DOI:
10.1039/D1RA07449K
(Paper)
RSC Adv., 2021,
11, 37482-37497
The performance of three novel Gemini surfactants as inhibitors for acid steel corrosion: experimental and theoretical studies†
Received
7th October 2021
, Accepted 12th November 2021
First published on 22nd November 2021
Abstract
Adipic acid was used to synthesize three nonionic Gemini surfactants containing different numbers of propylene oxide units in their structures. The produced surfactants have been characterized employing FTIR and 1H-NMR spectra. Some of the physical properties of them, namely, surface tension, maximum surface excess concentration, surface pressure, critical micelle concentration, and the minimal area of the surface taken by a single molecule, were computed. The inhibitory effect of the synthesized surfactants on the corrosion of C-steel (C45) in 1.0 M HCl solution was studied. Gravimetric and electrochemical methods were used for corrosion rate measurements. The outcomes acquired from the used methods showed that every one of the three surfactants works as a strong inhibitor for steel acidic corrosion. By raising surfactant concentration and exposure time, the inhibition proficiency improves. The inhibition efficiency exceeded 90% for the three compounds. The higher the propylene oxide units contained in the surfactant molecule the higher is its inhibition efficiency. Based on the findings, a mechanism for inhibitory action was proposed. Moreover, the density functional theory (DFT) and molecular electrostatic potential (MEP) were investigated for the three inhibitors. The calculated parameters were correlated with the inhibition efficiency.
1. Introduction
Corrosion represents a serious problem due to its significant negative impact on the economy, especially in industrial applications.1–5 The corrosion impact is not only on the metals and alloys that are corroded but extends to the loss of valuable materials contained in them. A clear example is the loss of petroleum from the corroded pipelines. However, it is well known that the corrosion phenomenon is spontaneous and cannot be completely stopped. Thus, a huge number of research studies are continuously conducted to reduce the effect of corrosion to its minimum.6–21 Many scenarios were suggested to achieve this goal among them using corrosion inhibitors. It was found that the use of some chemical compounds had a specific effect on the original solution properties. The main characteristic of these compounds is their capability to absorb on the metal surface because of their surface properties or possession of a lone pair of electrons besides other structural properties.
Carbon steel is considered a usual choice for many industrial applications. In the petroleum industry, for example, it is used in pipelines and tanks. Although stainless steel has higher corrosion resistance than carbon steel, the latter is often preferred due to its lower cost. However, scales, as well as corrosion products, are deposited at the steel surface during operation periods. The acid-pickling process is applied to remove the scales and restore the clean surface. A corrosion inhibitor must be employed in the procedure to prevent the acid from damaging the steel surface.22–28
Surfactant compounds tend to accumulate with high concentrations at the interfaces. Such property introduces this category of compounds as potential corrosion inhibitors. The special type of surface-active compounds is the Gemini surfactants with their unique structure that differs from that of other surfactants. The presence of many hydrophilic as well as hydrophobic groups improves their surface properties and thus promotes their role as corrosion inhibitors. Therefore, many studies were concentrated lately on the potential use of these compounds for corrosion inhibition.27,29–41 Quantum calculations based on the density functional theory are used to know the mechanism between the inhibitor and the metal surface. The efficiency of the inhibitor depends mainly on the electronic properties of the inhibitor.42–47
The goal of this research is to create novel Gemini surfactants that can be tested as corrosion inhibitors. The study also looks at how molecular structure affects their inhibitory power. Thus, three novel Gemini surfactants based on adipic acid with different numbers of propylene oxide units were synthesized. Their structures were characterized by NMR and IR spectra. Chemical and electrochemical techniques were used to investigate their impact on carbon steel acid corrosion. The inhibitory power and molecular structures of the studied surfactants were correlated using quantum chemical calculations.
2. Experimental techniques
2.1 Materials
Across Chemical Company (UK) was used to obtain adipic acid. Sigma-Aldrich (Germany) was used to obtain 1,4 butanediol, diethanolamine, and propylene oxide. Thionyl chloride and P-toluene sulfonic acid were from Fluka Chemika (Germany).
Benzene, xylene as well as potassium hydroxide were brought from (Al Gomhuria Trade Pharmaceuticals & Chemical Company, Egypt).
2.1.1 Synthesis of 5-{[6-(4-carboxybutoxy)-6-oxohexanoyl]oxy}pentanoic acid. Diester compound 5-{[6-(4-carboxybutoxy)-6-oxohexanoyl] oxy} pentanoic acid has been prepared via refluxing a mixture of 1,4 butanediol (0.05 mol) with adipic acid (0.1 mol) respectively, in 50 ml of dry benzene for six hours, in the presence of 0.1 wt% p-toluenesulfonic acid as a catalyst. Distilled water was added once the reaction mixture had cooled. The benzene layer which contains the diester was separated. To extract the crude product, the ester solution was vacuum distilled after being dried overnight. Fractional distillation under vacuum was used to further purify the product.48,49
2.1.2 Synthesis of 1,6-bis(5-chloro-5-oxopentyl)hexanedioate. To a cold thionyl-chloride (10 ml) at 0 °C was added 5-{[6-(4-carboxybutoxy)-6 oxohexanoyl]oxy}pentanoic acid (5.0 g, 24.72 mmol) portion-wise into the reaction flask with continuous stirring. The temperature was gradually raised to room temperature once the addition was completed, and the stirring was continued overnight. The resultant acid chloride was then taken in 50 ml CH2Cl2 then cooled to 0 °C after the volatile was expelled under vacuum.50
2.1.3 Synthesis of 1,6-bis({4-[bis(2-hydroxyethyl)carbamoyl]butyl})hexanedioate. In 50 ml xylene, the diester dichloro compound; 1,6-bis(5-chloro-5-oxopentyl)hexanedioate interacted with diethanolamine at a reaction molar ratio of 0.5
:
1.0, introducing dropwise triethylamine (TEA) as a catalyst while refluxing for five hours at 120 °C. Petroleum ether was being used to extract the product.51
2.1.4 Synthesis of nonionic Gemini surfactants. To remove oxygen from the system, 0.5 wt% KOH solutions containing 0.01 mol of the prepared (diester diamino compound) generated in the third step were heated to 70 °C and stirred whilst a constant flow of nitrogen is passed into the system. The nitrogen flow was turned off, and propylene oxide was supplied in droplets with constant stirring while heated under an effective reflux system to retain the propylene oxide. The reaction was carried out at various periods varying from one to ten hours. The reaction vessel was weighed after the apparatus was cooled and saturated with nitrogen. From growth in the reaction mixture mass, the amount of propylene oxide that has been reacted as well as the average degree of propoxylation was calculated.49 The surfactants I, II and II were obtained with molar ratios of 5, 10, and 15, respectively (Fig. 1).
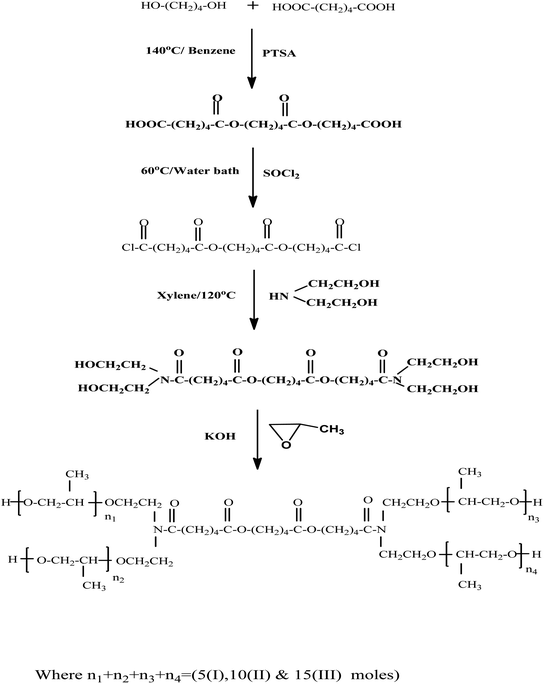 |
| Fig. 1 Synthesis procedure of nonionic Gemini surfactants. | |
2.2 Physical properties
2.2.1 Surface tension (γ). The surface parameters of aqueous solutions of surfactants I, II & III were studied at 25 °C. The values of critical micelle concentration (CMC) were determined via surface tension measuring employing a tensiometer K6 device (Krüss Company, Germany) with ring method measurements (±0.2 mN m−1). To establish equilibrium, the solution was kept for 30 min before measuring. Each measurement was carried out three times, with a 5 minutes interval between each measurement, and the average was obtained and used for subsequent studies.52
2.2.2 Critical micelle concentration (CMC). The inflection points in the γ versus [log
C] graphs (with of uncertainty 0.05–0.1 mM) matched to the CMCs correspond to the aqueous solutions of compounds I, II, and III at 25 °C.53
2.3 Corrosion study
2.3.1 Weight-loss. Titration against a standard solution of Na2CO3 was used to adjust the concentration of HCl carbon steel (C45) with composition of: (C 0.42–0.50%, P 0.045% max, Mn 0.50–0.80%, S 0.045% max and Si 0.15–0.40%) was used in the present study. Rectangular coupons of carbon steel with dimensions of (1 cm, 1 cm, 0.1 cm) were used in the gravimetric measurements. Coupons that were examined were abraded to a mirror shine using different grades of emery papers, rinsed with distilled water followed by acetone then inserted in the test solution. Every experiment has been conducted three times and the average value was recorded. The inhibition efficacy (η%) and the proportion of surface area that is covered by surfactants molecules (θ) have been calculated the usage of the subsequent equations, respectively: |
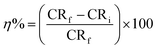 | (1) |
|
 | (2) |
where CRf and CRi stand respectively for corrosion rate (g cm−1 h−1) in the uninhibited and inhibitor-containing solutions.
2.3.2 Electrochemical polarization. A three-electrodes cell was used for conducting the electrochemical testing. A foil of platinum was a counter electrode while the reference one is a saturated calomel electrode. A rode of carbon steel became buried in a glass tube with Araldite, leaving an 0.1 cm2 backside facet uncovered to contact the corrosive medium was the working electrode. The working electrode naked tip was abraded utilizing emery papers with different grades, cleaned with distilled water, followed by acetone, dried using filter paper, and then inserted into the test solution. Before the measurements began, the steel electrode was kept in the tested solution for 10 min till it reached the value of its steady-state potential. At 25 °C, the corrosion parameters were monitored using a Metrohm potentiostat and Nova software at a 2.0 mVs−1 scan rate. The inhibition efficacy (η%) was calculated usage of the subsequent equation: |
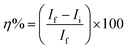 | (3) |
where, If and Ii, stand for corrosion current density of the uninhibited and inhibited solutions.Electrochemical impedance spectroscopy was applied on C-steel, in free HCl solution (1.0 M), and those inhibited by specific concentrations of the three tested surfactants. The technique was running with alternative current signals of 10 mV peak to peak amplitude over a range of frequency 105 Hz to 0.1 Hz. Both Nyquist and Bode spectra have been registered using Nova software associated with the Metrohm potentiostat. In Nyquist representation, the polarization resistance (RP) was derived from the diameter of the semicircle.
The inhibition efficacy (η%) was figured from polarization resistance (RP) values the use of the subsequent equation:
|
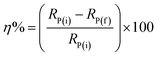 | (4) |
RP(f) & RP(i) stand respectively for polarization resistance of free and inhibited solutions.
2.4 Surface scanning
Using atomic force microscopy (AFM; Pico SPM-Picoscan 2100, Molecular Imaging, Arizona, AZ, USA), the carbon steel surface morphology was investigated. C-steel coupons left for 24 hours in the tested solutions containing and free of 10−3 M of I, II, and III surfactants and were taken to surface examination.
2.5 Computational details
The structure of the three synthesized surfactants were optimized using density functional theory (DFT). The B3LYP functional54 and 3-21g* basis set is used for optimization in gas and aqueous media. All calculations were done by G09 code.55 For the aqueous medium, the CPCM model was used.56
The quantum parameters such as the highest occupied molecular orbital (HOMO), the lowest unoccupied molecular orbital (LUMO), energy gap (ΔE). The global hardness (η), softness (σ), and the fraction of electron transferred (ΔN) which calculated using the following equations:
|
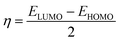 | (5) |
|
 | (6) |
|
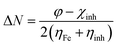 | (7) |
where
φ,
χinh are the work function (4.82 eV) and the electronegativity of inhibitors, respectively.
ηFe,
ηinh are the hardness of Fe (110) (0 eV) and hardness of inhibitors, respectively.
3. Results and discussion
3.1 FTIR spectrum
The structures of 5-{[6-(4-carboxybutoxy)-6-oxohexanoyl]oxy}pentanoic acid show absorption bands at 3320 cm−1 assigned to the (OH) group, 2910 and 2840 cm−1 ascribed to CH aliphatic, 1728 cm−1 assigned to C
O of the ester group, 1468 cm−1 C–H bond of CH2 group, and 1160 cm−1 ascribed to stretching of the C–O group (ESI† S1).
The structures of 1,6-bis(5-chloro-5-oxopentyl)hexanedioate show absorption bands at 2925 cm−1 ascribed to CH aliphatic, 1723 cm−1 assigned to C
O of the ester group, 1455 cm−1 C–H bond of CH2 group, and 1155 cm−1 ascribed to stretching of C–O group.
The structures of 1,6-bis({4-[bis(2-hydroxyethyl)carbamoyl]butyl})hexanedioate show absorption bands at 3360 cm−1 assigned to (OH) group, 2983 cm−1 ascribed to CH aliphatic, 1735 cm−1 assigned to C
O of the ester group, 1565 cm−1 assigned to stretching of C
O, 1470 cm−1 C–H bond of CH2 group, and 1180 cm−1 ascribed to stretching of C–O group (ESI† S2).
FTIR spectra were used to deduce the synthesized surfactants structures. The FTIR spectrum (Fig. 2A) of the synthesized nonionic Gemini surfactant (I) showed the following absorption bands at 3386 cm−1 were assigned to (OH) group, 2870 cm−1 were ascribed to CH aliphatic, 1731 cm−1 assigned to C
O of the ester group, 1454 cm−1 C–H bond of CH2 group and 1088 cm−1 were ascribed to C–O–C of ether group.
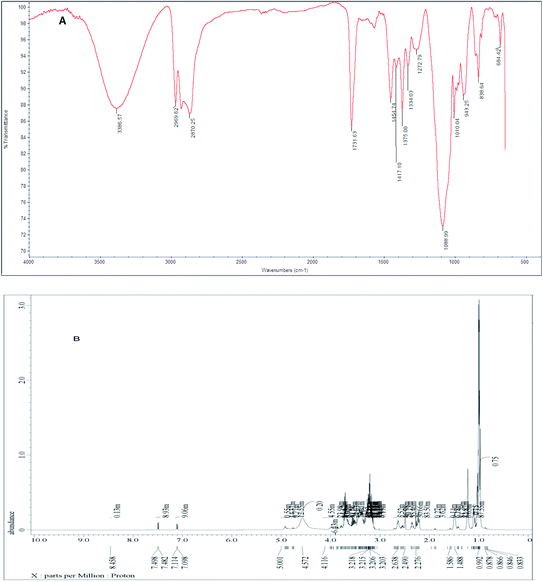 |
| Fig. 2 (A) FTIR spectra; (B) 1H-NMR of nonionic Gemini surfactants (I). | |
The FTIR spectrum of synthesized surfactant (II) displays absorption bands at 3340 cm−1 assigned to (OH) group, 2923 cm−1 ascribed to CH aliphatic, 1732 cm−1 assigned to C
O of the ester group, 1458 cm−1 C–H bond of CH2 group, and 1086 cm−1 ascribed to C–O–C of ether group (ESI† S3).
The FTIR spectrum of synthesized surfactant (III) displays absorption bands at 3464 cm−1 assigned to (OH) group, 2918 cm−1 ascribed to CH aliphatic, 1740 cm−1 assigned to C
O of the ester group, 1472 cm−1 C–H bond of CH2 group, and 1088 cm−1 ascribed to C–O–C of ether group (ESI† S4).
3.2 1H-NMR spectra
1H-NMR (DMSO-d6) spectrum of the produced nonionic Gemini surfactants (I) (Fig. 2B) showed different peaks at δ = 0.878 ppm (t, 12H of –CH3); δ = 1.166 ppm (m, 16H of 2(C
2)4); δ = 1.488 ppm (m, 8H of N–C
2); δ = 2.638 ppm (t, 8H of C
2–O–C
O); δ = 3.203–3.218 ppm (m, 12H of repeated propylene oxide units); and δ = 7.114 ppm (broad S, 4H of OH).
The 1H-NMR (DMSO-d6) spectrum of the synthesized nonionic Gemini surfactants (II) showed different peaks at δ = 0.855 ppm (t, 12H of –CH3); δ = 1.314 ppm (m, 16H of 2(C
2)4); δ = 1.73 ppm (m, 8H of N–C
2); δ = 2.499 ppm (t, 8H of C
2–O–C
O); δ = 3.304–3.728 ppm (m, 12H of repeated propylene oxide units); and δ = 7.95 ppm (broad S, 4H of OH). (ESI† S5).
The 1H-NMR (DMSO-d6) spectrum of the produced nonionic Gemini surfactants (III) showed different peaks at δ = 0.853 ppm (t, 12H of –CH3); δ = 1.004 ppm (m, 16H of 2(C
2)4); δ = 1.244 ppm (m, 8H of N–C
2); δ = 2.498 ppm (t, 8H of C
2–O–C
O); δ = 3.133–3.575 ppm (m, 12H of repeated propylene oxide units); and δ = 7.944 ppm (broad S, 4H of OH). (ESI† S6).
3.3 Physical properties
(CMC) values were derived from Fig. 3 and presented in Table 1. The lowest concentration at which surfactant monomers begin to aggregate and produce micelles is the (CMC) value. Values of surface pressure, maximum surface excess concentration, and minimum surface area occupied by one molecule of surfactant were calculated using eqn (8)–(10).57,58where (γ0) stands for pure water surface tension and (πCMC) is surfactant solution surface tension, at critical micelle concentration. |
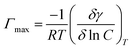 | (9) |
where δγ is surface pressure in mN m−1, and C is surfactant concentration. (δγ/δ
ln
C)T is the slope of the plot of surface tension and concentration curves below CMC at a constant temperature.
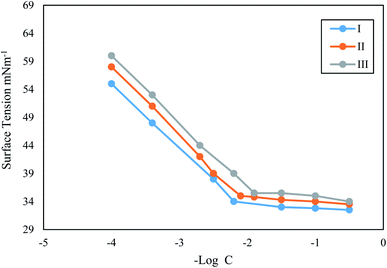 |
| Fig. 3 Dependence of surface tension on the concentration of the tested surfactants. | |
Table 1 Surface properties of the synthesized Gemini surfactants
Comp. |
Surface tension (mN m−1) 0.1 wt% at 25 °C |
γCMC (mN m−1) |
πCMC (mN m−1) |
CMC (mol L−1) |
Γmax × 10−10 (mol m−2) |
Amin (nm2 mol−1) |
I |
32.0 |
33 |
39 |
0.110 |
8.698 |
19.08 |
II |
33.5 |
35 |
37 |
0.122 |
8.298 |
20.20 |
III |
34.0 |
35 |
37 |
0.149 |
7.663 |
21.66 |
N is the Avogadro's number, πCMC, Γmax, and Amin. Values of the nonionic Gemini surfactants are shown in Table 1. The effectiveness of surfactants decreases as surface tension rises at a critical micelle concentration. The nonionic Gemini surfactant III possesses the highest Amin value, whilst surfactant I possess the lowest.
3.4 Corrosion study
3.4.1 Gravimetry measurements. Gravimetric experiments were carried out at various exposure time intervals with varying doses of the three produced surfactants inhibitors in a 1.0 M HCl solution. The information in Table 2 displays that the three surfactant compounds act as suitable inhibitors, wherein the inhibition performance will increase with the growing inhibitor's concentration. The inhibition performance for the three examined compounds increases withinside the order: I < II < III. Thus, this result could be related to the variety of propylene oxide units contained withinside the surfactant's molecule. As the propylene oxide units increase the molecular length increases covering a higher surface area and thus its inhibitive power increases.
Table 2 Corrosion parameters of C-steel in free and inhibited 1.0 M HCl solutions, as evaluated by weight loss. (CR) is the corrosion rate in g cm−1 h−1
Comp. |
t, h |
Free |
1 × 10−5 |
5 × 10−5 |
1 × 10−4 |
5 × 10−4 |
1 × 10−3 |
CR |
CR |
η% |
CR |
η% |
CR |
η% |
CR |
η% |
CR |
η% |
I |
12 |
0.514 |
0.402 |
22 |
0.316 |
38 |
0.199 |
61 |
0.173 |
66 |
0.161 |
69 |
24 |
0.614 |
0.401 |
35 |
0.294 |
52 |
0.198 |
68 |
0.173 |
72 |
0.159 |
74 |
36 |
0.723 |
0.400 |
45 |
0.288 |
60 |
0.197 |
73 |
0.172 |
76 |
0.158 |
78 |
48 |
0.822 |
0.387 |
53 |
0.283 |
65 |
0.197 |
76 |
0.171 |
79 |
0.157 |
81 |
60 |
0.955 |
0.386 |
60 |
0.271 |
71 |
0.197 |
79 |
0.170 |
82 |
0.157 |
83 |
72 |
1.062 |
0.385 |
64 |
0.271 |
74 |
0.197 |
81 |
0.162 |
85 |
0.156 |
85 |
94 |
1.188 |
0.384 |
68 |
0.266 |
77 |
0.196 |
83 |
0.161 |
86 |
0.155 |
87 |
106 |
1.301 |
0.383 |
71 |
0.255 |
80 |
0.196 |
85 |
0.160 |
88 |
0.154 |
88 |
118 |
1.447 |
0.382 |
74 |
0.255 |
82 |
0.195 |
86 |
0.158 |
89 |
0.153 |
89 |
130 |
1.607 |
0.381 |
76 |
0.249 |
84 |
0.194 |
88 |
0.158 |
90 |
0.152 |
90 |
II |
12 |
0.514 |
0.436 |
15 |
0.359 |
30 |
0.233 |
55 |
0.182 |
64 |
0.147 |
71 |
24 |
0.614 |
0.413 |
33 |
0.357 |
42 |
0.221 |
64 |
0.176 |
71 |
0.139 |
77 |
36 |
0.723 |
0.400 |
45 |
0.352 |
51 |
0.221 |
69 |
0.171 |
76 |
0.130 |
81 |
48 |
0.822 |
0.384 |
53 |
0.341 |
58 |
0.210 |
74 |
0.165 |
80 |
0.137 |
83 |
60 |
0.955 |
0.378 |
60 |
0.335 |
65 |
0.198 |
79 |
0.152 |
84 |
0.136 |
86 |
72 |
1.062 |
0.361 |
66 |
0.310 |
71 |
0.193 |
82 |
0.150 |
86 |
0.135 |
87 |
94 |
1.188 |
0.333 |
72 |
0.277 |
77 |
0.182 |
85 |
0.143 |
88 |
0.135 |
89 |
106 |
1.301 |
0.298 |
77 |
0.243 |
81 |
0.176 |
86 |
0.140 |
89 |
0.134 |
90 |
118 |
1.447 |
0.269 |
81 |
0.223 |
84 |
0.160 |
89 |
0.135 |
91 |
0.133 |
91 |
130 |
1.607 |
0.245 |
85 |
0.214 |
87 |
0.154 |
90 |
0.132 |
92 |
0.132 |
92 |
III |
12 |
0.514 |
0.381 |
26 |
0.211 |
59 |
0.171 |
67 |
0.152 |
70 |
0.139 |
73 |
24 |
0.614 |
0.380 |
38 |
0.210 |
66 |
0.170 |
72 |
0.151 |
75 |
0.138 |
78 |
36 |
0.723 |
0.380 |
47 |
0.208 |
71 |
0.169 |
76 |
0.150 |
79 |
0.131 |
82 |
48 |
0.822 |
0.377 |
54 |
0.207 |
75 |
0.167 |
79 |
0.147 |
82 |
0.129 |
84 |
60 |
0.955 |
0.376 |
61 |
0.205 |
78 |
0.164 |
83 |
0.1468 |
85 |
0.129 |
87 |
72 |
1.062 |
0.375 |
65 |
0.204 |
81 |
0.159 |
85 |
0.144 |
86 |
0.128 |
88 |
94 |
1.188 |
0.374 |
71 |
0.203 |
83 |
0.158 |
87 |
0.142 |
88 |
0.127 |
90 |
106 |
1.301 |
0.373 |
71 |
0.202 |
84 |
0.157 |
88 |
0.141 |
89 |
0.126 |
91 |
118 |
1.447 |
0.372 |
74 |
0.201 |
86 |
0.156 |
89 |
0.137 |
90 |
0.125 |
92 |
130 |
1.607 |
0.371 |
77 |
0.200 |
87 |
0.149 |
91 |
0.136 |
91 |
0.124 |
93 |
Fig. 4 shows the dependence of inhibition power on surfactant (I) concentration. The same trends for surfactants II and III can be seen in (S7 and S8,† respectively). It is clear from this curve that inhibition power increases by increasing inhibitor concentrations. It is important to notice that the increment of inhibition power achieves its minimum rate as the inhibitor concentration reaches 10−4 M. This result suggests that just a very small concentration of adsorbed inhibitor molecules is needed to cover all the available sites on the metal surface. Thus, it could be concluded that every inhibitor molecule adsorbs at the surface of the steel in this type of manner that covers the highest possible surface area. The molecules of the surfactant are expected to adsorb horizontally at the steel surface in the currently tested compounds.
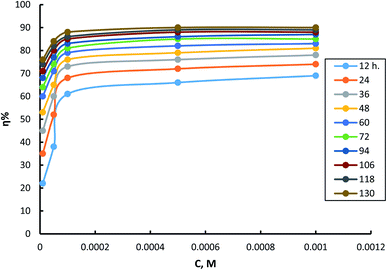 |
| Fig. 4 Dependence of inhibition efficiency on surfactant I concentration at different times of exposure. | |
The effect of exposure duration on the inhibitory power of different concentrations of surfactant (I) is shown in Fig. 5. The same figures for surfactants II & III are in (S9 and S10†). The figure shows that as the exposure time progresses on, inhibition efficiency improves. The increase in inhibition efficiency occurs at a rate that is higher in the cases of using low concentrations than using high concentrations. The increase in inhibition efficiency by increasing exposure time can be attributed, in all cases, to the presence of many surface sites available for adsorption at exposure start. Thus, over time, the number of surface sites occupied due to adsorption increases, and consequently the number of available surface sites decreases. It is obvious that with increasing inhibitor concentration at the start of exposure, the number of surface sites saturated with adsorbed molecules increases. So, the rate of inhibition efficiency increment is much lower in the case of high inhibitor concentrations than in lower ones.
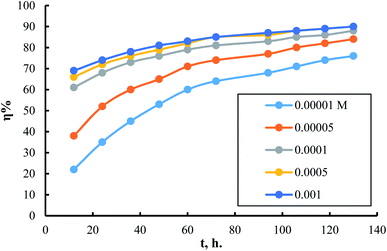 |
| Fig. 5 Dependence of inhibition efficiency on time of exposure for different concentrations of surfactant I. | |
3.4.2 Potentiodynamic polarization. Fig. 6 displays polarization curves of a C-steel electrode in 1.0 M HCl free as well as inhibited by different surfactant (I) concentrations. Surfactants II and III have almost the same figures (S11 and S12†). When the surfactants are added, the polarization curves change towards less negative potentials and lower current densities, as shown in Fig. 6. This indicates that the surfactants investigated had an inhibiting effect on steel corrosion in an acidic medium.
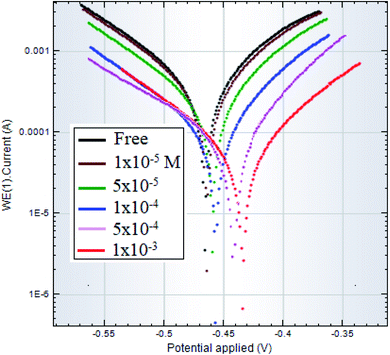 |
| Fig. 6 Polarization curves of C-steel in 1.0 M HCl solutions free and inhibited by different concentrations of surfactant I. | |
Table 3 lists the data retrieved from polarization curves, containing corrosion current, corrosion potential, cathodic Tafel constant, anodic Tafel constant, in addition to inhibition efficiency. According to the results of Table 3, the studied surfactants behave as inhibitors of mixed type. The fact that the presence of the surfactant has almost no effect on the values of both Tafel constants leads to this conclusion. Besides, it indicates that the corrosion mechanism did not change by adding the inhibitor. Furthermore, the addition of the surfactant only mildly changes the corrosion potential toward less negative values. This shift of corrosion potential suggests also a mixed-type inhibition mechanism that act predominantly as anodic inhibitors.
Table 3 Polarization characteristics of C-steel corrosion in 1.0 M HCl solutions free of and containing different concentrations of the three surfactants
Comp. |
Conc., (M) × 105 |
−Ecorr, mV (SCE) |
βa, mV per decade |
−βc, mV per decade |
icorr, μA cm−2 |
η% |
Free |
467 |
90 |
85 |
306.36 |
— |
I |
1 |
459 |
88 |
66 |
88.09 |
71.25 |
5 |
455 |
91 |
64 |
65.48 |
78.63 |
10 |
452 |
96 |
68 |
59.91 |
80.44 |
50 |
449 |
99 |
55 |
51.86 |
83.07 |
100 |
452 |
98 |
61 |
49.58 |
83.81 |
II |
1 |
463 |
89 |
75 |
87.65 |
71.45 |
5 |
458 |
92 |
59 |
80.08 |
73.86 |
10 |
453 |
86 |
73 |
43.41 |
85.83 |
50 |
465 |
97 |
91 |
22.75 |
92.57 |
100 |
461 |
91 |
85 |
21.84 |
92.87 |
III |
1 |
460 |
92 |
78 |
38.31 |
87.50 |
5 |
452 |
93 |
82 |
25.32 |
91.74 |
10 |
449 |
97 |
95 |
24.77 |
91.91 |
50 |
445 |
99 |
73 |
19.25 |
93.72 |
100 |
460 |
94 |
81 |
18.56 |
93.94 |
Further checking of the Table 3, it is apparent that increasing the surfactant concentration increases the inhibitory efficiency. The inhibitive power increases in the following order for all three compounds tested: I < II < III. This sequence is the same as that the weight-loss technique revealed.
3.4.3 Impedance spectroscopy. Fig. 7 represents the carbon steel impedance spectra in 1.0 M HCl free as well as inhibited by different surfactant (I) concentrations, as well as the fitted equivalent circuit. Similar spectra corresponding to surfactants (II) and (III) are in the ESI† (S13 and S14, respectively). The Nyquist plot (Fig. 7a) shows depressed semicircles which have centers below the true axis. Increasing the surfactant concentration leads to an increase in the semicircle diameter.The equivalent circuit is shown in Fig. 7b. This result is evidence that the surfactant inhibits carbon steel acid corrosion. Moreover, this implies that acid steel corrosion is primarily a charge transfer process.59 Fig. 7c represents the Bode plot which illustrates the dependence of impedance on the frequency. The figure clearly shows that carbon steel impedance increases by several orders of magnitude in inhibited acid solutions compared to free acid solutions. Impedance value increased as the surfactant's concentration is increased. Fig. 7d shows a peak of phase angle which increases and shifts toward low-frequency values with increasing surfactant concentration. It was stated that this shift in phase angle is attributed to the change of the metal interfacial structure owing to the development of surfactant molecules adsorbed layer.60
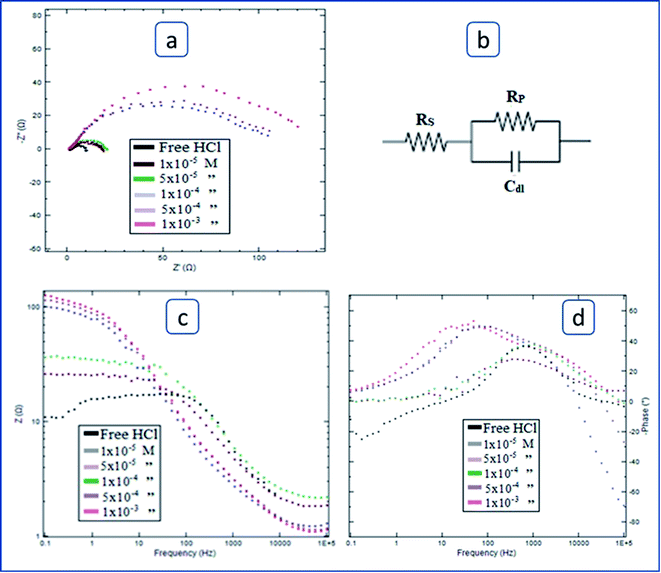 |
| Fig. 7 Carbon steel impedance spectra in 1.0 M HCl solutions without and with varying amounts of surfactant I. (a) Nyquist plot (b) equivalent circuit (c) Bode plot (d) frequency – phase angle relation. | |
Table 4 contains the impedance parameters related to the corrosion process obtained from calculations based on the equivalent circuit that has been fitted, as shown in Fig. 7b. The data of the table show that the polarization resistance, and thus the inhibition power, increases as the concentration of surfactant is increased. It is worth noticing that the studied surfactants' inhibitory efficacy increases in the same sequence as that of weight loss and polarization techniques: I < II < III.
Table 4 Impedance characteristics of carbon steel in 1.0 M HCl solutions, devoid of and containing different concentrations of the tested compounds
Compound |
Conc., (M) × 105 |
RS (Ω) |
RP (Ω) |
η% |
N |
Free |
3.20 |
11.00 |
— |
0.99885 |
Free |
3.20 |
11.00 |
— |
0.99885 |
|
I |
1 |
1.41 |
18.14 |
38.89 |
0.99509 |
5 |
1.49 |
22.31 |
50.00 |
0.99396 |
10 |
1.56 |
104.22 |
89.42 |
0.99301 |
50 |
1.47 |
112.15 |
90.17 |
0.99198 |
100 |
1.39 |
122.32 |
90.98 |
0.99474 |
II |
1 |
1.74 |
23.88 |
35.93 |
0.99509 |
5 |
2.01 |
34.46 |
68.07 |
0.9946 |
10 |
1.25 |
105.80 |
89.60 |
0.99429 |
50 |
1.69 |
118.48 |
90.71 |
0.99454 |
100 |
1.67 |
128.53 |
91.44 |
0.99485 |
III |
1 |
1.68 |
19.31 |
43.03 |
0.9942 |
5 |
1.39 |
96.19 |
88.56 |
0.99296 |
10 |
1.66 |
106.96 |
89.71 |
0.99466 |
50 |
1.94 |
121.55 |
90.95 |
0.99413 |
100 |
1.96 |
138.35 |
92.05 |
0.99434 |
3.5 Adsorption behavior
The adsorption of inhibitor molecules on the metal surface is the first stage in the inhibition process. At the metal/corrosive media interface, the inhibitor molecules form an isolating film. Adsorbed molecules maybe still with their chemical identity or combine with the dissolved metal cations, at the interface, forming a new chemical compound. The former case is called physical adsorption while the latter is chemical adsorption. The adsorption isotherm could be used to accurately describe the adsorption process's characteristics. A variety of adsorption isotherms were examined in this study using the results of weight loss studies. The Langmuir isotherm model is revealed to be the most suitable for the current data. As per the Langmuir isotherm model, the surface energy is influenced by the portion of the surface covered by inhibitor molecules (θ). Moreover, it assumes that there are no interactions between the molecules that have been adsorbed. The following equation describes the isotherm: |
 | (11) |
C denotes molar surfactant concentration, θ represents surface covering fraction while k is adsorption constant which is identified as:
|
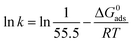 | (12) |
Δ
G0adsdenotes standard adsorption free energy while the numerical value 55.5 refers to water molar concentration.
Fig. 8 shows a relation between the surfactant's molar concentration
C and
C/
θ. The Langmuir isotherm of adsorption is confirmed by straight lines with unit value slopes. The calculated values of the standard adsorption free energies for the three surfactants are outlined in the
Table 5.
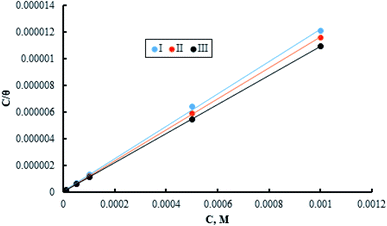 |
| Fig. 8 Langmuir adsorption isotherm for the three surfactants. | |
Table 5 Standard free energy of adsorption
Surfactant |
−ΔG0ads, kJ mol−1 |
I |
49.90 |
II |
51.61 |
III |
53.89 |
Data in the Table 5 shows negative standard free energy values for all the tested surfactants. This indicates that the surfactant molecule's adsorption at the steel surface is a spontaneous process. Moreover, their values decline in the sequence: I > II > III, reflecting the tendency for adsorption process which in turn reflects the inhibitive capability. Therefore, this sequence is consistent with that obtained for inhibition efficiency.
3.6 Atomic force microscopy
Atomic force microscopy is a research instrument that provides information on the topography of a surface. In corrosion research, using such a methodology is advantageous because it gives a lot of data about the surface roughness of the metal under investigation. 2-D and 3-D photos of C-steel surface after exposition to 1.0 M HCl uninhibited solutions and inhibited by 10−3 M of each of surfactants examined shown in Table 6. The original-sized photos, as well as the associated comprehensive surface data, are available in (S15–S22†). Visual examination of the images reveals clearly that any surfactant presence significantly reduces the roughness of the carbon steel surface. Roughness diminishes in the following manner, depending on the sort of additives:
Table 6 2-D and 3-D images of the carbon steel surface after exposure to 1.0 M HCl free of and containing 10−3M of the tested surfactants
Free acid > free acid + I > free acid + II > free acid + III.
Table 7 shows the roughness of C-steel samples after one day of exposition to 1.0 M HCl solutions containing 1 × 10−3 M from every one of the tested surfactants at 25 °C. Roughness average (Sa), peak height (Sp), and valley depth (Sv) are all displayed in the table. Table 7 shows that as progress from a free acid solution to an inhibited acid solution, all the measured values decline according to the following sequence:
Table 7 AFM roughness data of carbon steel surface after one-day exposure to 1.0 M HCl solutions free of and contains 1 × 10−3 M of surfactants (I–III), at 25 °C
|
Free acid |
I |
II |
III |
Area, pm2 |
100.8 |
100.8 |
100.8 |
100.8 |
Sa |
978.76 nm |
180.67 nm |
164.18 nm |
133.22 nm |
Sp |
2710.1 nm |
1145.1 nm |
1332.2 nm |
704.85 nm |
Sv |
−5.0681 μm |
−1090.6 nm |
−560.59 nm |
−565.41 nm |
Free acid > free acid + I > free acid + II > free acid + III.
The addition of any of the three tested surfactants in the corrosive medium results in a smoother carbon steel surface. The surfactant molecules adsorbed on the carbon steel surface are responsible for this result. As a result, the effectiveness of the surfactant increases in the same way as that achieved by previous techniques: III > II > I.
3.7 Quantum calculations
The optimized geometry of the three studied inhibitors were represented in Fig. 9. The Frontier molecular orbitals (HOMO and LUMO) and molecular electrostatic potentials (MEP) were seen in Fig. 10. The energies of HOMO and LUMO indicate electron-donating of the inhibitor to vacant 3d orbital of iron, and electron-accepting ability from the partially filled metal orbital, respectively.61,62 Therefore, a high value of HOMO energy and a low value of LUMO energy is associated with high inhibition efficiency. As shown in Table 8, inhibitor AE-15 in an aqueous medium has a higher value of HOMO and a lower LUMO value which gives the highest inhibition efficiency. The HOMO and LUMO orbitals of the three inhibitor molecules as seen in Fig. 10, it can be found that the HOMO electrons density is distributed on amid group in the three corrosion inhibitors, while the LUMO electron density is distributed over the ester group.
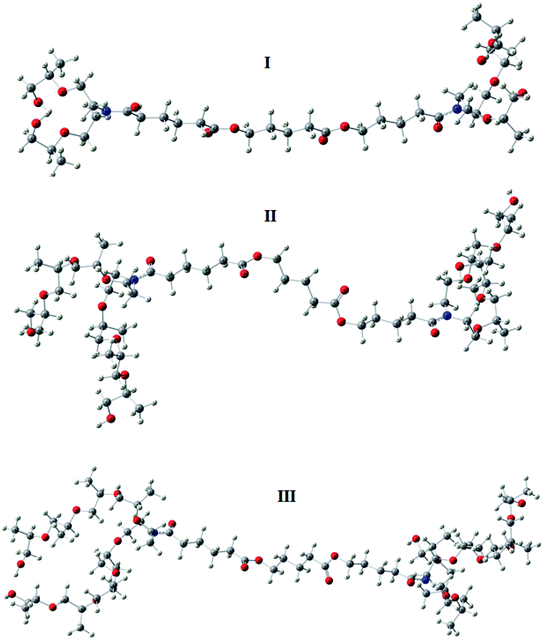 |
| Fig. 9 The optimized geometries of the surfactant compounds. | |
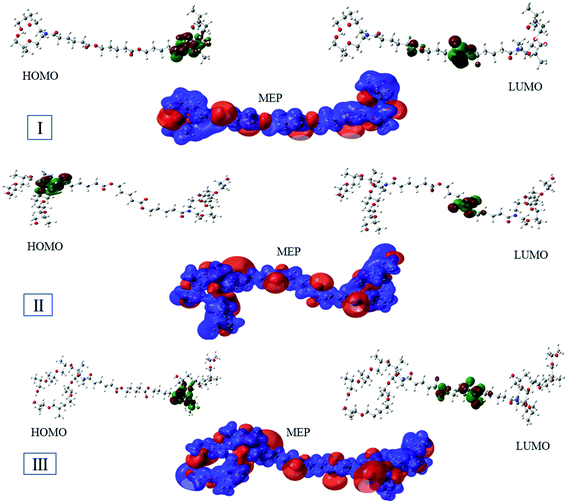 |
| Fig. 10 The Frontier orbitals and molecular electrostatic potentials of the surfactant compounds. | |
Table 8 Quantum parameters of the surfactant compounds
|
Gas phase |
Aqueous phase |
I |
II |
III |
I |
II |
III |
EHOMO (eV) |
−5.89 |
−5.45 |
−5.32 |
−5.97 |
−5.88 |
−5.83 |
ELUMO (eV) |
0.46 |
0.51 |
0.59 |
0.31 |
0.27 |
0.26 |
ΔE (eV) |
6.35 |
5.96 |
5.91 |
6.28 |
6.15 |
6.09 |
η (eV) |
3.18 |
2.98 |
2.96 |
3.14 |
3.07 |
3.04 |
σ (eV−1) |
0.31 |
0.34 |
0.34 |
0.32 |
0.33 |
0.33 |
ΔN (eV) |
0.33 |
0.39 |
0.42 |
0.32 |
0.33 |
0.33 |
The energy gap ΔE is another descriptor that benefits from assessing the inhibitor's reactivity to the metal surface.63 The chemical reactivity is inversely proportional to the energy gap.64 The calculated values of ΔE under investigation are listed in Table 8, which can show the trends of ΔE for the three inhibitors as follow: I > II > III in both gas and aqueous media which agrees with the experimental results.
Also, global softness and hardness are important properties for measuring reactivity and molecular stability and can adsorb when adjacent to the metal surface. The inhibitors with a high value of softness and low value of hardness have a high capacity to adsorb on metal surfaces.65,66 As listed in Table 8, the III inhibitors possess a higher value of softness that have the highest inhibition efficiency.
The fraction of electrons transferred (ΔN), from Lukovits's study, which shows that the inhibition efficiency is due to the donation of electrons.67 If ΔN <3.6, the inhibition efficiency increases by increasing the electron donation of an inhibitor to the metal surface. As listed in Table 8, that III has the highest positive value of ΔN in the gas and aqueous phases which is compatible with the experimental result.
MEP is considered an important indicator that reflects the reactive sites of electrophilicity and nucleophilicity. The blue and red regions indicate the positive and negative electrostatic potentials, respectively. As shown in Fig. 10, where the negative electrostatic potential is concentrated on the oxygen and nitrogen atoms, and the positive electrostatic potential is located on the whole region of the inhibitor molecules.
The molecular structure containing heterogeneous atoms undergo protonation in acidic solutions. The nitrogen atoms of the amide group become a preferred site for protonation which have a higher negative charge derived by Milliken atomic charges. As shown in Fig. 11, the HOMO and LUMO of the unprotonated compared with protonated form. The HOMO values for the three investigated inhibitors decrease which suggests lowering affinity to donate electrons. LUMO values decrease indicating a higher tendency of accepting electrons. The bandgap in the protonated form has lower values than unprotonated suggesting the inhibitors become easily adsorbed on the metal surface in protonated form.
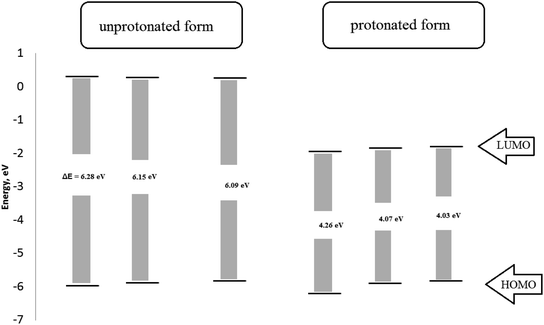 |
| Fig. 11 The Frontier orbital energies of protonated and unprotonated form for the studied surfactants. | |
3.8 Inhibition mechanism
Results obtained from this study could be summarized in the following:
- The tested three Gemini surfactants showed high inhibition performance as for C-steel acid corrosion. With increasing concentration, the efficiency of inhibition improves up to a definite value after which it remains almost constant.
- A very small concentration of the surfactant is quite enough for reaching the most attainable value of efficiency.
- The efficiency of inhibition increases with increasing the exposure time. The dependence of efficiency of inhibition on exposure time is higher for low surfactants concentrations than higher ones.
- The efficiency of inhibition rises in the sequence: I < II < III illustrating how the molecule's length affects the process.
- The tested surfactants behave as mixed-type inhibitors.
- The calculated parameters obtained from DFT agree with the experimental results.
Based on the information acquired thus far, a conclusion has been reached about the corrosion inhibition mechanism. As the carbon steel is brought into contact with the inhibited acid solution, the surfactant molecules adsorb at anodic as well as cathodic locations on the steel surface. By blocking the transmission of charge and mass between steel and the corrosive medium, the adsorbed molecules form an insulating layer retarding both anodic and cathodic half-reactions. The surfactant's molecules adsorb horizontally covering high surface areas, so the maximum available sites were occupied just by a very small concentration of the inhibitor based on the number of surfactant molecules, the high surfactant concentrations achieve almost their highest inhibition effect at a relatively small exposure time. On the other hand, surfactants with lower concentrations still depend on exposure time to bring enough molecules from the bulk solution to the interface region.
Gemini surfactants with their unique molecular structure that has oxygen and nitrogen atoms having lone pairs of electrons dispersed throughout their skeletons. This configuration encourages the surfactant molecules to horizontal adsorption on the steel surface. The comparatively high Amin values (Table 1) additionally support this adsorption mode. In such an adsorption route, the inhibitor molecules can cover a high surface area of the steel through relatively low concentration.
4. Conclusion
Three novel Gemini surfactants based on adipic acid were synthesized with different numbers of propylene oxide units. Their structures were characterized by IR and NMR spectra. Experimentally, their physical properties were determined. Detailed experiments were conducted to determine their inhibitory effects on carbon steel acid corrosion. It was found that all of them can retard steel corrosion with high efficiencies. The inhibition process was found to be dependent on the surfactant concentration, exposure time, and molecular length. The theoretical calculation made to correlate their inhibition effect with their structures agreed with the experimental results and shed more light on the mechanism of their action.
Author contributions
Mohamed Deef Allah was involved in the synthesis of the surfactants and made the measurements of their physical properties. Samar Abdelhamed and Mona A. El-Etre were involved in the corrosion measurements and wrote the discussion of the results as well as the inhibition mechanism part. Kamal A. Soliman was involved in the theoretical calculation and made the correlation between the structures and the inhibition efficiency. All the authors share in writing, review and editing the manuscript.
Conflicts of interest
The authors declare no financial or commercial conflict of interest.
References
- U. M. Angst, Challenges and opportunities in corrosion of steel in concrete, Mater. Struct., 2018, 51, 4 CrossRef.
- G. Koch, Cost of corrosion, trends in oil and gas corrosion research and technologies, Elsevier, 2017, pp. 3–30 Search PubMed.
- P. Pedeferri, General Principles of Corrosion, Corrosion Science and Engineering, Springer, 2018, pp. 1–16 Search PubMed.
- S. O. Pehkonen and S. Yuan, Tailored Thin Coatings for Corrosion Inhibition Using a Molecular Approach, Elsevier Science, 2018 Search PubMed.
- E. Shekari, F. Khan and S. Ahmed, Economic risk analysis of pitting corrosion in process facilities, Int. J. Pressure Vessels Piping, 2017, 157, 51–62 CrossRef.
- A. Altin, M. Rohwerder and A. Erbe, Cyclodextrins as carriers for organic corrosion inhibitors in organic coatings, J. Electrochem. Soc., 2017, 164, C128 CrossRef CAS.
- B. E. Brycki, I. H. Kowalczyk, A. Szulc, O. Kaczerewska and M. Pakiet, Organic Corrosion Inhibitors, Principles and Recent Applications, InTech Open, 2017 Search PubMed.
- R. S. Erami, M. Amirnasr, S. Meghdadi, M. Talebian, H. Farrokhpour and K. Raeissi, Carboxamide derivatives as new corrosion inhibitors for mild steel protection in hydrochloric acid solution, Corros. Sci., 2019, 151, 190–197 CrossRef.
- M. Goyal, S. Kumar, I. Bahadur, C. Verma and E. E. Ebenso, Organic corrosion inhibitors for industrial cleaning of ferrous and non-ferrous metals in acidic solutions: A review, J. Mol. Liq., 2018, 256, 565–573 CrossRef CAS.
- L. Guo, I. B. Obot, X. Zheng, X. Shen, Y. Qiang, S. Kaya and C. Kaya, Theoretical insight into an empirical rule about organic corrosion inhibitors containing nitrogen, oxygen, and sulfur atoms, Appl. Surf. Sci., 2017, 406, 301–306 CrossRef CAS.
- L. Guo, C. Qi, X. Zheng, R. Zhang, X. Shen and S. Kaya, Toward understanding the adsorption mechanism of large size organic corrosion inhibitors on an Fe (110) surface using the DFTB method, RSC Adv., 2017, 7, 29042–29050 RSC.
- S. Haddadi, S. Ramazani, M. Mahdavian, P. Taheri and J. Mol, Fabrication and characterization of graphene-based carbon hollow spheres for encapsulation of organic corrosion inhibitors, Chem. Eng. J., 2018, 352, 909–922 CrossRef CAS.
- M. A. Quraishi, D. S. Chauhan and V. S. Saji, Heterocyclic Organic Corrosion Inhibitors: Principles and Applications, Elsevier, 2020 Search PubMed.
- P. B. Raja, M. Ismail, S. Ghoreishiamiri, J. Mirza, M. C. Ismail, S. Kakooei and A. A. Rahim, Reviews on corrosion inhibitors: a short view, Chem. Eng. Commun., 2016, 203, 1145–1156 CrossRef CAS.
- H. Singh, Y. Kurapati and S. Sharma, Aggregation and Adsorption Behavior of Organic Corrosion Inhibitors studied using Molecular Simulations, NACE Corrosion Conference, 2019 Search PubMed.
- C. Verma, J. Haque, M. Quraishi and E. E. Ebenso, Aqueous phase environmental friendly organic corrosion inhibitors derived from one step multicomponent reactions: a review, J. Mol. Liq., 2019, 275, 18–40 CrossRef CAS.
- C. Verma, L. Olasunkanmi, E. E. Ebenso and M. Quraishi, Substituents effect on corrosion inhibition performance of organic compounds in aggressive ionic solutions: a review, J. Mol. Liq., 2018, 251, 100–118 CrossRef CAS.
- Z. Wang, Y. Gong, C. Jing, H. Huang, H. Li, S. Zhang and F. Gao, Synthesis of dibenzotriazole derivatives bearing alkylene linkers as corrosion inhibitors for copper in sodium chloride solution: A new thought for the design of organic inhibitors, Corros. Sci., 2016, 113, 64–77 CrossRef CAS.
- D. A. Winkler, Predicting the performance of organic corrosion inhibitors, Metals, 2017, 7, 553 CrossRef.
- K. Xhanari and M. Finšgar, Organic corrosion inhibitors
for aluminium and its alloys in acid solutions: a review, RSC Adv., 2016, 6, 62833–62857 RSC.
- K. Xhanari and M. Finšgar, Organic corrosion inhibitors for aluminum and its alloys in chloride and alkaline solutions: a review, Arabian J. Chem., 2019, 12, 4646–4663 CrossRef CAS.
- U. Eduok, E. Ohaeri and J. Szpunar, Electrochemical and surface analyses of X70 steel corrosion in simulated acid pickling medium: Effect of poly(N-vinyl imidazole) grafted carboxymethyl chitosan additive, Electrochim. Acta, 2018, 278, 302–312 CrossRef CAS.
- N. Iroha and A. Hamilton-Amachree, Adsorption and anticorrosion performance of Ocimum Canum extract on mild steel in sulphuric acid pickling environment, Am. J. Mater. Sci., 2018, 8, 39–44 Search PubMed.
- A.-R. I. Mohammed, M. M. Solomon, K. Haruna, S. A. Umoren and T. A. Saleh, Evaluation of the corrosion inhibition efficacy of Cola acuminata extract for low carbon steel in simulated acid pickling environment, Environ. Sci. Pollut. Res., 2020, 1–19 Search PubMed.
- E. Naveen, B. V. Ramnath, C. Elanchezhian and S. M. Nazirudeen, Influence of organic corrosion inhibitors on pickling corrosion behaviour of sinter-forged C45 steel and 2% Cu alloyed C45 steel, J. Alloys Compd., 2017, 695, 3299–3309 CrossRef CAS.
- M. Tourabi, K. Nohair, M. Traisnel, C. Jamam and F. Bentiss, Electrochemical and XPS studies of the corrosion inhibition of carbon steel in hydrochloric acid pickling solutions by 3,5-bis(2-thinylmethyl)-4-amino-1,2,4 triazole, Corros. Sci., 2013, 75, 123–133 CrossRef CAS.
- M. M. Khalaf, A. H. Tantawy, K. A. Soliman and H. M. Abd El-Lateef, Cationic gemini-surfactants based on waste cooking oil as new ‘green’inhibitors for N80-steel corrosion in sulphuric acid: a combined empirical and theoretical approaches, J. Mol. Struct., 2020, 1203, 127442 CrossRef CAS.
- M. Saleh, M. G. Mahmoud and H. M. Abd El-Lateef, Comparative study of synergistic inhibition of mild steel and pure iron by 1-hexadecylpyridinium chloride and bromide ions, Corros. Sci., 2019, 154, 70–79 CrossRef CAS.
- T. Han, J. Guo, Q. Zhao, Y. Wu and Y. Zhang, Enhanced corrosion inhibition of carbon steel by pyridyl gemini surfactants with different alkyl chains, Mater. Chem. Phys., 2020, 240, 122156 CrossRef CAS.
- F. E.-T. Heakal, M. Deyab, M. Osman, M. Nessim and A. Elkholy, Synthesis and assessment of new cationic gemini surfactants as inhibitors for carbon steel corrosion in oilfield water, RSC Adv., 2017, 7, 47335–47352 RSC.
- O. Kaczerewska, R. Leiva-Garcia, R. Akid and B. Brycki, Efficiency of cationic gemini surfactants with 3-azamethylpentamethylene spacer as corrosion inhibitors for stainless steel in hydrochloric acid, J. Mol. Liq., 2017, 247, 6–13 CrossRef CAS.
- O. Kaczerewska, R. Leiva-Garcia, R. Akid, B. Brycki, I. Kowalczyk and T. Pospieszny, Effectiveness of O-bridged cationic gemini surfactants as corrosion inhibitors for stainless steel in 3 M HCl: Experimental and theoretical studies, J. Mol. Liq., 2018, 249, 1113–1124 CrossRef CAS.
- S. M. Tawfik, Ionic liquids based gemini cationic surfactants as corrosion inhibitors for carbon steel in hydrochloric acid solution, J. Mol. Liq., 2016, 216, 624–635 CrossRef CAS.
- A. E. Elkholy and F. E.-T. Heakal, Electrochemical measurements and semi-empirical calculations for understanding adsorption of novel cationic Gemini surfactant on carbon steel in H2SO4 solution, J. Mol. Struct., 2018, 1156, 473–482 CrossRef CAS.
- F. E.-T. Heakal and A. E. Elkholy, Gemini surfactants as corrosion inhibitors for carbon steel, J. Mol. Liq., 2017, 230, 395–407 CrossRef CAS.
- M. H. Sliem, M. Afifi, A. B. Radwan, E. M. Fayyad, M. F. Shibl, F. E.-T. Heakal and A. M. Abdullah, AEO7 surfactant as an eco-friendly corrosion inhibitor for carbon steel in HCl solution, Sci. Rep., 2019, 9, 1–16 CAS.
- H. M. Abd El-Lateef, K. Shalabi and A. H. Tantawy, Corrosion inhibition and adsorption features of novel bioactive cationic surfactants bearing benzenesulphonamide on C1018-steel under sweet conditions: Combined modeling and experimental approaches, J. Mol. Liq., 2020, 320, 114564 CrossRef CAS.
- W. S. Abdrabo, B. Elgendy, K. A. Soliman, H. M. Abd El-Lateef and A. H. Tantawy, Synthesis, assessment and corrosion protection investigations of some novel peptidomimetic cationic surfactants: Empirical and theoretical insights, J. Mol. Liq., 2020, 315, 113672 CrossRef CAS.
- A. H. Tantawy, K. A. Soliman and H. M. Abd El-Lateef, Novel synthesized cationic surfactants based on natural piper nigrum as sustainable-green inhibitors for steel pipeline corrosion in CO2-3.5% NaCl: DFT, Monte Carlo simulations and experimental approaches, J. Cleaner Prod., 2020, 250, 119510 CrossRef CAS.
- H. M. Abd El-Lateef, A. H. Tantawy and A. A. Abdelhamid, Novel Quaternary Ammonium-Based Cationic Surfactants: Synthesis, Surface Activity and Evaluation as Corrosion Inhibitors for C1018 Carbon Steel in Acidic Chloride Solution, J. Surfactants Deterg., 2017, 20, 735–753 CrossRef CAS.
- H. M. Abd El-Lateef, K. Shalabi and A. H. Tantawy, Corrosion inhibition of carbon steel in hydrochloric acid solution using newly synthesized urea-based cationic fluorosurfactants: experimental and computational investigations, New J. Chem., 2020, 44, 17791–17814 RSC.
- H. M. Abd El-Lateef, K. A. Soliman, M. A. Al-Omair and M. S. S. Adam, A combination of modeling and experimental approaches to investigate the novel nicotinohydrazone Schiff base and its complexes with Zn(II) and ZrO(II) as inhibitors for mild-steel corrosion in molar HCl, J. Taiwan Inst. Chem. Eng., 2021, 120, 391–408 CrossRef.
- H. U. Nwankwo, L. O. Olasunkanmi and E. E. Ebenso, Experimental, quantum chemical and molecular dynamic simulations studies on the corrosion inhibition of mild steel by some carbazole derivatives, Sci. Rep., 2017, 7, 1–18 CrossRef CAS PubMed.
- D. Wang, S. Li, Y. Ying, M. Wang, H. Xiao and Z. Chen, Theoretical and experimental studies of structure and inhibition efficiency of imidazoline derivatives, Corros. Sci., 1999, 41, 1911–1919 CrossRef CAS.
- A. E. Elkholy, F. E.-T. Heakal, A. M. Rashad and K. Zakaria, Monte Carlo simulation for guar and xanthan gums as green scale inhibitors, J. Pet. Sci. Eng., 2018, 166, 263–273 CrossRef CAS.
- F. E.-T. Heakal, S. Rizk and A. Elkholy, Characterization of newly synthesized pyrimidine derivatives for corrosion inhibition as inferred from computational chemical analysis, J. Mol. Struct., 2018, 1152, 328–336 CrossRef.
- F. E.-T. Heakal, M. Deyab, M. Osman and A. Elkholy, Performance of Centaurea cyanus aqueous extract towards corrosion mitigation of carbon steel in saline formation water, Desalination, 2018, 425, 111–122 CrossRef.
- N. A. Negm, N. G. Kandile and M. A. Mohamad, Synthesis, characterization and surface activity of new eco-friendly Schiff bases vanillin derived cationic surfactants, J. Surfactants Deterg., 2011, 14, 325–331 CrossRef CAS.
- M. H. Ahmed, P. Sallay, I. Rusznak and L. Farkas, Determination of the average ethoxylation degree, Tenside, Surfactants, Deterg., 1996, 33, 410–411 CrossRef CAS.
- M. Mohamed, D. Ismail and A. Mohamed, Synthesis and evaluation of new amphiphilic polyethylene glycol-based triblock copolymer surfactants, J. Surfactants Deterg., 2005, 8, 175–180 CrossRef CAS.
- Y. I. Estrin, V. Komratova, G. Estrina, V. Lodygina and B. Rozenberg, Selectivity of acylation of ethanolamines with (meth)acryloyl chlorides, Russ. J. Appl. Chem., 2008, 81, 135–143 CrossRef CAS.
- M. Migahed, N. Negm, M. Shaban, T. Ali and A. Fadda, Synthesis, characterization, surface and biological activity of diquaternary cationic surfactants containing ester linkage, J. Surfactants Deterg., 2016, 19, 119–128 CrossRef CAS.
- M. Abd El-Ghaffar, M. Sherif and A. T. El-Habab, Synthesis, characterization, and evaluation of ethoxylated lauryl-myrisityl alcohol nonionic surfactants as wetting agents, anti-foamers, and minimum film forming temperature reducers in emulsion polymer lattices, J. Surfactants Deterg., 2017, 20, 117–128 CrossRef CAS.
- C. Lee, W. Yang and R. Parr, Phys. Rev. B: Condens. Matter Mater. Phys., 1988, 785–789 CrossRef CAS PubMed.
- M. J. Frisch, G. W. Trucks, H. B. Schlegel, G. E. Scuseria, M. A. Robb, J. R. Cheeseman, G. Scalmani, V. Barone, B. Mennucci and G. A. Petersson, et al., R.A. Gaussian09, 1, Gaussian, Inc., Wallingford CT, 2009, vol. 121, pp. 150–166 Search PubMed.
- V. Barone and M. Cossi, Quantum calculation of molecular energies and energy gradients in solution by a conductor solvent model, J. Phys. Chem. A, 1998, 102, 1995–2001 CrossRef CAS.
- M. El Hefnawy and M. D. Allah, Synthesis and evaluation of nonionic polymeric surfactants based on acrylated polyethylene glycol, J. Surfactants Deterg., 2013, 16, 161–171 CrossRef.
- D. Singh and A. Dey, Synergistic effects of inorganic and organic cations on inhibitive performance of propargyl alcohol on steel dissolution in boiling hydrochloric acid solution, Corrosion, 1993, 49, 594–600 CrossRef CAS.
- F. Bentiss, M. Lagrenee, M. Traisnel and J. Hornez, The corrosion inhibition of mild steel in acidic media by a new triazole derivative, Corros. Sci., 1999, 41, 789–803 CrossRef CAS.
- Y.-J. Tan, S. Bailey and B. Kinsella, An investigation of the formation and destruction of corrosion inhibitor films using electrochemical impedance spectroscopy (EIS), Corros. Sci., 1996, 38, 1545–1561 CrossRef CAS.
- B. Xu, Y. Ji, X. Zhang, X. Jin, W. Yang and Y. Chen, Experimental and theoretical studies on the corrosion inhibition performance of 4-amino-N,N-di-(2-pyridylmethyl)-aniline on mild steel in hydrochloric acid, RSC Adv., 2015, 5, 56049–56059 RSC.
- D. Kumar, V. Jain and B. Rai, Imidazole derivatives as corrosion inhibitors for copper: a DFT and reactive force field study, Corros. Sci., 2020, 171, 108724 CrossRef CAS.
- A. Zarrouk, I. El Ouali, M. Bouachrine, B. Hammouti, Y. Ramli, E. Essassi, I. Warad, A. Aouniti and R. Salghi, Theoretical approach to the corrosion inhibition efficiency of some quinoxaline derivatives of steel in acid media using the DFT method, Res. Chem. Intermed., 2013, 39, 1125–1133 CrossRef CAS.
- I. Obot and Z. Gasem, Theoretical evaluation of corrosion inhibition performance of some pyrazine derivatives, Corros. Sci., 2014, 83, 359–366 CrossRef CAS.
- H. Allal, Y. Belhocine and E. Zouaoui, Computational study of some thiophene derivatives as aluminium corrosion inhibitors, J. Mol. Liq., 2018, 265, 668–678 CrossRef CAS.
- N. a. Kovačević and A. Kokalj, DFT study of interaction of azoles with Cu (111) and Al (111) surfaces: role of azole nitrogen atoms and dipole–dipole interactions, J. Phys. Chem. C, 2011, 115, 24189–24197 CrossRef.
- I. Lukovits, E. Kalman and F. Zucchi, Corrosion inhibitors—correlation between electronic structure and efficiency, Corrosion, 2001, 57, 3–8 CrossRef CAS.
Footnote |
† Electronic supplementary information (ESI) available. See DOI: 10.1039/d1ra07449k |
|
This journal is © The Royal Society of Chemistry 2021 |
Click here to see how this site uses Cookies. View our privacy policy here.