DOI:
10.1039/D1RA07238B
(Paper)
RSC Adv., 2021,
11, 37083-37088
Nickel(II)-catalyzed reductive silylation of alkenyl methyl ethers for the synthesis of alkyl silanes†
Received
28th September 2021
, Accepted 25th October 2021
First published on 18th November 2021
Abstract
A new one pot protocol has been developed for the reductive silylation of alkenyl methyl ethers using Et3Si–BPin and HSiEt3 with nickel(II) catalyst. Styrene type methyl ethers, multi-substituted vinyl methyl ethers, heterocycles and unconjugated vinyl ethers are all tolerated to form alkyl silanes. Mechanistic study reveals that it is a cascade of a C–O bond silylation and vinyl double bond hydrogenation process. Internal nucleophilic substitution or oxidative addition pathways were both acceptable for C–O bond cleavage. The acquired intermediate alkenyl silanes then proceeded through an unconventional reduction process thus providing alkyl silanes.
Introduction
Organosilanes, prominently with their physical and chemical properties, have significant applications in organic syntheses,1 drug discovery,2 bioactive compound preparations,3 and advanced material developments.4 Alkyl silanes, as one of the principal research areas among organosilanes, are used as isosteres of quaternary carbons, which have found significant applications in pharmaceutical research (Fig. 1).5 Therefore, it has attracted much attention on their synthesis. Hydrosilylations of olefins using Pt, Rh, Pd, or Ru catalysts are pioneering works.6 Recently, instead of noble metal catalysts, several kinds of base metal catalysts such as Fe, Co, Ni, and Mn have been developed for this transformation, generally with elegant catalyst design.7 Other means including carbene insertion into silanes,8 cross-coupling with organosilicon reagents,9 or direct C(sp3)–H silylation10 all contribute to provide alkyl silanes.
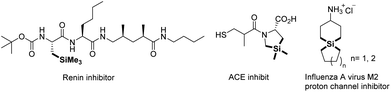 |
| Fig. 1 Pharmaceutical active molecules with tetraalkyl silane skeletons. | |
Due to the significant progress made by transition metal catalyzed carbon–heteroatom bond formation via unactivated bond cleavage in synthetic chemistry, especially for C–O bond activation,11 deoxygenative silylation has been applied for acquiring of multiple silicon compounds. Martin et al. reported the vital work in 2014 that Ni/Cu catalyzed C–O bond silylation of aryl or benzyl pivalates with electron rich backbones in mild conditions.12 This methodology was also introduced to aryl esters which underwent decarbonylative silylation via acyl C–O bond activation by Rueping's and Shi's group.13 Carbamates as appropriate substrates were also discovered using nickel or iron catalysts.14 Different from esters, cut off the C–O bond of aryl ethers are more difficult because of the high bond energy. Martin et al. developed the first nickel catalyzed silylation of aryl methyl ether compounds with silyl borate.15 Montgomery et al. then employed aryl silyl ethers as the source of C–O bond silylation.16 In addition, pyridine assisted silylation of aryl 2-pyridyl ethers with silyl zinc reagent were realised to generate aryl silanes.17 Alkyl ethers like benzyl methyl ethers or allyl methyl ethers were succeed as well in nickel catalyzed C–O bond silylation with silyl borate15 or silyl magnesium reagent18. However, vinyl C–O bond silylation was rarely reported, only two styrene type methyl ethers were tested affording alkenyl silicon compounds as the supplementary for silylation of conjugated ethers in Martin's work.15 In 2017, Studer et al. reported ring opening silylation of benzofurans which was similar to alkenyl ethers mediated by a silyl lithium reagent (Scheme 1a).19 Whereafter, Yorimitsu et al. reported CuCl catalyzed ring opening silylation of benzofurans with disilane that made the reaction more tolerable with functional groups (Scheme 1b). Also, an addition–elimination mechanism was proposed for C–O bond cleavage.20
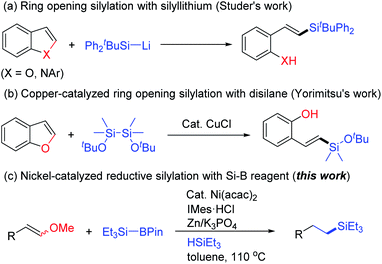 |
| Scheme 1 C–O bonds silylation of benzofurans and alkenyl methyl ethers. | |
Recently, our group has been interested in the valuable conversion of alkenyl ethers employing inexpensive transition metal catalysts.21 Combined with the high reactivity of nickel species in C–O bond activation, Ni(acac)2 catalyzed one pot reaction of demethoxylative silylation and olefin reduction were performed with silyl borate and hydrosilane (Scheme 1c), which offers an alternative process for late-stage functionalization of alkenyl ethers.
Results and discussion
Our investigation was started by testing the reaction of styrene methyl ether 1a with 2, a silyl borate usually applied for silylation reactions and easily accessible in bulk quantities.22 After careful optimization, we found a cocktail of cheap and air-stable catalyst Ni(acac)2 (10 mol%), N-heterocyclic carbene ligand IMes·HCl (10 mol%), catalytic amount of zinc powder (20 mol%), HSiEt3 (3 equiv.) and K3PO4 (1.5 equiv.) in toluene at 110 °C gave the best results, affording phenethyl silane 3a as the main product in 76% isolated yield. The ratio of 3a and double-bond reserved byproduct 4a was 97
:
3 (Table 1, entry 1). NiI2 instead of Ni(acac)2 gave a low yield of 3a and increased the amount of 4a (Table 1, entry 2). When using other nickel catalyst like Ni(OTf)2, obvious decrease of 3a took place (Table 1, entry 3). IMes·HCl was crucial for this transformation, other NHC or phosphine ligands showed little or no reaction of 1a (Table 1, entries 4 and 5). The reaction could not happen without nickel catalyst (Table 1, entry 6), which negated zinc's catalytic activity. While no Zn had little effect to this transformation which could be largely owing to the same reducing property of HSiEt3 (Table 1, entry 7). No reaction occurred when silyl borate was absent indicated that direct hydrosilylation followed by β-O elimination pathway was not possible (Table 1, entry 8). The additional base K3PO4 was essential and irreplaceable for this reaction. As shown in entries 9–12, no reaction was found with other sylvite, phosphate or other base indicating that a subtle balance was required for nucleophilicity and steric bulk of the base. Without HSiEt3, the yield of 3a dropped dramatically and 4a was obtained as the main product (Table 1, entry 13). A possible reason might be concluded from this result that 4a was an intermediate product and was hydrogenated by HSiEt3, and 4a could be partly reduced to 3a with little amount of H2O in reaction mixture when HSiEt3 was absent. To prove this, extra hydrogen sources such as H2O, MeOH and PhMe2SiH were added instead of HSiEt3, 4a was obtained discrepantly with relatively lower yields (Table 1, entries 14–16).
Table 1 Optimization of reaction conditionsa
With robust conditions in hand, we turned to exam the feasible scope and limitations for our Ni(acac)2 catalyzed reductive silylation of alkenyl methyl ethers. Styrene type methyl ethers were first carried out for alkyl silanes preparation (Table 2). Electron-donating group like methyl, tetra-butyl and phenyl at para- or meta-position were all tolerated providing up to 93% yield of products (3b–3e). When 2-pyridyl, a strong ortho-directing group, was fixed at para-position, 85% yield of 3f acquired under standard conditions without any C–H activation products detected. We are excited to find that aryl methyl ether which was readily for silylation through nickel catalyst in mild conditions15 showed no competition against vinyl methyl ether, yet affording 78% yield of 3g. Substituents with stronger electron donor property seemed to enhance the reactivity in this process. Some disubstituted or trisubstituted styrene methyl ethers all turned out to provide products with higher yields (3h–3k). Alkenyl methyl ether bearing benzodihydrofuran skeleton (1l) also gave a good result. Fluorine-containing groups such as F (3m) or CF3 (3n) were proved having no impact on this reaction. The chemical selectivity of this transformation were also demonstrated by employing substrates installing with ester or amide group (3o, 3p), which were deeply explored in nickel catalyzed C–O bond13 or C–N bond23 activations. Substrates with sterically hindered o-Me and o-OMe substituents were compatible enough to afford 3q and 3r in 47% and 71% yield respectively. Several π-extended alkenyl methyl ethers underwent this process with high reactivity thus providing 90% yield of (naphthalen-2-yl)ethyl silane (3s) and 85% yield of (naphthalen-1-yl)ethyl silane (3t). Even bulky 9-anthryl ethyl silane (3u) was obtained with 60% yield. To our delight, this reaction was not limited to simple disubstituted alkenyl methyl ethers, trisubstituted alkenyl methyl ethers could result in structurally diverse alkyl silanes in moderate yields (3v, 3w). Besides, aryl dienyl methyl ether (1x) reacted as well providing 3x through dual reductive silylation process with high reactivity.
Table 2 Scope of styrene type methyl ethersa
Reaction conditions: 1 (0.3 mmol), 2 (0.6 mmol), HSiEt3 (0.9 mmol) and K3PO4 (0.45 mmol) were reacted in toluene (1.5 mL) at 110 °C for 24 h under nitrogen atmosphere. Yields were obtained after purification through column chromatography on silica gel. React for 48 h. 20 mol% Ni(acac)2, 20 mol% IMes·HCl, 40 mol% Zn, 1 (0.3 mmol), 2 (1.2 mmol), HSiEt3 (1.8 mmol) and K3PO4 (0.9 mmol) were reacted in toluene (3 mL) at 110 °C for 24 h under nitrogen atmosphere. |
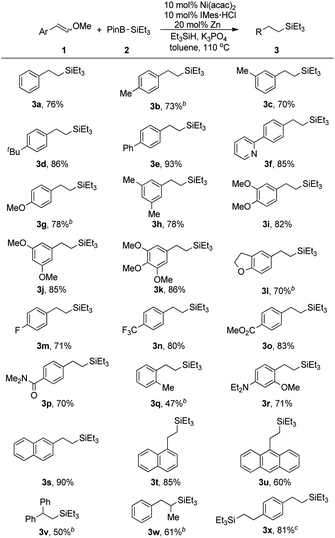 |
In addition of the styrene type methyl ethers, we are interested in the transformation containing heteromatic skeletons. Because of the striking impact exhibited by heteroatoms in hydrosilylation process, alkyl silicon compounds containing heteroatoms were really hard to obtain. In our conditions, several type of heteroaryl vinyl methyl ethers were tested. As shown in Table 3, furan was tolerated leading to the preparation of 6a in 64% yield. While 6b was obtained in a low yield probably because the competitive side reaction took place on the C–O bond of the benzofuran ring. N-heterocycles were more reactive and corresponding silanes containing heterocyclic ring such as pyridine, imidazole, pyrimidine, quinoline, indole, pyrrole, and carbazole were all acquired with good results (6c–6h, 6k, 6l). It was worth noting that unprotected indole products 6i and 6j were obtained without observably decline in yields implying the efficiency of this C–Si bond forming strategy.
Table 3 Scope of heterocycle conjugated vinyl methyl ethersa
Reaction conditions: 5 (0.3 mmol), 2 (0.6 mmol), HSiEt3 (0.9 mmol) and K3PO4 (0.45 mmol) were reacted in toluene (1.5 mL) at 110 °C for 24 h under nitrogen atmosphere. Yields were obtained after purification through column chromatography on silica gel. React for 48 h. |
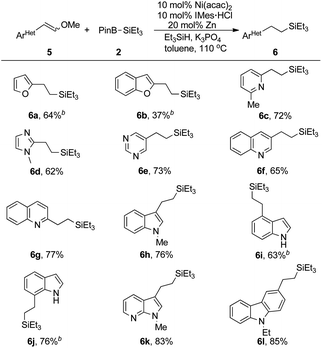 |
In order to excavate the applicability of this transformation, some unconjugated alkenyl methyl ethers were put into reaction (Scheme 2). Disubstituted alkenyl ethers were suitable for this reaction affording 8a and 8b with good results. Yet 8c and 8d was obtained in a lower yield presumably due to the steric effect caused by trisubstituted alkenyl methyl ethers.
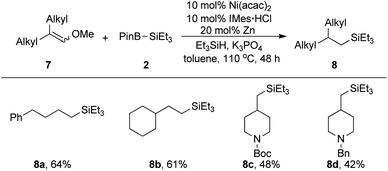 |
| Scheme 2 Reductive silylation with alkyl substituted vinyl methyl ethers. Conditions: 7 (0.3 mmol), 2 (0.6 mmol), HSiEt3 (0.9 mmol) and K3PO4 (0.45 mmol) were reacted in toluene (1.5 mL) at 110 °C for 48 h under nitrogen atmosphere. | |
Subsequently, some mechanistic studies were carried out to give a closer understanding of this process. Compounds E-4a and Z-4a, acquired from known report,24 were investigated under standard conditions and 3a was obtained in our prospection with favourable results despite the original configuration of 4a. Nevertheless, styrene failed to generate 3a when employed under the same conditions (Scheme 3a). These all suggested that compound 4a was probably an intermediate product and a cascade of C–O bond silylation followed with olefin reduction was the possible reaction pathways rather than the way of hydrosilylation to the potential alkenes. All the time it has been difficult to distinguish the oxidative addition mechanism from addition–elimination mechanism in transition metal catalyzed inert bond activation. We tried two substrates 7e and 9, derived from which intramolecular competition might exist if Ni–X elimination process would happen when reacting with 2 under standard conditions, to test the feasibility of addition–elimination pathway for C–O bond silylation (Scheme 3b). Actually, after the reaction of 7e, no structurally dominant addition–(Ni–H elimination) product 8e′ nor further reductive product 8e′′ were detected. Specifically, when 9 was conducted under the same reaction conditions as 7e, only few amount of compounds 10 and 11 were detected by GC-MS while anticipated product 12 was undiscovered, which suggested that allylic C–O bond scission along with vinyl C–O bond scission occurred in this reaction. No addition–(Ni–OMe elimination) product 13 nor 14 were detected further illuminating the improbability of the Ni–O elimination pathway.
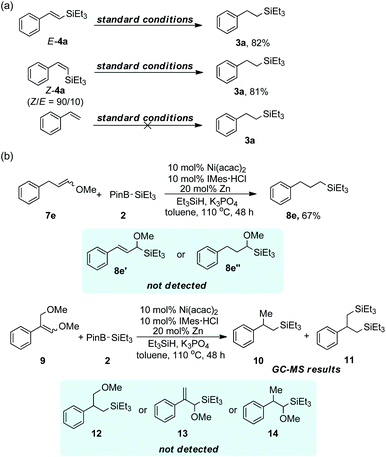 |
| Scheme 3 Mechanistic studies. | |
According to Martin's report,15 an internal nucleophilic substitution mechanism, supported by DFT calculations,25 was possible for C–O bond silylation. As in our process, K3PO4 was supposed to play an important role in the convention of 2 to either Et3SiK or [(Et3Si–Bpin)n(PO4)]K3 complex that might be regarded as a silyl anion surrogate. A tendency of a discrete [Ni(IMes)–SiEt3]K complex might be acquired when in situ generated Ni(0) species exposed to IMes·HCl, 2, and K3PO4. Unfortunately, isolation of the nickel complex or spectroscopic evidence for this suppositive intermediate was unavailable. Therefore, two feasible pathways were proposed in the C–O bond silylation procedure (Fig. 2). Classic oxidative addition mechanism was shown in path a, in which Ni(0) inserted into C–O bond followed by transmetalation with 2, reductive elimination then occurred forming the key intermediate 4a. Path b exhibited a constant Ni(0) mediated nucleophilic substitution mechanism, where Ni–Si complex C acted as a nucleophile, and methoxy from 1a was substituted by SiEt3 to provide intermediate 4a. Unconventional hydrogenation of 4a with HSiEt3 proceeded to generate 3a in this strategy, which was commonly seen as a side reaction appeared in transition metal catalyzed hydrosilylation of alkenes.26 The byproduct siloxane instead of disilane was isolated (see ESI† for details) which not only excluded the continues oxidative addition path of forming SiEt3–Ni–SiEt3 complex but also suggested trace amount of moisture in the reaction mixture maybe have some effect for this transformation.27
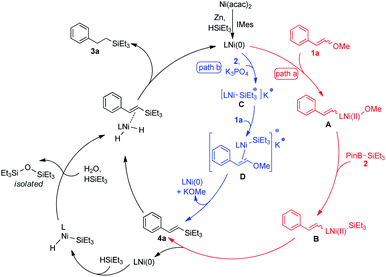 |
| Fig. 2 Probable reaction pathways. | |
Conclusions
In summary, we have provided a Ni(II) catalyzed one pot reaction of alkenyl methyl ethers with silyl borate through C–O bond silylation and olefin reduction process. This strategy is successfully implemented not only for electron-rich styrene methyl ethers and π-extended alkenyl methyl ethers, but also succeeded in generating alkyl silanes containing electron-deficient aryls, heterocycles, and unconjugated alkyl groups, that provides alternative approaches for carbon–hetero bond formation and extends the scope of unreactive C–O bond activations. Besides, a possible reaction mechanism including oxidative addition or internal nucleophilic substitution for C–O bond scission and nickel mediated double bond reduction by HSiEt3 is fully presented. Further mechanistic studies as well as functionalization of alkenyl or alkyl C–O bond are in progress in our lab.
Author contributions
X. Q. conceived the project and analysed the data. L. Z. and H. W. performed the experiments and analysed the data. L. L. helped with the substrates synthesis and analysed experimental data. Y. L. and Y. Z. participated in the mechanism discussion. The manuscript was written by X. Q. and Y. Z. contributed to the editing.
Conflicts of interest
There are no conflicts to declare.
Acknowledgements
This work was supported by the National Natural Science Foundation of China (22002063), Natural Science Foundation of Jiangsu Province (BK20200961), Natural Science Foundation of the Jiangsu Higher Education Institutions of China (19KJB150036), and the Science and Technology Program of Nantong (JC2019099).
Notes and references
-
(a) H. F. Sore, W. R. J. D. Galloway and D. R. Spring, Palladium-Catalysed Cross-Coupling of Organosilicon Reagents, Chem. Soc. Rev., 2012, 41, 1845 RSC;
(b) L. Li, Y. Zhang, L. Gao and Z. Song, Recent Advances in C–Si Bond Activation via a Direct Transition Metal Insertion, Tetrahedron Lett., 2015, 56, 1466 CrossRef CAS;
(c) T. Komiyama, Y. Minami and T. Hiyama, Recent Advances in Transition-Metal-Catalyzed Synthetic Transformations of Organosilicon Reagents, ACS Catal., 2017, 7, 631 CrossRef CAS.
- A. K. Franz and S. O. Wilson, Organosilicon Molecules with Medicinal Applications, J. Med. Chem., 2013, 56, 388 CrossRef CAS PubMed.
-
(a) L. Gai, J. Mack, H. Lu, T. Nyokong, Z. Li, N. Kobayashi and Z. Shen, Organosilicon Compounds as Fluorescent Chemosensors for Fluoride Anion Recognition, Coord. Chem. Rev., 2015, 285, 24 CrossRef CAS;
(b) E. Rémond, C. Martin, J. Martinez and F. Cavelier, Silicon-Containing Amino Acids: Synthetic Aspects, Conformational Studies, and Applications to Bioactive Peptides, Chem. Rev., 2016, 116, 11654 CrossRef PubMed.
-
(a) C. Sanchez, P. Belleville, M. Popall and L. Nicole, Applications of Advanced Hybrid Organic–Inorganic Nanomaterials: from Laboratory to Market, Chem. Soc. Rev., 2011, 40, 696 RSC;
(b) G. K. Min, D. Hernández and T. Skrydstrup, Efficient Routes to Carbon–Silicon Bond Formation for the Synthesis of Silicon-Containing Peptides and Azasilaheterocycles, Acc. Chem. Res., 2013, 46, 457 CrossRef CAS PubMed.
-
(a) B. Weidmann, (Trimethylsilyl)alanine: A Metabolically Stable “Bio-isostere” for Phenylalanine, Chimia, 1992, 46, 312 CAS;
(b) G. A. Dalkas, D. Marchand, F. Cavelier, J.-C. Galleyrand, J. Martinez, G. A. Spyroulias and P. Cordopatis, Study of a Lipophilic Captopril Analogue Binding to Angiotensin I Converting Enzyme, J. Pept. Sci., 2010, 16, 91 CrossRef CAS PubMed;
(c) J. Wang, C. Ma, Y. Wu, R. A. Lamb, L. H. Pinto and W. F. DeGrado, Exploring Organosilane Amines as Potent Inhibitors and Structural Probes of Influenza A Virus M2 Proton Channel, J. Am. Chem. Soc., 2011, 133, 13844 CrossRef CAS PubMed.
-
(a) D. Troegel and J. Stohrer, Recent Advances and Actual Challenges in Late Transition Metal Catalyzed Hydrosilylation of Olefins from an Industrial Point of View, Coord. Chem. Rev., 2011, 255, 1440 CrossRef CAS;
(b) Y. Nakajima and S. Shimada, Hydrosilylation Reaction of Olefins: Recent Advances and Perspectives, RSC Adv., 2015, 5, 20603 RSC.
-
(a) J. Y. Wu, B. N. Stanzl and T. Ritter, A Strategy for the Synthesis of Well-Defined Iron Catalysts and Application to Regioselective Diene Hydrosilylation, J. Am. Chem. Soc., 2010, 132, 13214 CrossRef CAS PubMed;
(b) Z. Mo, Y. Liu and L. Deng, Anchoring of Silyl Donors on a N-Heterocyclic Carbene through the Cobalt-Mediated Silylation of Benzylic C-H Bonds, Angew. Chem., Int. Ed., 2013, 52, 10845 CrossRef CAS PubMed;
(c) C. Chen, M. B. Hecht, A. Kavara, W. W. Brennessel, B. Q. Mercado, D. J. Weix and P. L. Holland, Rapid, Regioconvergent, Solvent-Free Alkene Hydrosilylation with a Cobalt Catalyst, J. Am. Chem. Soc., 2015, 137, 13244 CrossRef CAS PubMed;
(d) V. Srinivas, Y. Nakajima, W. Ando, K. Sato and S. Shimada, (Salicylaldiminato)Ni(ii)-Catalysts for Hydrosilylation of Olefins, Catal. Sci. Technol., 2015, 5, 2081 RSC;
(e) X. Jia and Z. Huang, Conversion of Alkanes to Linear Alkylsilanes Using an Iridium–Iron-Catalysed Tandem Dehydrogenation–Isomerization–Hydrosilylation, Nat. Chem., 2016, 8, 157 CrossRef CAS PubMed;
(f) C. H. Schuster, T. Diao, I. Pappas and P. J. Chirik, Bench-Stable, Substrate-Activated Cobalt Carboxylate Pre-Catalysts for Alkene Hydrosilylation with Tertiary Silanes, ACS Catal., 2016, 6, 2632 CrossRef CAS;
(g) J. H. Docherty, J. Peng, A. P. Dominey and S. P. Thomas, Activation and Discovery of Earth-Abundant Metal Catalysts Using Sodium tert-Butoxide, Nat. Chem., 2017, 9, 595 CrossRef CAS PubMed;
(h) B. Cheng, P. Lu, H. Zhang, X. Cheng and Z. Lu, Highly Enantioselective Cobalt-Catalyzed Hydrosilylation of Alkenes, J. Am. Chem. Soc., 2017, 139, 9439 CrossRef CAS PubMed;
(i) Z. Yang, D. Peng, X. Du, Z. Huang and S. Ma, Identifying a Cobalt Catalyst for Highly Selective Hydrosilylation of Allenes, Org. Chem. Front., 2017, 4, 1829 RSC;
(j) M.-Y. Hu, Q. He, S.-J. Fan, Z.-C. Wang, L.-Y. Liu, Y.-J. Mu, Q. Peng and S.-F. Zhu, Ligands with 1,10-Phenanthroline Scaffold for Highly Regioselective Iron-Catalyzed Alkene Hydrosilylation, Nat. Commun., 2018, 9, 221 CrossRef PubMed;
(k) R. Agahi, A. J. Challinor, J. Dunne, J. H. Docherty, N. B. Carterb and S. P. Thomas, Regiodivergent Hydrosilylation, Hydrogenation, [2π + 2π]-Cycloaddition and C–H Borylation Using Counterion Activated Earth-Abundant Metal Catalysis, Chem. Sci., 2019, 10, 5079 RSC;
(l) J. Dong, X.-A. Yuan, Z. Yan, L. Mu, J. Ma, C. Zhu and J. Xie, Manganese-Catalysed Divergent Silylation of Alkenes, Nat. Chem., 2021, 13, 182 CrossRef CAS PubMed.
-
(a) Z. Liu, J. Huo, T. Fu, H. Tan, F. Ye, M. L. Hossaina and J. Wang, Palladium(0)-Catalyzed C(sp3)–Si Bond Formation via Formal Carbene Insertion into a Si–H bond, Chem. Commun., 2018, 54, 11419 RSC;
(b) J. R. Jagannathan, J. C. Fettinger, J. T. Shaw and A. K. Franz, Enantioselective Si–H Insertion Reactions of Diarylcarbenes for the Synthesis of Silicon-Stereogenic Silanes, J. Am. Chem. Soc., 2020, 142, 11674 CrossRef CAS PubMed.
-
(a) C. K. Chu, Y. Liang and G. C. Fu, Silicon–Carbon Bond Formation via Nickel-Catalyzed Cross-Coupling of Silicon Nucleophiles with Unactivated Secondary and Tertiary Alkyl Electrophiles, J. Am. Chem. Soc., 2016, 138, 6404 CrossRef CAS PubMed;
(b) Y. Takeda, K. Shibuta, S. Aoki, N. Tohnai and S. Minakata, Catalyst-Controlled Regiodivergent Ring-Opening C(sp3)–Si Bond-Forming Reactions of 2-Arylaziridines with Silylborane Enabled by Synergistic Palladium/Copper Dual Catalysis, Chem. Sci., 2019, 10, 8642 RSC;
(c) S. Mallick, E.-U. Würthwein and A. Studer, Synthesis of Alkyl Silanes via Reaction of Unactivated Alkyl Chlorides and Triflates with Silyl Lithium Reagents, Org. Lett., 2020, 22, 6568 CrossRef CAS PubMed.
-
(a) C. Cheng and J. F. Hartwig, Catalytic Silylation of Unactivated C–H Bonds, Chem. Rev., 2015, 115, 8946 CrossRef CAS PubMed;
(b) B. Li and P. H. Dixneuf, Metal-Catalyzed Silylation of sp3C–H Bonds, Chem. Soc. Rev., 2021, 50, 5062 RSC.
-
(a) J. Cornella, C. Zarate and R. Martin, Metal-Catalyzed Activation of Ethers via C–O Bond Cleavage: A New Strategy for Molecular Diversity, Chem. Soc. Rev., 2014, 43, 8081 RSC;
(b) Z. Qiu and C.-J. Li, Transformations of Less-Activated Phenols and Phenol Derivatives via C–O Cleavage, Chem. Rev., 2020, 120, 10454 CrossRef CAS PubMed;
(c) T. B. Boit, A. S. Bulger, J. E. Dander and N. K. Garg, Activation of C–O and C–N Bonds Using Non-Precious-Metal Catalysis, ACS Catal., 2020, 10, 12109 CrossRef CAS PubMed.
- C. Zarate and R. Martin, A Mild Ni/Cu-Catalyzed Silylation via C–O Cleavage, J. Am. Chem. Soc., 2014, 136, 2236 CrossRef CAS PubMed.
-
(a) L. Guo, A. Chatupheeraphat and M. Rueping, Decarbonylative Silylation of Esters by Combined Nickel and Copper Catalysis for the Synthesis of Arylsilanes and Heteroarylsilanes, Angew. Chem., Int. Ed., 2016, 55, 11810 CrossRef CAS PubMed;
(b) X. Pu, J. Hu, Y. Zhao and Z. Shi, Nickel-Catalyzed Decarbonylative Borylation and Silylation of Esters, ACS Catal., 2016, 6, 6692 CrossRef CAS.
-
(a) V. Murugesan, V. Balakrishnan and R. Rasappan, Nickel-Catalyzed Cross-Coupling Reaction of Carbamates with Silylmagnesium Reagents, J. Catal., 2019, 377, 293 CrossRef CAS;
(b) J. Zhang, Y. Zhang, S. Geng, S. Chen, Z. Liu, X. Zeng, Y. He and Z. Feng, C–O Bond Silylation Catalyzed by Iron: A General Method for the Construction of Csp2–Si Bonds, Org. Lett., 2020, 22, 2669 CrossRef CAS PubMed.
- C. Zarate, M. Nakajima and R. Martin, A Mild and Ligand-Free Ni-Catalyzed Silylation via C–OMe Cleavage, J. Am. Chem. Soc., 2017, 139, 1191 CrossRef CAS PubMed.
- E. M. Wiensch, D. P. Todd and J. Montgomery, Silyloxyarenes as Versatile Coupling Substrates Enabled by Nickel-Catalyzed C–O Bond Cleavage, ACS Catal., 2017, 7, 5568 CrossRef CAS.
- Y.-Y. Konga and Z.-X. Wang, Nickel-Catalyzed Reaction of Aryl 2-Pyridyl Ethers with Silylzinc Chlorides: Silylation of Aryl 2-Pyridyl Ethers via Cleavage of the Carbon−Oxygen Bond, Adv. Synth. Catal., 2019, 361, 5440 CrossRef.
- V. Balakrishnan, V. Murugesan, B. Chindan and R. Rasappan, Nickel-Mediated Enantiospecific Silylation via Benzylic C–OMe Bond Cleavage, Org. Lett., 2021, 23, 1333 CrossRef CAS PubMed.
- P. Xu, E.-U. Wgrthwein, C. G. Daniliuc and A. Studer, Transition-Metal-Free Ring-Opening Silylation of Indoles and Benzofurans with (Diphenyl-tert-butylsilyl)lithium, Angew. Chem., Int. Ed., 2017, 56, 13872 CrossRef CAS PubMed.
- H. Saito, K. Nogi and H. Yorimitsu, Copper-Catalyzed Ring-Opening Silylation of Benzofurans with Disilane, Angew. Chem., Int. Ed., 2018, 57, 11030 CrossRef CAS PubMed.
- X. Qiu, Y. Li, L. Zhou, P. Chen, F. Li, Y. Zhang and Y. Ling, Nickel(II)-Catalyzed Borylation of Alkenyl Methyl Ethers via C–O Bond Cleavage, Org. Lett., 2020, 22, 6424 CrossRef CAS PubMed.
-
(a) T. A. Boebel and J. F. Hartwig, Iridium-Catalyzed Preparation of Silylboranes by Silane Borylation and Their Use in the Catalytic Borylation of Arenes, Organometallics, 2008, 27, 6013 CrossRef CAS;
(b) J.-J. Feng, W. Mao, L. Zhang and M. Oestreich, Activation of the Si–B Interelement Bond Related to Catalysis, Chem. Soc. Rev., 2021, 50, 2010 RSC.
- S.-C. Lee, L. Guo, H. Yue, H.-H. Liao and M. Rueping, Nickel-Catalyzed Decarbonylative Silylation, Borylation, and Amination of Arylamides via a Deamidative Reaction Pathway, Synlett, 2017, 28, 2594 CrossRef CAS.
-
(a) R. Xu and C. Cai, FeCl2/DTBP: An Efficient and Highly E-Selective Cross-Coupling of Silanes with Styrene and Its Derivatives, Catal. Commun., 2018, 107, 5 CrossRef CAS;
(b) V. S. Sridevi, W. Y. Fan and W. K. Leong, Stereoselective Hydrosilylation of Terminal Alkynes Catalyzed by [Cp*IrCl2]2: A Computational and Experimental Study, Organometallics, 2007, 26, 1157 CrossRef CAS.
-
(a) H. Ogawa, H. Minami, T. Ozaki, S. Komagawa, C. Wang and M. Uchiyama, How and Why Does Ni0 Promote Smooth Etheric C-O Bond Cleavage and C-C Bond Formation? A Theoretical Study, Chem.–Eur. J., 2015, 21, 13904 CrossRef CAS PubMed;
(b) B. Wang, Q. Zhang, J. Jiang, H. Yu and Y. Fu, Mechanistic Study on Nickel-Catalyzed Silylation of Aryl Methyl Ethers, Chem.–Eur. J., 2017, 23, 17249 CrossRef PubMed;
(c) P. Jain, S. Pal and V. Avasare, Ni(COD)2-Catalyzed ipso-Silylation of 2-Methoxynaphthalene: A Density Functional Theory Study, Organometallics, 2018, 37, 1141 CrossRef CAS.
-
(a) F. Burgos, I. Chávez, J. M. Manriquez and M. Valderrama, A New Heterobimetallic Ru, Rh Complex with a Dianionic Pentalene as Bridging Ligand. Synthesis, Crystal Structure, and Catalytic Activity of [Cp*Ru(μ-η5,η3-C8H6)Rh(η4-COD)], Organometallics, 2001, 20, 1287 CrossRef CAS;
(b) J. Li, C. Yang, Y. Bai, X. Yang, Y. Liu and J. Peng, The Effect of an Acylphosphine Ligand on the Rhodium-Catalyzed Hydrosilylation of Alkenes, J. Organomet. Chem., 2018, 855, 7 CrossRef CAS;
(c) W. Lu, C. Li, X. Wu, X. Xie and Z. Zhang, [Rh(COD)Cl]2/PPh3-Catalyzed Dehydrogenative Silylation of Styrene Derivatives with NBE as a Hydrogen Acceptor, Organometallics, 2020, 39, 3780 CrossRef CAS.
- S. Pattanaik and C. Gunanathan, Cobalt-Catalyzed Selective Synthesis of Disiloxanes and Hydrodisiloxanes, ACS Catal., 2019, 9, 5552 CrossRef CAS.
Footnote |
† Electronic supplementary information (ESI) available. See DOI: 10.1039/d1ra07238b |
|
This journal is © The Royal Society of Chemistry 2021 |