DOI:
10.1039/D1RA07203J
(Paper)
RSC Adv., 2021,
11, 37254-37267
Highly efficient visible light active Cu–ZnO/S-g-C3N4 nanocomposites for efficient photocatalytic degradation of organic pollutants†
Received
27th September 2021
, Accepted 29th October 2021
First published on 19th November 2021
Abstract
The photocatalytic activity of photocatalysts is severely hampered by limited visible light harvesting and unwanted fast recombination of photogenerated e− and h+. In the current study, the photocatalytic efficiency of Cu–ZnO/S-g-C3N4 (CZS) nanocomposites was investigated against MB dye. The composite materials were designed via chemical co-precipitation method and characterised by important analytical techniques. Distinctive heterojunctions developed between S-g-C3N4 and Cu–ZnO in the CZS composite were revealed by TEM. The synthesized composites exhibit a huge number of active sites, a large surface area, a smaller size and better visible light absorption. The considerable enhancement in the photocatalytic activity of CZS nanocomposites might be accredited to the decay in the e–h pair recombination rate and a red shift in the visible region, as observed by PL and optical analysis, respectively. Furthermore, the metal (Cu) doping into the S-g-C3N4/ZnO matrix created exemplary interfaces between ZnO and S-g-C3N4, and maximized the photocatalytic activity of CZS nanocomposites. In particular, CZS nanocomposites synthesized by integrating 25% S-g-C3N4 with 4% Cu–ZnO (CZS-25 NCs) exhibited the 100% photocatalytic degradation of MB in 60 minutes under sunlight irradiation. After six cycles, the photocatalytic stability of CZS-25 NCs was excellent. Likewise, a plausible MB degradation mechanism is proposed over CZS-25 NCs based on photoluminescence and reactive species scavenger test observation. The current research supports the design of novel composites for the photocatalytic disintegration of organic contaminants.
1. Introduction
Waste industrialization and subsequent pollution are certainly among the significant problems the world faces presently. Human activities have evolved different substances such as metal ions, organic pollutants, and pesticides, becoming a major threat for living beings. These organic pollutants need to be degraded to lower their impact on the environment because they tend to be toxic.1–3 Primarily, water pollution demands focus urgently. Semiconductor photocatalysis can be an excellent solution to the severe problem of pollution.4–7 To date, numerous metal oxides, such as ZnO, WO3, In2O3, SnO2, Cu2O and TiO2, have been used for the photocatalytic degradation of contaminants.8–10 Because of its low cost, outstanding stability, high yield, and good optical properties, ZnO is a potential photocatalyst.11,12 Unfortunately, the light absorption of ZnO is mainly limited to the UV region, and it has almost no visible light response due to its large bandgap (∼3.37 eV).13,14 Moreover, ZnO has some issues, such as low charge separation and photocorrosion. These problems have hindered the practical applications of ZnO in photocatalysis.15 Different techniques, including doping with ions and composite formation with semiconductors, were employed to overcome the inherent deficiencies of ZnO.16,17 Metal ion-doped ZnO has improved visible light absorption and photocatalytic activity.18–20 Various transition elements, such as Ni, Fe, Cr, Mn, and Cu, have been used to elevate the photocatalytic efficiency of ZnO.21 Cu is thought to be the most effective metal ion dopant for improving the photocatalytic competence of ZnO. Cu-doping produces defects in the ZnO lattice, and improves the optical and electrical properties. The Cu-doped ZnO with corn seed morphology exhibited 3.5 times better methyl orange photocatalytic degradation than pure ZnO.22 Vaiano et al. reported the complete oxidation of As(III) to As(V) within 120 minutes by Cu-doped ZnO nanoparticles under sunlight.23 The photocatalytic efficiency of Cu–ZnO/TiO2 was assessed against MO dye under sunlight, and 3% Cu–ZnO/TiO2 NPs exhibited approximately 28.3 and 12 times better catalytic efficiency than TiO2 and ZnO/TiO2, respectively.24 Researchers found that oxygen vacancies and carbonaceous species increased the photocatalytic activity of ZnO. Oxygen vacancies can reduce the bandgap energy of ZnO by forming shallow levels of oxygen vacancies that partially overlapped the ZnO valence band. Furthermore, the creation of additional levels below the conduction band (CB) of ZnO can narrow the band gap.25 Carbon species, including multi-wall carbon nanotube, g-C3N4 and graphenes, when incorporated with ZnO, acted as both photo-sensitizers and stabilisers.
It is widely known that modifying ZnO with a suitable semiconductor, such as g-C3N4, can effectively inhibit the electron–hole recombination and improve the photocatalytic activity. g-C3N4, as a polymeric semiconductor, has remarkable stability, good mechanical, thermal, optical, and electrical properties, and cost-effectiveness.26 It has proper absorption in the visible region (up to 450 nm), and an appropriate bandgap (∼2.7 eV) that makes it a potential photocatalyst for water splitting and pollution degradation.27 Conversely, the pristine g-C3N4 has some intrinsic limitations, such as relatively lower quantum efficiency and inadequate visible light absorption.28 As a result, the photocatalytic effectiveness of g-C3N4 in its pure form is insufficient for practical use. To boost the photocatalytic activity, the bandgap of g-C3N4 can be lowered by doping with sulfur. The S-doping modifies the bandgap of g-C3N4 by stacking its 2p orbitals on the VB of bulk g-C3N4, and improves the e–h pairs' mobility and separation. Hong et al. detailed that a better photocatalytic H2 (30 times) was produced by mesoporous S-g-C3N4 than pristine g-C3N4.29 Wang et al. stated that S-g-C3N4 exhibited a nearly 1.38 times better photocatalytic CO2 reduction rate than pristine g-C3N4.30 In another study, porous S-g-C3N4 showed improved adsorption and photocatalytic degradation of rhodamine B dye compared to pure g-C3N4 under visible light.31 Theoretically and experimentally, it has been recognized that S-doping has revised the g-C3N4 structurally, reduced its Eg value, and improved its e–h pair separation efficiency.32 To boost the photocatalytic activity of ZnO/g-C3N4, we used the concept of the coordinated action of both non-metal (sulphur) doped g-C3N4 and metal (Cu) doped ZnO in the nanocomposites.
So, it looks vital to combine the S-g-C3N4 and Cu–ZnO NPs to produce a hybrid composite (S-g-C3N4/Cu–ZnO) with excellent catalytic properties. In the study, a series of S-g-C3N4/Cu–ZnO nanocomposites (NCs) was made by physical mixing of variable amounts of S-g-C3N4 with Cu–ZnO NPs, and the photocatalytic efficiency of the resultant ternary composites was investigated against the MB dye. The comparative photocatalytic MB degradation by S-g-C3N4/Cu–ZnO NCs indicates that the heterojunction with ratio (3
:
1 = S-g-C3N4
:
Cu–ZnO) exhibited a maximum of 97% degradation of dye in 60 minutes. Due to the hybridization of diverse materials, more and more active sites are produced, which increases the e–h pairs separation and catalytic efficiency of the composites.
2. Materials and methods
2.1 Materials
Analytical grade thiourea ((NH2)2CS, ≥99.5%), copper nitrate trihydrate (Cu(NO3)2·3H2O, 99%), methylene blue (C16H18N3SCl, ≥99.0%), zinc nitrate hexahydrate (Zn(NO3)2·6H2O, 99%), ammonia solution (25%), ammonium oxalate (C2H8N2O4, ≥99.0%), ethanol (C2H5OH), isopropanol (C3H8O, ≥99.9%), and benzoquinone (C6H4O2, 99.5%) were bought from Merck and consumed without any purification.
2.2 Synthesis of ZnO NPs
ZnO and Cu-doped ZnO nanoparticles (NPs) were prepared via the co-precipitation mode.33 For the synthesis of ZnO, sodium hydroxide solution (4 M) was added dropwise into 0.04 M solution of Zn (NO3)2·6H2O (2.97 g/250 ml) with constant stirring. At pH = 11, the white precipitates yielded were washed with ethanol/DI water, and then allowed to dry at room temperature. Finally, calcination of the precipitate was carried out in a muffle furnace at 450 °C for 3 h to achieve ZnO NPs (with heating rate: 5 °C min−1). Similarly, Cu–ZnO NPs with various loadings of Cu (1%, 2%, 3%, 4%, and 5%) were synthesized by following the same co-precipitation method.33 To fabricate 1% Cu–ZnO NPs, 0.029 grams of Cu(NO3)2·3H2O were dissolved in 80 ml of DI water by constant stirring and heating gently. Then, the Cu precursor solution was added to 0.04 M Zn (NO3)2·6H2O solution. Then, the Cu–ZnO white precipitates were obtained by adding 25% ammonia solution. The final 1% Cu–ZnO NPs were obtained by repeating the steps, as previously described for the synthesis of ZnO. In the same way, (2%, 3%, 4%, and 5%) Cu–ZnO NPs were prepared. The synthesis conditions of the Cu–ZnO NPs with compositions are tabulated in Table 1.
Table 1 Contents and synthesis conditions of Cu–ZnO NPs
S. no. |
Nanoparticles |
wt% ratio |
MB dye degradation (%) (after 105 min) |
Cu(NO3)2·3H2O |
Zn(NO3)2·6H2O |
1 |
ZnO |
0 |
100 |
53 |
2 |
1% Cu–ZnO |
1 |
99 |
55 |
3 |
2% Cu–ZnO |
2 |
98 |
63 |
4 |
3% Cu–ZnO |
3 |
97 |
76 |
5 |
4% Cu–ZnO |
4 |
96 |
82 |
6 |
5% Cu–ZnO |
5 |
95 |
61 |
2.3 Synthesis of sulphur-doped g-C3N4 (S-g-C3N4)
S-g-C3N4 was fabricated by heating thiourea at 3 °C min−1 to 550 °C for 5 hours in a muffle furnace (direct low-temperature thermal condensation).34 The S-g-C3N4 so-attained was in the form of a yellowish porous product (Fig. 1).
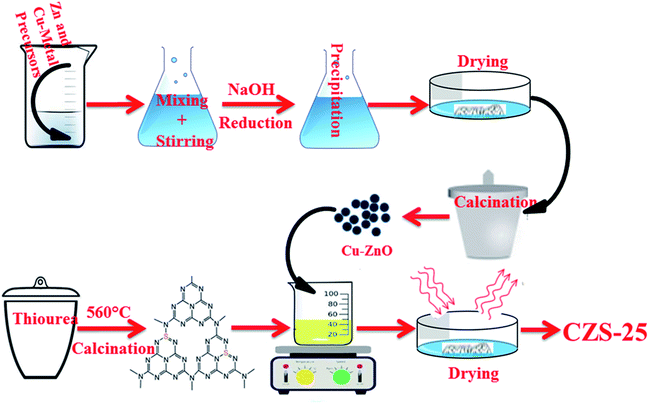 |
| Fig. 1 Diagrammatic illustration of the synthesis of 4% Cu–ZnO/S-g-C3N4 composites. | |
2.4 Synthesis of S-g-C3N4/Cu–ZnO nanocomposites
The maximum MB photodegradation was exhibited by the 4% Cu–ZnO NPs in the photocatalytic degradation experiment of MB by (0, 1, 2, 3, 4 & 5%) Cu–ZnO NPs. Then, the 4% Cu–ZnO NPs were mixed with diverse amounts of S-g-C3N4 to yield ternary nanocomposites (NCs). Then, the ternary composite with a (1
:
1) ratio was prepared by mixing and stirring 0.1 g of 4% Cu–ZnO NPs and 0.1 g of S-g-C3N4 in 50 ml of ethanol. The final (1
:
1) composite was obtained by evaporating ethanol in sunlight. By following the same process, the 4% Cu–ZnO/S-g-C3N4 composites in (1
:
3), (1
:
5), and (3
:
1) ratios were prepared. Similarly, the ZnO/S-g-C3N4 composite was synthesized for comparative photocatalytic study. The detailed composition and the resultant catalytic efficiency of the 4% Cu–ZnO/S-g-C3N4 NCs are given in Table 2.
Table 2 Composition of 4% Cu–ZnO/S-g-C3N4 nanocomposites and their corresponding photocatalytic activities
S. no. |
Nanocomposites |
wt% ratio of heterostructures |
Sample abbreviations |
S-g-C3N4 |
4% Cu@ZnO |
(S-g-C3N4 : Cu@ZnO) ratio |
1 |
4% Cu–ZnO/S-g-C3N4 |
50 |
50 |
(1 : 1) |
CZS-50 |
2 |
4% Cu–ZnO/S-g-C3N4 |
75 |
25 |
(3 : 1) |
CZS-75 |
3 |
4% Cu–ZnO/S-g-C3N4 |
83.33 |
16.67 |
(5 : 1) |
CZS-83 |
4 |
4% Cu–ZnO/S-g-C3N4 |
25 |
75 |
(1 : 3) |
CZS-25 |
5 |
S-g-C3N4 |
100 |
00 |
(1 : 0) |
SGN |
6 |
4% Cu–ZnO |
00 |
100 |
(0 : 1) |
4-CZN |
7 |
ZnO/S-g-C3N4 |
|
|
|
ZS |
2.5 Characterization
The XRD (Bruker AXS, D8-S4) approach was employed to identify the structure of the fabricated catalysts, and the morphology of the fabricated catalysts was detected by SEM (Hitachi, S-4800). The elemental composition was recognised with EDS. A UV-vis-NIR diffuse reflectance spectrophotometer was used to study the photocatalytic and UV-visible absorption spectra (UV-770, Jasco). Functional groups were measured using an FT-IR spectrometer (Perkin 400 FT-IR).
2.6 Photocatalytic activity
Photocatalytic study of the photocatalysts was accomplished under sunlight by observing the photocatalytic degradation of the MB dye. 0.05 g of each photocatalyst was added to 50 ml of dye solution (10 mg L−1) during the MB photocatalytic degradation experiment. Prior to conducting photocatalysis, each catalyst suspension was stirred for 0.5 hours to reach adsorption equilibrium between the catalyst and dye under dark conditions in a customized quartz reactor. Then, each sample was provided with sunlight to start the photocatalytic degradation of MB, and 5 ml aliquots were taken out after specific intervals. The dye catalysis was performed under solar radiation at ambient conditions (68–73 k lux). The taken aliquots were centrifuged (@9000 rpm) for 9 minutes to remove any suspended particles, and then scanned on an ultraviolet-visible spectrophotometer. Then, each photocatalyst's percent dye degradation (D%) was estimated by using the equation below: |
D% = ((Co − C)/Co) × 100
| (1) |
where Co and C are the initial and any time (t) concentrations of MB, respectively.
3. Results and discussions
3.1 Morphological and compositional analysis
Fig. 2a and b show the surface morphology of the synthesized ZnO and 4-CZN catalysts, as examined by the SEM technique. In the SEM image of ZnO, several nanosheets are arranged to build flower-like 3D shapes (Fig. 2a). In Fig. 2b, the stacked thin sheet morphology of the 4-CZN is exhibited. The SGN morphology consisted of typically curled two-dimensional sheets agglomerated to form bulk structures (Fig. 2c). The ternary CZS-75 photocatalyst displayed a porous, semi-round, sponge-like heterostructure with agglomeration (Fig. 2d). It is acclaimed that such heterojunction morphology increases the surface area, active sites, and corresponding photocatalytic activity of the material. Furthermore, the structural morphology of the catalysts was examined by TEM, as shown in Fig. 3a–d. The TEM images reveal the nanosized structures of ZnO and 4-CZN NPs, respectively, as shown in Fig. 3a and b. The ZnO and 4-CZN NPs show varying diameters (10–30 nm). The TEM images of ZS manifest the existing junction between the ZnO NPs and SGN sheets, as shown by Fig. 3c. The 4-CZN NPs are dispersed over the delicate sheets of SGN to design the CZS-25 heterostructure, as shown by Fig. 3d. The SGN soft sheets engross the 4-CZN NPs, which form the basis to reinforce the NPs and increase the photocatalytic efficiency of the resultant composite. The EDX analysis of the prepared materials was performed to identify their chemical elemental composition. Fig. 2e exhibits the EDX spectrum of ZnO NPs, which consists of only Zn and O elemental peaks. The EDX spectrum of the g-C3N4/Cu-doped ZnO composites contain C, N, S, Zn, O and Cu elemental peaks (Fig. 2f), and thus shows the purity of the synthesized composites.
 |
| Fig. 2 (a–d) SEM morphology of the ZnO (a), 4-CZN (b), SGN (c) and CZS-25 (d) composites, and EDS spectra of ZnO (e) and CZS-25 (f) samples. | |
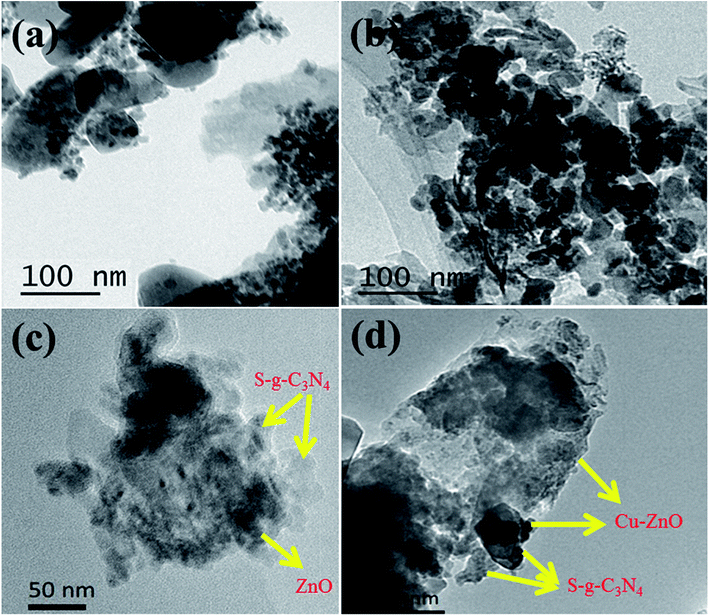 |
| Fig. 3 TEM images of (a) ZnO, (b) 4-CZN, (c) SGN and (d) CZS-25 composites. | |
3.2 XRD analysis
The purity and phase of the produced samples were investigated using XRD analysis. The XRD patterns of SGN, ZnO, 4-CZN, ZS, and CZS-25 are shown in Fig. 4a. The presence of the decisive peaks in XRD pattern of SGN at 27.4° corresponds to the crystal planes of (100) that endorse the existence of nanosheets in pristine SGN samples.35 The characteristic diffraction peaks for ZnO appeared at 2θ values of 32.06°, 35.0°, 36.98°, 48.01°, 57.03°, 63.05°, 69.0° and at 70.04°, which stand for the crystal planes of (100), (002), (101), (102), (110), (103), (112) and (201). The observed peaks are in complete consonance with the standard XRD pattern of the hexagonal wurtzite structure of ZnO (JCPDS 00-036-1451).36 The two-phase composition of the ZS and CZS-25 composites is endorsed by the co-existence of the characteristic diffraction peaks of 4-CZN and SGN in the corresponding XRD patterns.37 In the XRD patterns of the CZS-25 and 4-CZN samples, a pronounced reduction in intensity and slight shifting of the major peaks ((100), (101)) towards the lower angle is noticed, which verify the effective metal (Cu) ions doping into ZnO and incorporation of 4-CZN with SGN (Fig. 4b).38 In addition, a general broadening in the 4-CZN and SGN peaks and the shift towards the lower angle particular at (002) was noticeable mainly due to Cu doping.39
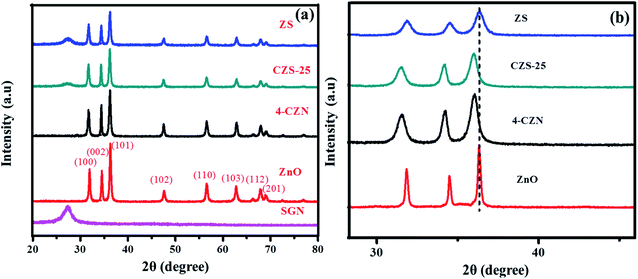 |
| Fig. 4 (a and b) XRD pattern of ZnO, 4-CZN, ZS, SGN and CZS-25 composites. | |
3.3 FTIR analysis
FTIR spectroscopy was used to characterize the functional groups and the chemical composition of the ZnO, 4-CZN, CZS-25, CZS-50, and CZS-75 samples (Fig. 5). The peaks occurring in ZnO NPs and 4-CZN, from 400 cm−1 to 500 cm−1, represent the metal oxide bond (Zn–O), while the peaks from 780 cm−1 to 980 cm−1 appear due to metal peroxides (M–O–O–M).40 A broad band at around 3350 cm−1 in the prepared samples is due to the stretching vibration of the –O–H bonds.41 In the CZS-25, CZS-50, and CZS-75 composites, several strong stretching bands are detected in the 1220–1630 cm−1 region, which is ascribed to the heptazine repeat units of g-C3N4.42 The peaks in composites at 807 cm−1 and about 3145 cm−1 are attributed to the N–H stretching frequency and the tri-s-triazine units, respectively.42,43 The presence of the corresponding peaks of g-C3N4 and ZnO in the CZS-25, CZS-50, and CZS-75 samples validated the purity and successful formation of the composites.44
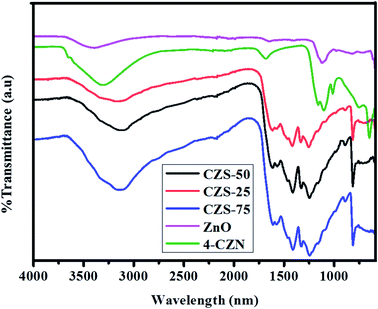 |
| Fig. 5 FTIR analysis of the ZnO, 4-CZN, CZS-25, CZS-50 and CZS-75 composites. | |
3.4 Optical properties
UV-visible diffuse reflectance spectroscopy was used to examine the optical properties of the produced catalysts (Fig. 6). The absorption band for pure ZnO was observed at 385 nm, indicating that ZnO NPs are unresponsive to visible radiation due to their high-energy bandgap. For 4-CZN NPs, there is a slight enhancement in visible light harvesting, which is congruent with their decreased bandgap energy (3.09 eV). In contrast to ZnO, SGN has an absorption edge at 460 nm. The absorption of the SGN exhibits an improvement because of sulfur doping into g-C3N4 that corresponds well with its observed bandgap energy of 2.60 eV and improved photocatalytic efficiency. Equally, the CZS-25 photocatalyst demonstrated outstanding visible-light absorption, inferring its enriched photocatalytic action under sunlight. Compared to pure ZnO and SGN, the ZS and CZS-25 NCs showed better absorption in the visible area (red-shift). Table 3 lists the bandgap energies of the fabricated materials calculated using Tauc's relation.45
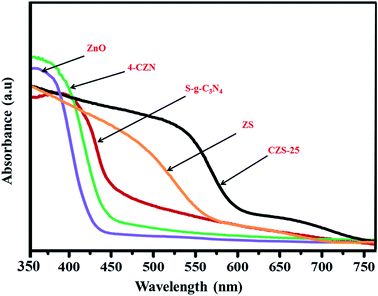 |
| Fig. 6 UV-vis DRS absorption spectra of the ZnO, 4-CZN, ZS and CZS-25 composites. | |
Table 3 Energy bandgap of ZnO, 4-CZN, S-g-C3N4, ZS and CZS-25
S. no. |
Sample |
Bandgap (eV) |
1 |
ZnO |
3.22 |
2 |
4-CZN |
3.09 |
3 |
S-g-C3N4 |
2.60 |
4 |
ZS |
2.87 |
5 |
CZS-25 |
2.54 |
3.5 PL analysis
Photoluminescence (PL) spectroscopy is a common method being used to probe the charge separation capacity of the photo-excited (e–h pairs) in the photocatalytic materials.46 The photoluminescence emission spectra of the produced catalysts observed at the excitation wavelength of 365 nm are shown in Fig. 7. The SGN has a prominent emission peak at 460 nm, indicating a high frequency of e–h pairs recombination. Compared with that of ZnO, the PL intensity of 4-CZN showed a significant drop, which can be attributed to the origin of certain trapped states and decreased density of intrinsic defects.47 In addition, compared with the bare SGN, there is a drop in the PL peak intensity of ZS, which is attributed to the formation of a ZS heterostructure, zinc defects and surface oxygen vacancies.48 The strong conjugation between the SGN sheets and 4-CZN NPs resulted in a low PL peak of the CZS-25 heterostructure. The interfacial transfer of charge (electrons) between ZnO and g-C3N4 might be responsible for the diminished PL peak and substantially decreased rate of e–h pairs recombination in the CZS-25 NCs. As cited in the previous literature, the doped transition metal assists the transfer of charge between ZnO and g-C3N4 in CZS NCs.49 Thus, in the CZS-25 nanocomposites, the photo-excited electrons were effectively conducted and used up in ROS production. Thus, the degree of e–h pairs recombination in CZ-25 is repressed, and its photocatalytic activity is improved.
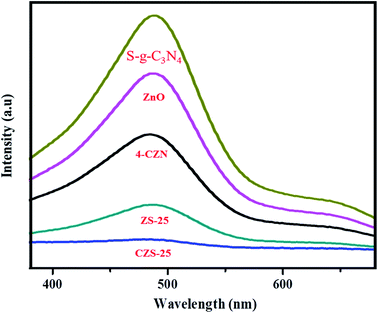 |
| Fig. 7 PL analysis of the ZnO, 4-CZN, ZS and CZS-25 composites. | |
3.6 Surface area analysis
The prepared catalytic materials' surface area and pore volumes were estimated using the BET method via the N2-adsorption technique. Table 4 lists the surface area of the materials calculated via the N2 adsorption–desorption technique. Compared to the other photocatalysts, the CZS-25 composite has the greatest surface area and a large pore volume. The Cu dopant inserted between the ZnO and SGN sheets might be responsible for the observed largest surface area of the CZS-25. Greater surface areas are thought to provide more active sites, allowing photocatalysts to absorb a greater number of contaminants on the surface. As a result, the CZS-25 may be likely to absorb and decompose the greatest amount of contaminants compared to other samples.50,51
Table 4 Nitrogen physisorption data of ZnO, 4-CZN, S-g-C3N4, ZS-25 and CZS-25
Sample |
Surface area (m2 g−1) |
Average pore width (Å) |
Pore volume (cm3 g−1) |
ZnO |
6.33 |
138.12 |
0.04 |
4-CZN |
15.39 |
1141.22 |
0.06 |
ZS |
26.24 |
170.10 |
0.09 |
CZS-25 |
63.44 |
84.59 |
0.12 |
3.7 Photoactivity
Fig. 8a and b shows the photocatalytic activity of ZnO and Cu-doped ZnO NPs (1–5%) against MB under sunlight. The sample-dye solutions were kept under dark for 1 hour before the photocatalysis to attain an adsorption–desorption equilibrium. The photocatalytic degradation rate of the MB dye and absorption graph of samples is shown in Fig. 8b. Maximal adsorption was achieved by 4-CZN NPs (16.7%), as compared to other samples (Fig. 8b).
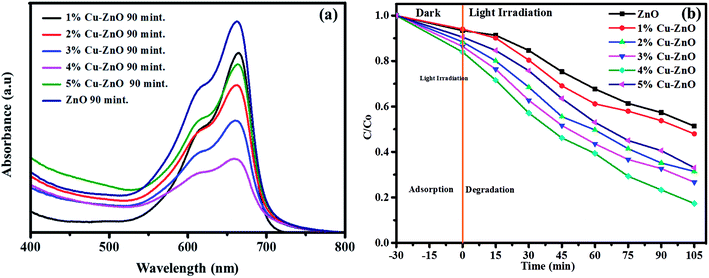 |
| Fig. 8 Photocatalytic efficiency (a) and % degradation of MB (b) by ZnO and (1, 2, 3, 4, and 5 wt%) Cu–ZnO nanoparticles. | |
The photocatalytic degradation of the MB solution was initiated by irradiating the sample-dye solutions, and Fig. 8a depicts the corresponding dye degradation behaviour of the photocatalysts. From the spectral graph, there is a gradual decline in dye degradation with time for all of the samples. 4-CZN NPs exhibit the maximum photocatalytic degradation of MB (82%) after 105 minutes (Fig. 8b), and this better photocatalytic activity of 4-CZN might happen due to the formation of additional trapping sites in 4-CZN due to optimum Cu doping into ZnO. These trapping sites (defects) minimize the e–h pair recombination by improving the induced charge carriers' lifetime and improving the dye degradation rate.52
As the photocatalytic efficiency of 4-CZN NPs against MB is not ideal, these NPs were coupled with variable concentrations of SGN to form nanocomposites (4% Cu–ZnO/SGN) with better photocatalytic efficiency. The detailed composition of the resultant NCs and their abbreviations are given in Table 2. Fig. 9a reveals the photocatalytic activity of synthesized materials (SGN, 4-CZN, ZS, CZS-25, CZS-50, and CZS-75) against MB under sunlight. Before sunlight exposure, an adsorption–desorption equilibrium was achieved to explore the adsorption aptitudes of the samples by keeping each under dark for 60 minutes. Variable concentrations of MB were adsorbed by the fabricated materials, as given in Fig. 9a. The CZS-25 NC exhibited the maximum adsorption, as compared to other samples, because of its higher surface area and maximum pore size (Table 4).53
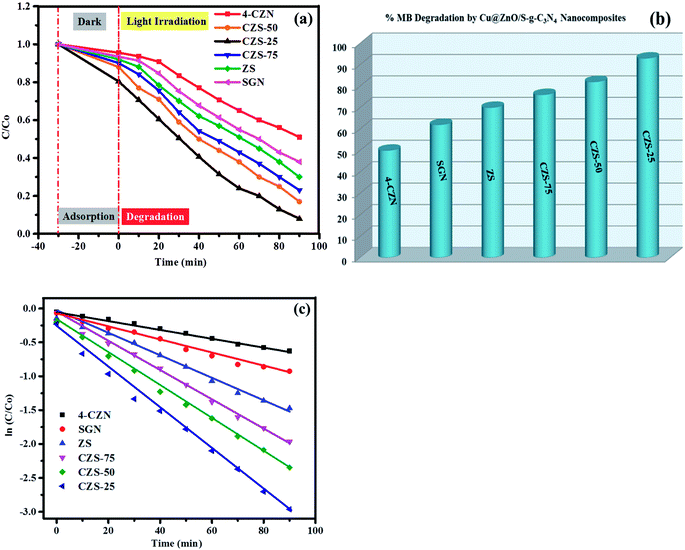 |
| Fig. 9 Degradation rate (a), % degradation (b), and kinetic characteristics of MB under sunlight (c) by 4-CZN, ZS, SGN, CZS-25, CZS-50 and CZS-75 composites. | |
Remarkably, the 4% Cu–ZnO/S-g-C3N4 sample revealed an improved photocatalytic degradation of MB compared to the SGN, 4-CZN, and ZS samples. The dye degradation rate for CZS-25 NC approaches 78% as compared to CZS-50 NC (62%) and CZS-75 NC (58%) after 60 minutes of sunlight illumination (Fig. 9a). Furthermore, the dye degradation rate of CZS-25 NC reached up to 93% after 90 minutes, as given by the bar graph in Fig. 9c. The enhanced dye degradation by the CZS-25 NC might be ascribed to the better charge separation. The fast charge transfer and enriched visible light absorption by CZS-25 NC are ascribed to the coupling of Cu–ZnO with SGN.46,47 The electronic and optical properties of ZnO and SGN are significantly improved by Cu ion doping.54,55 It might be the reason for the maximum photocatalytic efficiency of CZS-25 NC compared to the other synthesized composites.
From Fig. 9c, it is evident that the kinetics of the photocatalytic degradation of MB by NCs is pseudo-first-order. The value of k was calculated from the plot ln(C/Co) vs. time t, as shown in Fig. 9c. The largest rate constant, 0.0334 min−1, is found for CZS-25 NC because it decolorized the MB in 90 minutes. The “k” value of the ternary CZS-25 NC for MB degradation is almost 3 times greater than the “k” value of SGN (0.011 min−1) and 5.5 times more than that of 4-CZN (0.006 min−1). The order of the k values for the fabricated materials is as follows: CZS-25 NC (0.0334 min−1) > CZS-50 NC (0.0210 min−1) > CZS-75 NC (0.0171 min−1) > ZS NC (0.0134 min−1) > SGN (0.011 min−1) > 4-CZN (0.006 min−1). The maximum rate constant value observed for CZS-25 NC indicated that the synergistic effect between SGN, Cu, and ZnO has improved the number of active sites, and correspondingly enhanced the photocatalytic efficiency against MB dye.56–58 Nonetheless, as the quantity of S-g-C3N4 in CZS NCs was increased beyond 25%, the photocatalytic performance of CZS NCs consistently decreased. This effect is consistent with previous findings, which show that as the constituent content surpasses the optimum value, the photocatalytic efficacy of the type-II nanoheterostructures decreases.59–62
The previous literature revealed that the photocatalytic efficiency of Cu-doped g-C3N4 and Cu-doped ZnO had been enhanced due to their improved charge carrier transportation, reduced particle size, and modified energy structure.58,63 In addition, the photocatalytic efficiency of the CZS-25 NC is practically higher compared to the previously reported studies, as presented in Table 5.64–70 Hence, the CZS-25 NC is the most effective composite for the photocatalytic dye degradation and was further employed for the recycling study.
Table 5 Photocatalytic efficiency of 4% Cu–ZnO/S-g-C3N4 and some previous photocatalysts
S. no. |
Composite |
Organic pollutant |
Light source |
Irradiation time (min) |
Percent degradation |
Ref. |
1 |
Cu doped ZnO/Cu/g-C3N4 |
MB |
Visible |
60 |
98 |
64 |
2 |
Mn–ZnO/RGO |
RhB |
Visible |
140 |
99 |
65 |
3 |
Ag-doped g-C3N4 |
MB |
Xe lamp |
120 |
96 |
66 |
4 |
ZnO/Fe3O4/g-C3N4 |
MO |
Visible |
150 |
97.87 |
67 |
5 |
Cu–TiO2 |
MB |
Xe lamp |
120 |
99 |
68 |
6 |
g-C3N4@Ag2ZrO3 |
RhB |
Visible |
120 |
99.70 |
69 |
7 |
Mn–ZnO/CSAC |
BG |
Solar |
120 |
97.47 |
70 |
8 |
CZS-25 |
MB |
Solar |
90 |
93 |
Current study |
3.8 Reusability and stability of the CZS-25 composite
As the durability of the photocatalyst under repetitive photocatalytic processes is essential for practical applications, the stability of the CZS-25 NCs was thus studied by MB dye degradation experiment up to six runs. As given in Fig. 10a, the CZS-25 NCs kept up the dye degradation rate, and no marked decline in catalytic efficiency was seen even after the twelfth consecutive cycle. Additionally, no marked change in XRD results of the CZS-25 NCs was detected after the twelfth cycle (Fig. 10b). Hence, the CZS-25 photocatalyst can be taken as a stable photocatalyst to be applied under sunlight.
 |
| Fig. 10 The stability (a) XRD analysis (b) of CZS-25 after the 6th cycle. | |
3.9 Radical trapping experiments
To investigate the feasible dye degradation mechanism by the CZS-25 NC, it is critical to recognize the leading ROS for the photocatalytic degradation of MB. The photogenerated ROS produced during the dye degradation was captured by applying the important scavengers. The observed scavenger effect on the photocatalytic degradation of MB has been tabularized in Table 6 and given by Fig. 11.
Table 6 Dye degradation inhibition of 4% Cu–ZnO/S-g-C3N4 by employed scavengers
Trapping agents |
Captured species |
% inhibition |
Ammonium oxalate |
h+ |
17 |
Benzoquinone |
˙O2− |
95 |
Isopropanol |
˙OH |
65 |
No trapping agent |
No capturing |
00 |
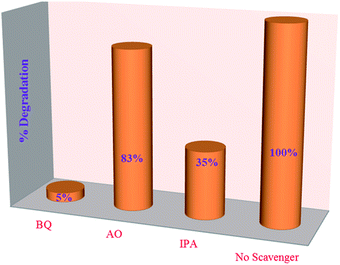 |
| Fig. 11 Effect of scavengers on the photocatalytic degradation of MB by the 4% Cu–ZnO/S-g-C3N4 composite. | |
These observations validate that the primary species involved in the photocatalytic degradation of MB are ˙O2− and ˙OH. Simultaneously, the holes with a 17% inhibition effect are the least effective active species for catalytic dye degradation.
3.10 Mechanism
The proposed photocatalytic MB degradation mechanism over the CZS-25 photocatalyst under sunlight radiation is depicted in Fig. 12. When CZS-25 NC is irradiated, e–h pairs are produced by sulphurized g-C3N4 and Cu-doped ZnO. The electrons from the valence band (VB) of ZnO and SGN are excited to the corresponding conduction band (CB). Then, these electrons from the CB of SGN are shifted to the CB of ZnO, owing to the lower CB edge potential (−0.5 eV) of ZnO than CB potential (−1.12 eV) of g-C3N4.71–74 The doped Cu atoms in the CZS-25 NC at the SGN and ZnO interfaces function as mediators to transfer electrons from SGN to ZnO, and decrease the recombination frequency of electrons and holes. The electrons at the CB of ZnO are consumed in the induction of superoxide radicals (O2−) by reacting with chemisorbed O2 on the surface of CZS-25 NC. Meanwhile, the h+ in the VB of ZnO are migrated to the VB of g-C3N4 due to the lower VB potential of ZnO than the VB potential of g-C3N4.75,76 The trapped holes on g-C3N4 yield hydroxyl radicals (OH˙) by reacting with H2O.76,77 In addition, the accumulated h+ in the VB of g-C3N4 might directly degrade MB.78–82 The produced (O2− and OH˙) reactive species interact with the MB molecules and decompose them by following a sequence of redox reactions, as given by eqn (2)–(6). The better photocatalytic activity of the CZS-25 NC might be ascribed to the suppressed e–h pairs recombination rate because of the synergetic effect between g-C3N4 and Cu-doped ZnO, and due to the formation of sufficient interfaces between these two phases. |
S-g-C3N4/Cu-doped ZnO + hυ → g-C3N4/Cu-doped ZnO (e−/h+)
| (2) |
|
Cu-doped ZnO (e−) + O2 → ˙O2−
| (3) |
|
S-g-C3N4 + H2O/OH− → ˙OH
| (4) |
|
˙OH/˙O2− + MB → degraded products
| (5) |
|
h+ + MB → degraded products
| (6) |
 |
| Fig. 12 A schematic MB photocatalytic degradation mechanism over the Cu@ZnO/S-g-C3N4 composite under sunlight. | |
4. Conclusions
In conclusion, we successfully synthesized highly efficient Cu@ZnO/S-g-C3N4 photocatalysts via the chemical co-precipitation method. The characterization of the photocatalysts was done by TEM, XRD, EDX, FTIR, SEM and UV-visible, and PL spectroscopy. The comparative photocatalytic MB degradation revealed the excellent photocatalytic efficiency of CZS-25 NC compared to SGN, 4-CZN, ZS, CZS-50, and CZS-75 samples, and it degraded 100% of MB in 60 minutes under sunlight illumination. The well-developed interfaces between Cu–ZnO NPs and delicate sheets of SGN in the CZS-25 NC as depicted by morphological characterization favoured the effective charge conduction and harvesting of visible radiations. The improved photocatalytic activity of CZS-25 NC might be attributed to effective e–h pair separation due to the establishment of a well-defined heterojunction among the ZnO, SGN, and Cu ions. Overall, the present research outcomes provide us with new insight into the synthesis of photocatalytic materials and their applications for organic pollution degradation from wastewater.
Conflicts of interest
The authors hereby declare that there is no conflict of interest and satisfy all ethics in publishing policy.
Acknowledgements
The authors gratefully acknowledge the support received for this research work from the HEC start up grant (SRGP Project No. 2272) and Department of Chemistry, University of Management & Technology, Lahore-54770, Pakistan.
References
- X. F. Liang, G. L. Wang, X. L. Dong, G. W. Wang, H. C. Ma and X. F. Zhang, Graphitic carbon nitride with carbon vacancies for photocatalytic degradation of bisphenol A, ACS Appl. Nano Mater., 2019, 2, 517–524 CrossRef CAS.
- X. B. Feng, H. M. Ju, T. H. Song, T. S. Fang, W. C. Liu and W. Huang, Highly efficient photocatalytic degradation performance of CsPb(Br1-xClx)3-Au nanoheterostructures, ACS Sustainable Chem. Eng., 2019, 7, 5152–5156 CrossRef CAS.
- R. C. Ding, Y. Z. Fan and G. S. Wang, High efficient Cu2O/TiO2 nanocomposite Photocatalyst todegrade organic Polluant under visible light irradiation, ChemistrySelect, 2018, 3, 1682–1687 CrossRef CAS.
- J. N. Schrauben, R. Hayoun, C. N. Valdez, M. Braten, L. Fridley and J. M. Mayer, Titanium and zinc oxide nanoparticles are proton-coupled electron transfer agents, Science, 2012, 336, 1298–1301 CrossRef CAS PubMed.
- D. Vidyasagar, S. G. Ghugal, A. Kulkarni, A. G. Shende, S. S. Umare and R. Sasikala, Microwave assisted: in situ decoration of a g-C3N4 surface with CdCO3 nanoparticles for visible light driven photocatalysis, New J. Chem., 2018, 42, 6322–6331 RSC.
- G. Mamba and A. K. Mishra, Graphitic carbon nitride (g-C3N4) nanocomposites: a new and exciting generation of visible light driven photocatalysts for environmental pollution remediation, Appl. Catal., B, 2016, 198, 347–377 CrossRef CAS.
- K. Ravichandran and E. Sindhuja, Fabrication of cost effective g-C3N4+Ag activated ZnO photocatalyst in thin film form for enhanced visible light responsive dye degradation, Mater. Chem. Phys., 2019, 221, 203–215 CrossRef CAS.
- J. Šíma and P. Hasal, Photocatalytic degradation of textile dyes in a TiO2/UV system, Chem. Eng. J., 2013, 32, 79–84 Search PubMed.
- M. Mousavi, A. Habibi-Yangjeh and S. R. Pouran, Review on the criteria anticipated for the fabrication of highly efficient ZnO-based visible-light-driven photocatalysts, J. Ind. Eng. Chem., 2018, 62, 1–25 CrossRef.
- S. Iqbal, Spatial charge separation and transfer in L-cysteine capped NiCoP/CdS nano-heterojunction activated with intimate covalent bonding for high-quantum-yield photocatalytic hydrogen evolution, Appl. Catal., 2020, 274, 119097 CrossRef CAS.
- K. S. Siddiqi, A. Ur Rahman and A. T. Husen, Properties of zinc oxide nanoparticles and their activity against microbes, Nanoscale Res. Lett., 2018, 13, 141 CrossRef PubMed.
- M. Shekofteh-Gohari, A. Habibi-Yangjeh, M. Abitorabi and A. Rouhi, Magnetically separable nanocomposites based on ZnO and their applications in photocatalytic processes: a review, Crit. Rev. Environ. Sci. Technol., 2018, 48, 806–857 CrossRef CAS.
- S. Liang, K. Xiao, Y. Mo and X. Huang, A novel ZnO nanoparticle blended poly-vinylidene fluoride membrane for anti-irreversible fouling, J. Membr. Sci., 2012, 394, 184–192 CrossRef.
- I. Djerdj, Z. Jagličić, D. Arčon and M. Niederberger, Co-doped ZnO nanoparticles: minireview, Nanoscale, 2010, 2, 1096–1104 RSC.
- O. Długosz, K. Szostak and M. Banach, Photocatalytic properties of zirconium oxide-zinc oxide nanoparticles synthesised using microwave irradiation, Appl. Nanosci., 2020, 10, 941–954 CrossRef.
- A. M. Tama, S. Das, S. Dutta, M. D. I. Bhuyan, M. N. Islam and M. A. Basith, MoS2 nanosheet incorporated α-Fe2O3/ZnO nanocomposite with enhanced photocatalytic dye degradation and hydrogen production ability, RSC Adv., 2019, 9, 40357–40367 RSC.
- S. Iqbal, M. Javed, A. Bahadur, M. A. Qamar, M. Ahmad, M. Shoaib, M. Raheel, N. Ahmad, M. B. Akbar and H. Li, Controlled synthesis of Ag-doped CuO nanoparticles as a core with poly(acrylic acid) microgel shell for efficient removal of methylene blue under visible light, J. Mater. Sci.: Mater. Electron., 2020, 31, 8423–8435 CrossRef CAS.
- V. Vaiano, G. Iervolino and L. Rizzo, Cu-doped ZnO as efficient photocatalyst for the oxidation of arsenite to arsenate under visible light, Appl. Catal., B, 2018, 238, 471–479 CrossRef CAS.
- T. Welderfael, O. P. Yadav, A. M. Taddesse and J. Kaushal, Synthesis, characterization and photocatalytic activities of Ag-N-codoped ZnO nanoparticles for degradation of methyl red, Bull. Chem. Soc. Ethiop., 2013, 27, 221–232 CAS.
- Y. Lv, J. Lin, S. Peng, L. Zhang and L. Yu, Effective ways to enhance the photocatalytic activity of ZnO nanopowders: high crystalline degree, more oxygen vacancies, and preferential growth, New J. Chem., 2019, 43, 19223–19231 RSC.
- S. Kuriakose, B. Satpati and S. Mohapatra, Highly efficient photocatalytic degradation of organic dyes by Cu doped ZnO nanostructures, Phys. Chem. Chem. Phys., 2015, 17, 25172–25181 RSC.
- A. N. Kadam, T. G. Kim, D. S. Shin, K. M. Garadkar and J. Park, Morphological evolution of Cu doped ZnO for enhancement of photocatalytic activity, J. Alloys Compd., 2017, 710, 102–113 CrossRef CAS.
- V. Vaiano, G. Iervolino and L. Rizzo, Cu-doped ZnO as efficient photocatalyst for the oxidation of arsenite to arsenate under visible light, Appl. Catal., B, 2018, 238, 471–479 CrossRef CAS.
- M. R. D. Khaki, M. S. Shafeeyan, A. A. A. Raman and W. M. A. W. Daud, Enhanced UV–Visible photocatalytic activity of Cu-doped ZnO/TiO2 nanoparticles, J. Mater. Sci.: Mater. Electron., 2018, 29, 5480–5495 CrossRef.
- M. Samadi, H. A. Shivaee, A. Pourjavadi and A. Z. Moshfegh, Synergism of oxygen vacancy and carbonaceous species on enhanced photocatalytic activity of electrospun ZnO-carbon nanofibers: charge carrier scavengers mechanism, Appl. Catal., A, 2013, 466, 153–160 CrossRef CAS.
- S. Zhang, C. Su, H. Ren, M. Li, L. Zhu, S. Ge, M. Wang, Z. Zhang, L. Li and X. Cao, In situ fabrication of ZnO/g-C3N4 nanocomposites for photocatalytic degradation of methylene blue: synthesis procedure does matter, Nanomaterials, 2019, 9, 215 CrossRef CAS PubMed.
- R. Kuriki, K. Sekizawa, O. Ishitani and K. Maeda, Visible-light-driven CO 2 reduction with carbon nitride: enhancing the activity of ruthenium catalysts, Angew. Chem., Int. Ed., 2015, 54, 2406–2409 CrossRef CAS PubMed.
- J. Wang, Z. Yang, X. Gao, W. Yao, W. Wei, X. Chen and Y. Zhu, Core-shell g-C3N4@ZnO composites as photoanodes with double synergistic effects for enhanced visible-light photoelectrocatalytic activities, Appl. Catal., B, 2017, 217, 169–180 CrossRef CAS.
- J. Hong, X. Xia, Y. Wang and R. Xu, Mesoporous carbon nitride with in situ sulfur doping for enhanced photocatalytic hydrogen evolution from water under visible light, J. Mater. Chem., 2012, 22, 15006–15012 RSC.
- K. Wang, Q. Li, B. Liu, B. Cheng, W. Ho and J. Yu, Sulfur-doped g-C3N4 with enhanced photocatalytic CO2-reduction performance, Appl. Catal., B, 2015, 176–177, 44–52 CAS.
- Q. Fan, J. Liu, Y. Yu, S. Zuo and B. Li, A simple fabrication for sulfur doped graphitic carbon nitride porous rods with excellent photocatalytic activity degrading RhB dye, Appl. Surf. Sci., 2017, 391, 360–368 CrossRef CAS.
- L. Jiang, X. Yuan, Y. Pan, J. Liang, G. Zeng, Z. Wu and H. Wang, Doping of graphitic carbon nitride for photocatalysis: a review, Appl. Catal., B, 2017, 217, 388–406 CrossRef CAS.
- M. Sher, M. Javed, S. Shahid, S. Iqbal, M. A. Qamar, A. Bahadur and M. A. Qayyum, The controlled synthesis of g-C3N4/Cd-doped ZnO nanocomposites as potential photocatalysts for the disinfection and degradation of organic pollutants under visible light irradiation, RSC Adv., 2020, 11, 2025–2039 RSC.
- K. Wang, Q. Li, B. Liu, B. Cheng, W. Ho and J. Yu, Sulfur-doped g-C3N4 with enhanced photocatalytic CO2-reduction performance, Appl. Catal., B, 2015, 176–177, 44–52 CAS.
- X. Yang, Z. Tian, Y. Chen, H. Huang, J. Hu and B. Wen, In situ synthesis of 2D ultrathin cobalt doped g-C3N4 nanosheets enhances photocatalytic performance by accelerating charge transfer, J. Alloys Compd., 2021, 859, 157754 CrossRef CAS.
- X. Wu, Z. Wei, L. Zhang, X. Wang, H. Yang and J. Jiang, Optical and magnetic properties of Fe doped ZnO nanoparticles obtained by hydrothermal synthesis, J. Nanomater., 2014, 2014, 4 Search PubMed.
- Y. P. Zhu, M. Li, Y. L. Liu, T. Z. Ren and Z. Y. Yuan, Carbon-doped ZnO hybridized homogeneously with graphitic carbon nitride nanocomposites for photocatalysis, J. Phys. Chem. C, 2014, 118, 10963–10971 CrossRef CAS.
- M. F. Manzoor, E. Ahmad, M. Ullah, A. M. Rana, A. S. Malik, M. Farooq, I. Ahmad, M. Hasnain, Z. A. Shah, W. Q. Khan and U. Mehtab, Impact of Copper Doping on the Structural, Electrical and Optical Properties of Auto-Combustion Synthesized ZnO Nanocomposites, Acta Phys. Pol., A, 2019, 135, 458–466 CrossRef CAS.
- Y. H. Lu, W. H. Lin, C. Y. Yang, Y. H. Chiu, Y. C. Pu, M. H. Lee, Y. C. Tseng and Y. J. Hsu, A facile green antisolvent approach to Cu2+-doped ZnO nanocrystals with visible-light-responsive photoactivities, Nanoscale, 2014, 6, 8796–8803 RSC.
- S. C. Yan, Z. S. Li and Z. G. Zou, Photodegradation performance of g-C3N4 fabricated by directly heating melamine, Langmuir, 2009, 25, 10397–10401 CrossRef CAS PubMed.
- F. Guo, W. Shi, W. Guan, H. Huang and Y. Liu, Carbon dots/g-C3N4/ZnO nanocomposite as efficient visible-light driven photocatalyst for tetracycline total degradation, Sep. Purif. Technol., 2017, 173, 295–303 CrossRef CAS.
- S. P. Adhikari, H. R. Pant, H. J. Kim, C. H. Park and C. S. Kim, One pot synthesis and characterization of Ag-ZnO/g-C3N4 photocatalyst with improved photoactivity and antibacterial properties, Colloids Surf., A, 2015, 482, 477–484 CrossRef CAS.
- G. Di, Z. Zhu, H. Zhang, J. Zhu, Y. Qiu, D. Yin and S. Küppers, Visible-light degradation of sulfonamides by Z-scheme ZnO/g-C3N4 heterojunctions with amorphous Fe2O3 as electron mediator, J. Colloid Interface Sci., 2019, 538, 256–266 CrossRef CAS PubMed.
- N. Kumaresan, M. M. A. Sinthiya, M. Sarathbavan, K. Ramamurthi, K. Sethuraman and R. R. Babu, Synergetic effect of g-C3N4/ZnO binary nanocomposites heterojunction on improving charge carrier separation through 2D/1D nanostructures for effective photocatalytic activity under the sunlight irradiation, Sep. Purif. Technol., 2019, 116356 Search PubMed.
- D. Jiang, L. Chen, J. Xie and M. Chen, Ag2S/g-C3N4 composite photocatalysts for efficient Pt-free hydrogen production. The co-catalyst functions of Ag/Ag2S formed by simultaneous photodeposition, Dalton Trans., 2014, 43, 4878–4885 RSC.
- N. Kumaresan, M. M. Sinthiya, M. P. Kumar, S. Ravichandran, R. R. Babu, K. Sethurman and K. Ramamurthi, Investigation on the g-C3N4 encapsulated ZnO nanorods heterojunction coupled with GO for effective photocatalytic activity under visible light irradiation, Arabian J. Chem., 2020, 13, 2826–2843 CrossRef CAS.
- L. N. Tong, T. Cheng, H. B. Han, J. L. Hu, X. M. He, Y. Tong and C. M. Schneider, Photoluminescence studies on structural defects and room temperature ferromagnetism in Ni and Ni–H doped ZnO nanoparticles, J. Appl. Phys., 2010, 108, 023906 CrossRef.
- D. Theyvaraju and S. Muthukumaran, Microstructure, crystallographic and photoluminescence examination of Ni doped ZnO nanoparticles Co-doped with Co by sol–gel method, J. Inorg. Organomet. Polym. Mater., 2017, 27, 1572–1582 CrossRef CAS.
- Y. A. Chen, Y. T. Wang, H. S. Moon, K. Yong and Y. J. Hsu, Yolk-shell nanostructures: synthesis, photocatalysis and interfacial charge dynamics, RSC Adv., 2021, 20, 12288–12305 RSC.
- A. J. Reddy, M. K. Kokila, H. Nagabhushana, R. P. S. Chakradhar, C. Shivakumara, J. L. Rao and B. M. Nagabhushana, J. Alloys Compd., 2011, 509, 5349–5355 CrossRef CAS.
- M. A. Mohamed, M. F. M. Zain, L. J. Minggu, M. B. Kassim, J. Jaafar, N. A. S. Amin, M. S. Mastuli, H. Wu, R. J. Wong and Y. H. Ng, Bio-inspired hierarchical hetero-architectures of in situ C-doped g-C3N4 grafted on C, N co-doped ZnO micro-flowers with booming solar photocatalytic activity, J. Ind. Eng. Chem., 2019, 77(25), 393–407 CrossRef CAS.
- D. Neena, M. Humayun, D. Bhattacharyya and D. Fu, Hierarchical Sr-ZnO/g-C3N4 heterojunction with enhanced photocatalytic activities, J. Photochem. Photobiol., A, 2020, 112515 Search PubMed.
- Y. Matsukawa, S. Hirata, M. Inada, N. Enomoto, J. Hojo and K. Hayashi, Kinetic effects of polymorphs and surface areas on adsorption and photocatalytic decomposition of acetaldehyde on titania, Chem. Eng. J., 2020, 125422 CrossRef CAS.
- R. S. Das, S. K. Warkhade, A. Kumar, G. S. Gaikwad and A. V. Wankhade, Graphitic carbon nitride@silver zirconate nanocomposite (g-C3N4@Ag2ZrO3): a type-II heterojunction for an effective visible light photocatalysis and bacterial photo-inactivation, J. Alloys Compd., 2020, 155770 CrossRef CAS.
- M. Rashid, M. Ikram, A. Haider, S. Naz, J. Haider, A. Ul-Hamid, A. Shahzadi and M. Aqeel, Photocatalytic, dye degradation, and bactericidal behavior of Cu-doped ZnO nanorods and their molecular docking analysis, Dalton Trans., 2020, 49, 8314–8330 RSC.
- D. Toloman, A. Popa, M. Stan, M. Stefan, G. Vlad, S. Ulinici, G. Baisan, T. D. Silipas, S. Macavei, C. Leostean and S. Pruneanu, Visible-light-driven photocatalytic degradation of different organic pollutants using Cu doped ZnO-MWCNT nanocomposites, J. Alloys Compd., 2021, 866, 159010 CrossRef CAS.
- D. D. Qin, J. J. Quan, S. F. Duan, J. San Martin, Y. Lin, X. Zhu, X. Q. Yao, J. Z. Su, I. Rodríguez-Gutiérrez, C. L. Tao and Y. Yan, High-performance photoelectrochemical water oxidation with phosphorus-doped and metal phosphide co catalyst-modified g-C3N4 formation through gas treatment, ChemSusChem, 2019, 12, 898–907 CrossRef CAS PubMed.
- F. Guo, W. Shi, W. Guan, H. Huang and Y. Liu, Carbon dots/g-C3N4/ZnO nanocomposite as efficient visible-light driven photocatalyst for tetracycline total degradation, Sep. Purif. Technol., 2017, 173, 295–303 CrossRef CAS.
- Y. F. Lin and Y. J. Hsu, Interfacial charge carrier dynamics of type-II semiconductor nanoheterostructures, Appl. Catal., B, 2013, 130, 93–98 CrossRef.
- T. H. Lai, K. I. Katsumata and Y. J. Hsu, In situ charge carrier dynamics of semiconductor nanostructures for advanced photoelectrochemical and photocatalytic applications, Nanophotonics, 2021, 10, 777–795 CrossRef CAS.
- C. W. Tsao, M. J. Fang and Y. J. Hsu, Modulation of interfacial charge dynamics of semiconductor heterostructures for advanced photocatalytic applications, Coord. Chem. Rev., 2021, 438, 213876 CrossRef CAS.
- Y. H. Chiu, T. F. M. Chang, C. Y. Chen, M. Sone and Y. J. Hsu, Mechanistic insights into photodegradation of organic dyes using heterostructure photocatalysts, Catalysts, 2019, 9, 430 CrossRef CAS.
- D. Toloman, A. Popa, M. Stan, M. Stefan, G. Vlad, S. Ulinici, G. Baisan, T. D. Silipas, S. Macavei, C. Leostean and S. Pruneanu, Visible-light-driven photocatalytic degradation of different organic pollutants using Cu doped ZnO-MWCNT nanocomposites, J. Alloys Compd., 2021, 866, 159010 CrossRef CAS.
- M. A. Bajiri, A. Hezam, N. Keerthiraj, B. M. Al-Maswari, H. S. B. Naik, K. Byrappa and R. AlasmariNon-Noble, Metallic Cu with Three Different Roles in Cu doped ZnO/Cu/g-C3N4 Heterostructure for Enhanced Z-Scheme Photocatalytic Activity, New J. Chem., 2021, 45, 13499–13511 RSC.
- P. K. Labhane, L. B. Patle, G. H. Sonawane and S. H. Sonawane, Fabrication of ternary Mn doped ZnO nanoparticles grafted on reduced graphene oxide (RGO) sheet as an efficient solar light driven photocatalyst, Chem. Phys. Lett., 2018, 710, 70–77 CrossRef CAS.
- D. R. Paul, R. Sharma, P. Panchal, R. Malik, A. Sharma, V. K. Tomer and S. P. Nehra, Silver doped graphitic carbon nitride for the enhanced photocatalytic activity towards organic dyes, J. Nanosci., 2019, 19, 5241–5248 CAS.
- Z. Wu, X. Chen, X. Liu, X. Yang and Y. Yang, A ternary magnetic recyclable ZnO/Fe3O4/g-C3N4 composite photocatalyst for efficient photodegradation of monoazo dye, Nanoscale Res. Lett., 2019, 14, 147–151 CrossRef PubMed.
- L. Anju Chanu, W. Joychandra Singh, K. Jugeshwar Singh and K. Nomita Devi, Effect of operational parameters on the photocatalytic degradation of Methylene blue dye solution using manganese doped ZnO nanoparticles, Results Phys., 2019, 12, 1230–1237 CrossRef.
- M. Ikram, E. Umar, A. Raza, A. Haider, S. Naz, D. A. Ul-Hamid, J. Haider, I. Shahzadi, J. Hassan and S. Ali, Dye degradation performance, bactericidal behavior and molecular docking analysis of Cu-doped TiO2 nanoparticles, RSC Adv., 2020, 10, 24215–24233 RSC.
- R. S. Das, S. K. Warkhade, A. Kumar, G. S. Gaikwad and A. V. Wankhade, Graphitic carbon nitride@silver zirconate nanocomposite (g-C3N4@Ag2ZrO3): a type-II heterojunction for an effective visible light photocatalysis and bacterial photo inactivation, J. Alloys Compd., 2020, 155770 CrossRef CAS.
- R. Nithya, S. Ragupathy, D. Sakthi, V. Arun and N. Kannadasan, A study on Mn doped ZnO loaded on CSAC for the photocatalytic degradation of brilliant green dye, Chem. Phys. Lett., 2020, 755, 137769 CrossRef CAS.
- S. Ma, S. Zhan, Y. Xia, P. Wang, Q. Hou and Q. Zhou, Enhanced photocatalytic bactericidal performance and mechanism with novel Ag/ZnO/g-C3N4 composite under visible light, Catal. Today, 2019, 330, 179–188 CrossRef CAS.
- Y. Wu, Y. Zhou, H. Xu, Q. Liu, Y. Li, L. Zhang, H. Liu, Z. Tu and X. Cheng, Highly active, superstable, and biocompatible Ag/polydopamine/g-C3N4 bactericidal photocatalyst: synthesis, characterization, and mechanism, ACS Sustainable Chem. Eng., 2018, 6, 14082–14094 CrossRef CAS.
- J. C. Wang, H. C. Yao, Z. Y. Fan, L. Zhang, J. S. Wang, S. Q. Zang and Z. J. Li, Indirect Z- scheme BiOI/g-C3N4 photocatalysts with enhanced photoreduction CO2 activity under visible light irradiation, ACS Appl. Mater. Interfaces, 2016, 8, 3765–3775 CrossRef CAS PubMed.
- S. C. Yan, S. B. Lv, Z. S. Li and Z. G. Zou, Organic-inorganic composite photocatalyst of g-C3N4 and TaON with improved visible light photocatalytic activities, Dalton Trans., 2010, 39, 1488–1491 RSC.
- Y. Feng, Y. Wang, M. Li, S. Lv, W. Li and Z. Li, Novel
visible light induced Ag2S/g-C3N4/ZnO nanoarrays heterojunction for efficient photocatalytic performance, Appl. Surf. Sci., 2018, 462, 896–903 CrossRef CAS.
- M. J. Fang, C. W. Tsao and Y. J. Hsu, Semiconductor nanoheterostructures for photoconversion applications, J. Phys. D: Appl. Phys., 2020, 53, 143001 CrossRef CAS.
- M. A. Qamar, S. Shahid and M. Javed, Synthesis of dynamic g-C3N4/Fe@ZnO nanocomposites for environmental remediation applications, Ceram. Int., 2020, 46, 22171–22180 CrossRef CAS.
- M. A. Qamar, S. Shahid, S. A. Khan, S. Zaman and M. N. Sarwar, Synthesis characterization, optical and antibacterial studies of Co-doped SnO2 nanoparticles, Dig. J. Nanomater. Biostructures, 2017, 12, 1127–1135 Search PubMed.
- M. A. Qamar, S. Shahid, M. Javed, S. Iqbal, M. Sher and M. B. Akbar, Highly efficient g-C3N4/Cr-ZnO nanocomposites with superior photocatalytic and antibacterial activity, J. Photochem. Photobiol., A, 2020, 401, 112776 CrossRef CAS.
- S. Iqbal, N. Ahmad, M. A. Qamar, M. Javed, A. Bahadur, S. Ali, Z. Ahmad, R. M. Irfan, G. Liu, M. B. Akbar and M. A. Qayyum, Designing highly potential photocatalytic comprising silver deposited ZnO NPs with sulfurized graphitic carbon nitride (Ag/ZnO/Sg-C3N4) ternary composite, J. Environ. Chem. Eng., 2020, 9(2021), 104919 Search PubMed.
- M. A. Qamar, S. Shahid, M. Javed, M. Sher, S. Iqbal, A. Bahadur and D. Li, Fabricated novel g-C3N4/Mn doped ZnO nanocomposite as highly active photocatalyst for the disinfection of pathogens and degradation of the organic pollutants from wastewater under sunlight radiations, Colloids Surf., A, 2020, 125863 Search PubMed.
Footnote |
† Electronic supplementary information (ESI) available. See DOI: 10.1039/d1ra07203j |
|
This journal is © The Royal Society of Chemistry 2021 |
Click here to see how this site uses Cookies. View our privacy policy here.