzuki, S. Kubo, T. Komino, H. Oiwa, F. Suzuki, A. Wakamiya, Y. Murata and C. Adachi, Nat. Commun., 2015, 6, 8476 CrossRef CAS PubMed.
- D. Di, A. S. Romanov, L. Yang, J. M. Richter, J. P. Rivett, S. Jones, T. H. Thomas, M. Abdi Jalebi, R. H. Friend, M. Linnolahti, M. Bochmann and D. Credgington, Science, 2017, 356, 159 CrossRef CAS PubMed.
- Y. H. Lee, S. Park, J. Oh, S. J. Woo, A. Kumar, J. J. Kim, J. Jung, S. Yoo and M. H. Lee, Adv. Opt. Mater., 2018, 6, 1800385 CrossRef.
- Y. C. Liu, C. S. Li, Z. J. Ren, S. K. Yan and M. R. Bryce, Nat. Rev. Mater., 2018, 3, 18020 CrossRef CAS.
- R. Hamze, J. L. Peltier, D. Sylvinson, M. Jung, J. Cardenas, R. Haiges, M. Soleilhavoup, R. Jazzar, P. I. Djurovich, G. Bertrand and M. E. Thompson, Science, 2019, 363, 601 CrossRef CAS PubMed.
- R. Hamze, S. Shi, S. C. Kapper, D. S. Muthiah Ravinson, L. Estergreen, M. C. Jung, A. C. Tadle, R. Haiges, P. I. Djurovich, J. L. Peltier, R. Jazzar, G. Bertrand, S. E. Bradforth and M. E. Thompson, J. Am. Chem. Soc., 2019, 141, 8616 CrossRef CAS PubMed.
- S. Shi, M. C. Jung, C. Coburn, A. Tadle, M. R. D. Sylvinson, P. I. Djurovich, S. R. Forrest and M. E. Thompson, J. Am. Chem. Soc., 2019, 141, 3576 CrossRef CAS PubMed.
- P. J. Conaghan, C. S. B. Matthews, F. Chotard, S. T. E. Jones, N. C. Greenham, M. Bochmann, D. Credgington and A. S. Romanov, Nat. Commun., 2020, 11, 1758 CrossRef CAS PubMed.
- H. T. Feng, J. Zeng, P. A. Yin, X. D. Wang, Q. Peng, Z. Zhao, J. W. Y. Lam and B. Z. Tang, Nat. Commun., 2020, 11, 2617 CrossRef CAS PubMed.
- A. S. Romanov, F. Chotard, J. Rashid and M. Bochmann, Dalton Trans., 2019, 48, 15445 RSC.
- J. Feng, E. J. Taffet, A. P. M. Reponen, A. S. Romanov, Y. Olivier, V. Lemaur, L. Yang, M. Linnolahti, M. Bochmann, D. Beljonne and D. Credgington, Chem. Mater., 2020, 32, 4743 CrossRef CAS.
- C. R. Hall, A. S. Romanov, M. Bochmann and S. R. Meech, J. Phys. Chem. Lett., 2018, 9, 5873 CrossRef CAS PubMed.
- A. S. Romanov, L. Yang, S. T. E. Jones, D. Di, O. J. Morley, B. H. Drummond, A. P. M. Reponen, M. Linnolahti, D. Credgington and M. Bochmann, Chem. Mater., 2019, 31, 3613 CrossRef CAS.
- A. S. Romanov, S. T. E. Jones, L. Yang, P. Conaghan, D. W. Di, M. Linnolahti, D. Credgington and M. Bochmann, Adv. Opt. Mater., 2018, 6, 1801347 CrossRef.
- M. Gernert, L. Balles-Wolf, F. Kerner, U. Müller, A. Schmiedel, M. Holzapfel, C. M. Marian, J. Pflaum, C. Lambert and A. Steffen, J. Am. Chem. Soc., 2020, 142, 8897 CrossRef CAS PubMed.
- For thematic issues on NHCs, see:
(a) F. E. Hahn, Chem. Rev., 2018, 118, 9455 CrossRef CAS PubMed;
(b) A. J. Arduengo and G. Bertrand, Chem. Rev., 2009, 109, 3209 CrossRef CAS PubMed; For thematic books on NHCs, see:
(c)
S. P. Nolan, N-Heterocyclic Carbenes: Effective Tools for Organometallic Synthesis, Wiley-VCH, Weinheim, 2014 Search PubMed;
(d)
S. Diez-Gonzalez
N-Heterocyclic Carbenes: From Laboratory Curiosities to Efficient Synthetic Tools, Royal Society of Chemistry, Cambridge, 2016 RSC; For concise reviews on carbene ligands, see:
(e) M. N. Hopkinson, C. Richter, M. Schedler and F. Glorius, Nature, 2014, 510, 485 CrossRef CAS PubMed;
(f) D. Munz, Organometallics, 2018, 37, 275 CrossRef CAS; For reviews on NHC complexes of the groups 1 and 2, see:
(g) S. Bellemin-Laponnaz and S. Dagorne, Chem. Rev., 2014, 114, 8747 CrossRef CAS PubMed;
(h) V. Nesterov, D. Reiter, P. Bag, P. Frisch, R. Holzner, A. Porzelt and S. Inoue, Chem. Rev., 2018, 118, 9678 CrossRef CAS PubMed.
- J. Foller and C. M. Marian, J. Phys. Chem. Lett., 2017, 8, 5643 CrossRef PubMed.
- E. J. Taffet, Y. Olivier, F. Lam, D. Beljonne and G. D. Scholes, J. Phys. Chem. Lett., 2018, 9, 1620 CrossRef CAS PubMed.
- S. Thompson, J. Eng and T. J. Penfold, J. Chem. Phys., 2018, 149, 014304 CrossRef CAS PubMed.
- R. Gomez-Bombarelli, J. Aguilera-Iparraguirre, T. D. Hirzel, D. Duvenaud, D. Maclaurin, M. A. Blood-Forsythe, H. S. Chae, M. Einzinger, D. G. Ha, T. Wu, G. Markopoulos, S. Jeon, H. Kang, H. Miyazaki, M. Numata, S. Kim, W. Huang, S. I. Hong, M. Baldo, R. P. Adams and A. Aspuru-Guzik, Nat. Mater., 2016, 15, 1120 CrossRef CAS PubMed.
- E. Peris, Chem. Rev., 2018, 118, 9988 CrossRef CAS PubMed.
- M. Poyatos, J. A. Mata and E. Peris, Chem. Rev., 2009, 109, 3677 CrossRef CAS PubMed.
- E. Peris and R. H. Crabtree, Coord. Chem. Rev., 2004, 248, 2239 CrossRef CAS.
- A. Röther and R. Kretschmer, J. Organomet. Chem., 2020, 918, 121289 CrossRef.
- D. Munz, Chem. Sci., 2018, 9, 1155 RSC.
- A. Grünwald and D. Munz, J. Organomet. Chem., 2018, 864, 26 CrossRef.
- A. Grünwald, N. Orth, A. Scheurer, F. W. Heinemann, A. Pothig and D. Munz, Angew. Chem., Int. Ed., 2018, 57, 16228 CrossRef PubMed.
- M. S. Bennington, H. L. Feltham, Z. J. Buxton, N. G. White and S. Brooker, Dalton Trans., 2017, 46, 4696 RSC.
- H. C. Gee, C. H. Lee, Y. H. Jeong and W. D. Jang, Chem. Commun., 2011, 47, 11963 RSC.
- H. Aihara, L. Jaquinod, D. J. Nurco and K. M. Smith, Angew. Chem., Int. Ed., 2001, 40, 3439 CrossRef CAS PubMed.
- M. Inoue and M. Nakada, Heterocycles, 2007, 72, 133 CrossRef CAS.
- L. Arnold, H. Norouzi-Arasi, M. Wagner, V. Enkelmann and K. Mullen, Chem. Commun., 2011, 47, 970 RSC.
- M. Nath, J. C. Huffman and J. M. Zaleski, Chem. Commun., 2003, 858 RSC.
- I. Pryjomska-Ray, D. Zornik, M. Patzel, K. B. Krause, L. Grubert, B. Braun-Cula, S. Hecht and C. Limberg, Chem.–Eur. J., 2018, 24, 5341 CrossRef CAS PubMed.
- B. Schulze, C. Friebe, M. Jäger, H. Görls, E. Birckner, A. Winter and U. S. Schubert, Organometallics, 2017, 37, 145 CrossRef.
- X. Zhang, L. Y. Zhang, J. Y. Wang, F. R. Dai and Z. N. Chen, J. Mater. Chem., 2020, 8, 715 RSC.
- M. Inoue, T. Suzuki and M. Nakada, J. Am. Chem. Soc., 2003, 125, 1140 CrossRef CAS PubMed.
- J. A. Gaunt, V. C. Gibson, A. Haynes, S. K. Spitzmesser, A. J. P. White and D. J. Williams, Organometallics, 2004, 23, 1015 CrossRef CAS.
- G. J. P. Britovsek, V. C. Gibson, O. D. Hoarau, S. K. Spitzmesser, A. J. P. White and D. J. Williams, Inorg. Chem., 2003, 42, 3454 CrossRef CAS PubMed.
- V. C. Gibson, S. K. Spitzmesser, A. J. P. White and D. J. Williams, Dalton Trans., 2003, 2718 RSC.
- G. Kleinhans, M. M. Hansmann, G. Guisado-Barrios, D. C. Liles, G. Bertrand and D. I. Bezuidenhout, J. Am. Chem. Soc., 2016, 138, 15873 CrossRef CAS PubMed.
- D. I. Bezuidenhout, G. Kleinhans, G. Guisado-Barrios, D. C. Liles, G. Ung and G. Bertrand, Chem. Commun., 2014, 50, 2431 RSC.
- G. Kleinhans, G. Guisado-Barrios, E. Peris and D. I. Bezuidenhout, Polyhedron, 2018, 143, 43 CrossRef CAS.
- P. Jerabek, L. Vondung and P. Schwerdtfeger, Chem.–Eur. J., 2018, 24, 6047 CrossRef CAS PubMed.
- G. Kleinhans, A. K. W. Chan, M. Y. Leung, D. C. Liles, M. A. Fernandes, V. W. W. Yam, I. Fernández and D. I. Bezuidenhout, Chem.–Eur. J., 2020, 26, 6993 CrossRef CAS PubMed.
- M. Moser, B. Wucher, D. Kunz and F. Rominger, Organometallics, 2007, 26, 1024 CrossRef CAS.
- E. Jürgens, K. N. Buys, A. T. Schmidt, S. K. Furfari, M. L. Cole, M. Moser, F. Rominger and D. Kunz, New J. Chem., 2016, 40, 9160 RSC.
- A. Seyboldt, B. Wucher, S. Hohnstein, K. Eichele, F. Rominger, K. W. Tornroos and D. Kunz, Organometallics, 2015, 34, 2717 CrossRef CAS.
- T. Maulbetsch, E. Jürgens and D. Kunz, Chem.–Eur. J., 2020, 26, 10634 CrossRef CAS PubMed.
- E. Jürgens, O. Back, J. J. Mayer, K. Heinze and D. Kunz, Z. Naturforsch., B: J. Chem. Sci., 2016, 71, 1011 CrossRef.
- Y. Y. Tian, E. Jürgens, K. Mill, R. Jordan, T. Maulbetsch and D. Kunz, ChemCatChem, 2019, 11, 4028 CrossRef CAS.
- S. C. Sau, P. K. Hota, S. K. Mandal, M. Soleilhavoup and G. Bertrand, Chem. Soc. Rev., 2020, 49, 1233 RSC.
- Á. Vivancos, C. Segarra and M. Albrecht, Chem. Rev., 2018, 118, 9493 CrossRef PubMed.
- O. Schuster, L. Yang, H. G. Raubenheimer and M. Albrecht, Chem. Rev., 2009, 109, 3445 CrossRef CAS PubMed.
- R. S. Ghadwal, Dalton Trans., 2016, 45, 16081 RSC.
-
H. V. Huynh, in The Organometallic Chemistry of N-heterocyclic Carbenes, 2017, p. 293 Search PubMed.
- D. Schweinfurth, L. Hettmanczyk, L. Suntrup and B. Sarkar, Z. Anorg. Allg. Chem., 2017, 643, 554 CrossRef CAS.
- G. Guisado-Barrios, M. Soleilhavoup and G. Bertrand, Acc. Chem. Res., 2018, 51, 3236 CrossRef CAS PubMed.
- H. V. Huynh, Chem. Rev., 2018, 118, 9457 CrossRef CAS PubMed.
- D. J. Nelson and S. P. Nolan, Chem. Soc. Rev., 2013, 42, 6723 RSC.
- V. Leigh, W. Ghattas, R. Lalrempuia, H. Müller-Bunz, M. T. Pryce and M. Albrecht, Inorg. Chem., 2013, 52, 5395 CrossRef CAS PubMed.
- A. Baschieri, F. Monti, E. Matteucci, A. Mazzanti, A. Barbieri, N. Armaroli and L. Sambri, Inorg. Chem., 2016, 55, 7912 CrossRef CAS.
- J. Soellner, M. Tenne, G. Wagenblast and T. Strassner, Chem.–Eur. J., 2016, 22, 9914 CrossRef CAS PubMed.
- B. Sarkar and L. Suntrup, Angew. Chem., Int. Ed., 2017, 56, 8938 CrossRef CAS PubMed.
- E. Matteucci, F. Monti, R. Mazzoni, A. Baschieri, C. Bizzarri and L. Sambri, Inorg. Chem., 2018, 57, 11673 CrossRef CAS PubMed.
- L. Suntrup, F. Stein, G. Hermann, M. Kleoff, M. Kuss-Petermann, J. Klein, O. S. Wenger, J. C. Tremblay and B. Sarkar, Inorg. Chem., 2018, 57, 13973 CrossRef CAS PubMed.
- J. Soellner and T. Strassner, Chem.–Eur. J., 2018, 24, 5584 CrossRef CAS PubMed.
- J. Soellner and T. Strassner, ChemPhotoChem, 2019, 3, 1000 CrossRef CAS.
- D. G. Brown, N. Sanguantrakun, B. Schulze, U. S. Schubert and C. P. Berlinguette, J. Am. Chem. Soc., 2012, 134, 12354 CrossRef CAS PubMed.
- T. Bens, P. Boden, P. Di Martino-Fumo, J. Beerhues, U. Albold, S. Sobottka, N. I. Neuman, M. Gerhards and B. Sarkar, Inorg. Chem., 2020, 59, 15504 CrossRef CAS PubMed.
- J. Messelberger, A. Grünwald, P. Pinter, M. M. Hansmann and D. Munz, Chem. Sci., 2018, 9, 6107 RSC.
- P. Pinter and D. Munz, J. Phys. Chem. A, 2020, 124, 10100 CrossRef CAS PubMed.
- T. Maulbetsch and D. Kunz, Angew. Chem., Int. Ed., 2021, 60, 2007 CrossRef CAS PubMed.
- O. Back, C. Förster, T. Basché and K. Heinze, Chem.–Eur. J., 2019, 25, 6542 CrossRef CAS PubMed.
- Note that lithium quinolates found use as material in OLEDs:
(a) Z. Liu, O. V. Salata and N. Male, Synth. Met., 2002, 128, 211 CrossRef CAS;
(b) C. Schmitz, H.-W. Schmidt and M. Thelakkat, Chem. Mater., 2000, 12, 3012 CrossRef CAS.
- T. J. Feuerstein, B. Goswami, P. Rauthe, R. Köppe, S. Lebedkin, M. M. Kappes and P. W. Roesky, Chem. Sci., 2019, 10, 4742 RSC.
- R. D. Ribson, G. Choi, R. G. Hadt and T. Agapie, ACS Cent. Sci., 2020, 6, 2088 CrossRef CAS PubMed.
- For leading reviews, see:
(a) C. Förster and K. Heinze, Chem. Soc. Rev., 2020, 49, 1057 RSC;
(b) O. S. Wenger, J. Am. Chem. Soc., 2018, 140, 13522 CrossRef CAS PubMed; For leading recent examples, see:
(c) Y. Zhang, T. S. Lee, J. M. Favale, D. C. Leary, J. L. Petersen, G. D. Scholes, F. N. Castellano and C. Milsmann, Nat. Chem., 2020, 12, 345 CrossRef CAS PubMed;
(d) K. S. Kjær, N. Kaul, O. Prakash, P. Chábera, N. W. Rosemann, A. Honarfar, O. Gordivska, L. A. Fredin, K. E. Bergquist, L. Häggström, T. Ericsson, L. Lindh, A. Yartsev, S. Styring, P. Huang, J. Uhlig, J. Bendix, D. Strand, V. Sundström, P. Persson, R. Lomoth and K. Wärnmark, Science, 2019, 363, 249 CrossRef PubMed;
(e) P. Chábera, Y. Liu, O. Prakash, E. Thyrhaug, A. E. Nahhas, A. Honarfar, S. Essén, L. A. Fredin, T. C. B. Harlang, K. S. Kjær, K. Handrup, F. Ericson, H. Tatsuno, K. Morgan, J. Schnadt, L. Häggström, T. Ericsson, A. Sobkowiak, S. Lidin, P. Huang, S. Styring, J. Uhlig, J. Bendix, R. Lomoth, V. Sundström, P. Persson and K. Wärnmark, Nature, 2017, 543, 695 CrossRef PubMed.
- M. C. Tseng, H. T. Cheng, M. J. Shen and Y. H. Chu, Org. Lett., 2011, 13, 4434 CrossRef CAS PubMed.
- Intensity data of single crystals of the investigated compounds were collected using Mo Kα radiation. Semiempirical absorption corrections were performed on the basis of multiple scans using SADABS see:
(a) L. Krause, R. Herbst-Irmer, G. M. Sheldrick and D. Stalke, J. Appl. Crystallogr., 2015, 48, 3 CrossRef CAS PubMed. The structures were solved by direct methods using SHELXT see:
(b) G. Sheldrick, Acta Crystallogr., Sect. A: Found. Crystallogr., 2015, 71, 3 CrossRef PubMed. The structures were refined by full-matrix least-squares procedures on F2 using SHELXL see:
(c) G. Sheldrick, Acta Crystallogr., Sect. C: Struct. Chem., 2015, 71, 3 Search PubMed in the graphical user interface Shelxle see:
(d) C. B. Hübschle, G. M. Sheldrick and B. Dittrich, J. Appl. Crystallogr., 2011, 44, 1281 CrossRef PubMed . More details are given in the ESI.†.
- M. Baltrun, F. A. Watt, R. Schoch, C. Wölper, A. G. Neuba and S. Hohloch, Dalton Trans., 2019, 48, 14611 RSC.
- S. Hohloch, L. Suntrup and B. Sarkar, Inorg. Chem. Front., 2016, 3, 67 RSC.
- K. R. D. Johnson, B. L. Kamenz and P. G. Hayes, Organometallics, 2014, 33, 3005 CrossRef CAS.
- H. Esbak and U. Behrens, Z. Anorg. Allg. Chem., 2005, 631, 1581 CrossRef CAS.
- C. Lambert, F. Hampel and P. von Raguė Schleyer, Angew. Chem., Int. Ed., 1992, 31, 1209 CrossRef.
- M. T. Reetz, S. Hutte, R. Goddard and U. Minet, J. Chem. Soc., Chem. Commun., 1995, 275 RSC.
- A. Ünal and Ö. Ayın, J. Cluster Sci., 2021, 32, 507 CrossRef.
- C. L. Raston, C. R. Whitaker and A. H. White, J. Chem. Soc., Dalton Trans., 1988, 991 RSC.
- S. Komagawa, S. Usui, J. Haywood, P. J. Harford, A. E. Wheatley, Y. Matsumoto, K. Hirano, R. Takita and M. Uchiyama, Angew. Chem., Int. Ed., 2012, 51, 12081 CrossRef CAS PubMed.
- Z. Fei, R. Scopelliti and P. J. Dyson, Inorg. Chem., 2003, 42, 2125 CrossRef CAS PubMed.
- H. V. R. Dias and W. C. Jin, J. Chem. Crystallogr., 1997, 27, 353 CrossRef CAS.
- See the ESI† for synthetic details.
- XRD data for a Mg complex with a much related ancillary ligand further suggests that MgBr5 is a monomer.
- D. Jacquemin, B. Mennucci and C. Adamo, Phys. Chem. Chem. Phys., 2011, 13, 16987 RSC.
- C. Adamo and D. Jacquemin, Chem. Soc. Rev., 2013, 42, 845 RSC.
- A. D. Laurent and D. Jacquemin, Int. J. Quantum Chem., 2013, 113, 2019 CrossRef CAS.
- M. Nooijen and R. J. Bartlett, J. Chem. Phys., 1997, 106, 6441 CrossRef CAS.
- R. Berraud-Pache, F. Neese, G. Bistoni and R. Izsák, J. Chem. Theory Comput., 2020, 16, 564 CrossRef PubMed.
- R. Izsák, Wiley Interdiscip. Rev.: Comput. Mol. Sci., 2019, 10, e1445 Search PubMed.
- C. Riplinger and F. Neese, J. Chem. Phys., 2013, 138, 034106 CrossRef PubMed.
- C. Riplinger, B. Sandhoefer, A. Hansen and F. Neese, J. Chem. Phys., 2013, 139, 134101 CrossRef PubMed.
- I. B. Berlman, J. Phys. Chem. A, 1970, 74, 3085 CrossRef CAS.
- N. I. Nijegorodov and W. S. Downey, J. Phys. Chem. A, 1994, 98, 5639 CrossRef CAS.
- G. Baryshnikov, B. Minaev and H. Agren, Chem. Rev., 2017, 117, 6500 CrossRef CAS PubMed.
- D. Escudero and D. Jacquemin, Dalton Trans., 2015, 44, 8346 RSC.
- J. Mei, N. L. C. Leung, R. T. K. Kwok, J. W. Y. Lam and B. Z. Tang, Chem. Rev., 2015, 115, 11718 CrossRef CAS PubMed.
- A. Loudet and K. Burgess, Chem. Rev., 2007, 107, 4891 CrossRef CAS PubMed.
- G. Ulrich, R. Ziessel and A. Harriman, Angew. Chem., Int. Ed., 2008, 47, 1184 CrossRef CAS PubMed.
- M. E. Huston, K. W. Haider and A. W. Czarnik, J. Am. Chem. Soc., 1988, 110, 4460 CrossRef CAS.
- E. U. Akkaya, M. E. Huston and A. W. Czarnik, J. Am. Chem. Soc., 1990, 112, 3590 CrossRef CAS.
- D. H. Vance and A. W. Czarnik, J. Am. Chem. Soc., 1994, 116, 9397 CrossRef CAS.
- M. D. Shults, D. A. Pearce and B. Imperiali, J. Am. Chem. Soc., 2003, 125, 10591 CrossRef CAS PubMed.
- J. Y. Kwon, Y. J. Jang, Y. J. Lee, K. M. Kim, M. S. Seo, W. Nam and J. Yoon, J. Am. Chem. Soc., 2005, 127, 10107 CrossRef CAS PubMed.
- H. Y. Lee, D. R. Bae, J. C. Park, H. Song, W. S. Han and J. H. Jung, Angew. Chem., Int. Ed., 2009, 48, 1239 CrossRef CAS PubMed.
- N. Sinha, L. Stegemann, T. T. Y. Tan, N. L. Doltsinis, C. A. Strassert and F. E. Hahn, Angew. Chem., Int. Ed., 2017, 56, 2785 CrossRef CAS PubMed.
Footnotes |
† Electronic supplementary information (ESI) available. CCDC 1953709, 2047516 and 2060428. For ESI and crystallographic data in CIF or other electronic format see DOI: 10.1039/d1sc00846c |
‡ Dedicated to Dr Volker Huch on the occasion of his retirement. |
§ Authors contributed equally. |
|
This journal is © The Royal Society of Chemistry 2021 |
Click here to see how this site uses Cookies. View our privacy policy here.