DOI:
10.1039/D1RA06676E
(Paper)
RSC Adv., 2021,
11, 35711-35717
Synthesis of dimethyl carbonate from methanol and CO2 under low pressure†
Received
6th September 2021
, Accepted 27th October 2021
First published on 4th November 2021
Abstract
A mild and highly efficient approach has been developed for the direct synthesis of dimethyl carbonate (DMC) from methanol and CO2 under low initial pressure. The key to a successful transformation is the use of 1,8-diazabicyclo[5.4.0]undec-7-ene (DBU), CH2Br2 and ionic liquid. Under the optimized reaction conditions, the yield of DMC was obtained up to 81% under 0.25 MPa. The direct synthesis of DMC can be carried out at balloon pressure using CH2Br2 and DBU. In this case, after the reaction, DBU was proved to be recyclable after having been treated with KOH in ethanol. In addition, a plausible mechanism for this synthetic reaction was proposed according to the experimental results.
1. Introduction
With the continuous development of the greenhouse effect, the excessive emission of CO2, one of the main causes of this effect, has attracted widespread attention.1 The effective use of CO2 for the benefit of mankind has always been one of the focuses of scientists since CO2 is a non-toxic, abundant, and economical C1 chemical source. Among these uses, the capture of CO2,2 activation and conversion of CO2 (ref. 3 and 4) are the most attractive. Converting CO2 to dimethyl carbonate (DMC) is an important direction for the effective use of CO2 since this conversion has a 100% atom economy and conforms to the concept of Green Chemistry.5,6 DMC is a green chemical product with a wide range of uses since it is an eco-friendly solvent and versatile intermediate. It can replace toxic dimethyl sulfate, methyl iodide, and phosgene as methylation reagents and carbonylation reagents.7 It is deemed to be a gasoline additive in place of methyl tert-butyl ether (MTBE) to increase the gasoline octane number.8 Besides, DMC can also be used as an additive for electrolytes in lithium batteries and as a solvent for dopes and inks.9 In 1992, it was listed as a non-toxic product in Europe. Therefore, the development of efficient and green synthetic routes for DMC has attracted extensive attention.
So far, various methods have been reported to synthesize DMC, including methanol phosgenation, oxidative carbonylation of methanol, urea alcoholysis, two-step transesterification, one-pot synthesis, and direct synthesis. Methanol phosgenation was the unique commercialized available method for producing DMC until 1980. Nonetheless, it is limited due to the employment of toxic and hazardous phosgene.10 The synthesis of DMC by oxidative carbonylation of methanol has improved the yield and atom utilization.11 However, it is recognized that the reaction mixture is highly flammable and hazardous. In recent years, the preparation of DMC by urea alcoholysis has been developed.12 In this process, thermodynamic limitations and ammonia interferences should be concerned although this method is environmentally friendly. In the first step of transesterification method cyclic carbonate was produced using CO2 and epoxide as starting materials,13,14 and then reacted with methanol to produce DMC and the corresponding 1,2-diols.15 High energy consumption and investment occurred by the separation of intermediate products in two-step transesterification. One-pot synthesis of DMC has been proposed as an alternative route,16,17 but this method is generally accompanied by side reactions. Given the disadvantages mentioned above, the direct synthesis of DMC from methanol and CO2 is more attractive in terms of green technology, atomic utilization, and cost.
On account of the high stability of CO2 and the limitation of thermodynamic equilibrium, direct synthesis of DMC is subjected to two problems: extreme operating conditions and low conversion. Therefore, the capture and activation of CO2 are the key issues to achieve this transformation, and catalysts and activators play an extremely important role in the process. To date, numerous catalysts and activators for this transformation have been reported, including metal carbonates,18 organotin compounds,19 metal oxides,20,21 dehydrating agent22–29 and ionic liquids (ILs),30–32 etc. A list of the literature reported systems for the synthesis of DMC has been provided in the ESI (Table S1†). Although many efforts have been made for direct synthesis of DMC, these methods usually encounter with high reaction temperature, long reaction time, high pressure, and low production efficiency. ILs can be used as CO2 adsorbents due to their special physical and chemical properties. By selecting appropriate cations and anions, the solubility of CO2 in the reaction solution can be improved.33 A bi-component system of tri-cationic ILs/super base was developed by Chaugule et al.34 and a 37.1% conversion of CH3OH was obtained at 130 °C under 7.5 MPa CO2. It is reported that DBU can capture and activate CO2 and produce an amidinium alkyl carbonate intermediate [DBUH]+[OCOOR]− in the presence of alcohol.35–38 Lim et al.39 reported that CH2Br2 was a unique solvent in the synthesis of dibenzyl carbonate from benzyl alcohol and CO2. Later, Zhao et al.40 found that CH2Br2 was the optimum solvent for the direct synthesis of DMC in the presence of imidazolium bicarbonate ([CnCmIm][HCO3]). Therefore, the combination of DBU and CH2Br2 is expected to play a crucial role in the efficient transformation of DMC from CO2 and methanol in the presence of ILs. In this paper, we report a mild and efficient approach for direct synthesis of DMC from methanol and CO2 under low initial pressure in the presence of CH2Br2, DBU, and ionic liquid. The reaction can be carried out under balloon pressure, providing a green process with more application prospects for direct synthesis of DMC.
2. Experimental section
2.1 Materials
CO2 was purchased from Dalian Guangming Special Gas Co., Ltd. The other commercially available starting materials were purchased and used without any prior purification unless otherwise indicated.
2.2 Techniques used
NMR spectra were recorded on a Brucker Advance II 400 spectrometer using TMS as an internal standard (400 MHz for 1H NMR). ESI-MS were obtained on a Thermo Scientific LTQ Orbitrap XL mass spectrometer. Gas chromatography (GC) analysis was recorded on a gas chromatograph (Agilent 7820) equipped with a capillary column (HP-5, 30 m × 320 μm × 0.25 μm) using a flame ionization detector with a flow rate of 1 mL min−1 using the following method: the oven temperature was held at 50 °C for 5 min and then increased linearly to 240 °C over 20 min with a final hold of 5 min.
2.3 General procedure for the direct synthesis of DMC
For the direct synthesis of DMC, the reactions were conducted in a 25 mL stainless-steel autoclave equipped with a magnetic stirrer and automatic temperature control system. A typical reaction was carried out as follows: an appropriate amount of CO2 was charged to an autoclave containing a mixture of CH3OH (1.0 mmol), 1-ethyl-3-methylimidazole hexafluoro-phosphate (IL, 1.0 mmol), DBU (1.5 mmol), CH2Br2 (3.0 mmol) and CH3CN (0.3 mL) at room temperature. Then, the autoclave was placed in an oil bath preheated to the designated temperature. After stirred for a period the autoclave was cooled down to room temperature, and the remaining CO2 was removed slowly. The reaction mixture was analyzed by GC using biphenyl as an internal standard.
3. Results and discussion
3.1 Effect of solvent on the direct synthesis of DMC
Direct synthesis of DMC from CH3OH and CO2 in the presence of DBU and IL was carried out systematically. The solvents were first screened under the conditions of oil bath temperature 60 °C, initial pressure 0.25 MPa, reaction time 6 h, methanol 1.0 mmol, DBU 1.0 equivalent and IL 1.0 equivalent. The experimental results are shown in Table 1. The conversion of methanol was 50% and the yield of DMC was 42% in CH2Br2 (Table 1, entry 1). The DMC yield was only 16% in CH2Cl2 (Table 1, entry 2). The conversions of methanol were above 71% in CH3(CH2)3Br and CH3CH2Br, and the yields of DMC were 6% and 4%, respectively (Table 1, entries 3 and 4). Butyl methyl carbonate (BMC) and dibutyl carbonate were found as byproducts in CH3(CH2)3Br, and the byproducts in CH3CH2Br were ethyl methyl carbonate (EMC) and diethyl carbonate, analyzed by GC-MS. It was found that no DMC was generated in CH3CN, THF and DMF (Table 1, entries 5–7). In summary, CH2Br2 was selected as the solvent to carry out the reaction.
Table 1 Effect of solvent on the direct synthesis of DMCa
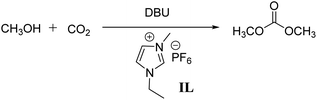
|
Entry |
Solvent |
Con.b (%) |
Yieldc (%) |
Reaction conditions: CH3OH 1.0 mmol, IL 1.0 mmol, DBU 1.0 mmol, solvent 2.0 mL, CO2 initial pressure 0.25 MPa, 60 °C, 6 h. Conversion of CH3OH. Yield of DMC determined by GC using an internal standard technique. |
1 |
CH2Br2 |
50 |
42 |
2 |
CH2Cl2 |
27 |
16 |
3 |
CH3(CH2)3Br |
78 |
6 |
4 |
CH3CH2Br |
71 |
4 |
5 |
CH3CN |
2 |
0 |
6 |
THF |
1 |
0 |
7 |
DMF |
0 |
0 |
3.2 Effect of CH2Br2 volume and DBU loadings on the direct synthesis of DMC
The effect of CH2Br2 volume and DBU loading on the direct synthesis of DMC has been investigated and the results are shown in Table 2. It can be seen from the results that the conversion of methanol was 58% and the yield of DMC was 50% using 0.5 mL CH2Br2 (Table 2, entry 1). Increasing the volume of CH2Br2 to 1.0 mL, the methanol conversion and the DMC yield were 57% and 48%, respectively (Table 2, entry 2). The conversion and the yield did not increase on further increasing the volume of CH2Br2 (Table 2, entries 3–5). No DMC was generated in the absence of DBU (Table 2, entry 6). The yield of DMC increased from 25 to 62% in the range of DBU loadings from 0.50 to 1.50 molar equiv (Table 2, entries 1, 7–9). Further increasing the DBU loading to 1.75 molar equiv did not lead to an increase in DMC yield (Table 2, entry 10). In summary, 0.5 mL of CH2Br2 and 1.50 molar equiv of DBU were considered to be the optimal reaction conditions on the direct synthesis of DMC.
Table 2 Effect of CH2Br2 volume and DBU loadings on the direct synthesis of DMCa
Entry |
CH2Br2 (mL) |
DBU (equiv) |
Con.b (%) |
Yieldc (%) |
Sel. (%) |
Reaction conditions: CH3OH 1.0 mmol, IL 1.0 mmol, CO2 initial pressure 0.25 MPa, 60 °C, 6 h. Conversion of CH3OH. Yield of DMC determined by GC using an internal standard technique. |
1 |
0.5 |
1.00 |
58 |
50 |
86 |
2 |
1.0 |
1.00 |
57 |
48 |
84 |
3 |
1.5 |
1.00 |
53 |
44 |
84 |
4 |
2.0 |
1.00 |
50 |
42 |
84 |
5 |
2.5 |
1.00 |
51 |
42 |
83 |
6 |
0.5 |
0 |
3 |
0 |
0 |
7 |
0.5 |
0.50 |
32 |
25 |
61 |
8 |
0.5 |
1.25 |
64 |
55 |
86 |
9 |
0.5 |
1.50 |
72 |
62 |
86 |
10 |
0.5 |
1.75 |
72 |
62 |
86 |
3.3 Effect of initial pressure and oil bath temperature on the direct synthesis of DMC
The influence of initial pressure and oil bath temperature on the direct synthesis of DMC has been investigated and the results are shown in Table 3. It can be seen from the results that when the initial pressure was 0.15 MPa, the conversion of methanol was 61% and the yield of DMC was 43% (Table 3, entry 1). At 0.25 MPa, the methanol conversion and the DMC yield were increased to 72% and 62%, respectively (Table 3, entry 2). After that, the yield of DMC showed a downward trend as the initial pressure increased from 0.25 to 1.50 MPa (Table 3, entries 2–5). It is speculated that the reason for this phenomenon may be related to the dilution effect. As the initial pressure increases, excess CO2 in the reaction system will reduce the concentration of methanol near the DBU.41,42 When the oil bath temperature was 25 °C, the methanol conversion and the DMC yield were only 17% and 8%, respectively (Table 3, entry 6). This result indicates that a lower temperature is not conducive to the reaction. When the temperature was lower than 60 °C, the DMC yield gradually increased with the increase of temperature (Table 3, entries 6–8). At 60 °C, the yield of DMC reached a maximum of 62% (Table 3, entry 2). When the temperature was higher than 60 °C, the yield of DMC decreased (Table 3, entries 9–11). The reason may be that as the temperature increases, the solubility of CO2 in the solution will decrease, thereby reducing the efficiency of DBU in combination with CO2. In addition, high temperatures will promote some side reactions, such as the decomposition of DMC into some gaseous products.43 Hence, it is considered that 0.25 MPa and an oil bath temperature of 60 °C were selected as the optimal conditions for direct synthesis of DMC.
Table 3 Effect of initial pressure and oil bath temperature on the reactiona
Entry |
Initial pressure (MPa) |
T (°C) |
Con.b (%) |
Yieldc (%) |
Sel. (%) |
Reaction conditions: CH3OH 1.0 mmol, IL 1.0 mmol, DBU 1.5 mmol, CH2Br2 0.5 mL, 6 h. Conversion of CH3OH. Yield of DMC determined by GC using an internal standard technique. |
1 |
0.15 |
60 |
61 |
43 |
71 |
2 |
0.25 |
60 |
72 |
62 |
86 |
3 |
0.50 |
60 |
69 |
57 |
83 |
4 |
1.00 |
60 |
66 |
53 |
80 |
5 |
1.50 |
60 |
65 |
52 |
80 |
6 |
0.25 |
25 |
17 |
8 |
47 |
7 |
0.25 |
40 |
37 |
22 |
59 |
8 |
0.25 |
50 |
65 |
52 |
80 |
9 |
0.25 |
70 |
70 |
60 |
86 |
10 |
0.25 |
80 |
64 |
56 |
87 |
11 |
0.25 |
100 |
61 |
52 |
85 |
3.4 Effect of IL loading on the direct synthesis of DMC
The effect of IL loading on the direct synthesis of DMC has been investigated and the results are shown in Table 4. It can be seen that the yield of DMC was 21% in the absence of IL (Table 4, entry 1). The DMC yield increased from 34 to 62% in the range of IL loading increasing from 0.25 to 1.00 molar equiv (Table 4, entries 2–5). After that, on further increasing the IL loading, the DMC yield did not increase anymore (Table 4, entries 6 and 7). Therefore, an IL loading of 1.00 equiv was considered to be the optimal reaction condition.
Table 4 Effect of IL loading on the direct synthesis of DMCa
Entry |
IL (equiv.) |
Con.b (%) |
Yieldc (%) |
Sel. (%) |
Reaction conditions: CH3OH 1.0 mmol, DBU 1.5 mmol, CH2Br2 0.5 mL, CO2 initial pressure 0.25 MPa, 60 °C, 6 h. Conversion of CH3OH. Yield of DMC determined by GC using an internal standard technique. |
1 |
0 |
40 |
21 |
52 |
2 |
0.25 |
48 |
34 |
71 |
3 |
0.50 |
59 |
43 |
73 |
4 |
0.75 |
66 |
55 |
83 |
5 |
1.00 |
72 |
62 |
86 |
6 |
1.25 |
72 |
62 |
86 |
7 |
1.50 |
73 |
63 |
86 |
3.5 Effect of the reaction time on the direct synthesis of DMC
Reaction times in the range of 3–18 h have been tested and the results are shown in Fig. 1. It can be seen that the methanol conversion and the DMC yield would gradually increase with the extension of the reaction time. When the reaction time was 12 h, an 81% conversion of methanol and a 73% yield of DMC were obtained. On further prolonging the reaction time to 18 h, the yield of DMC remained roughly constant. Therefore, a reaction time of 12 h was considered to be the optimal reaction time for direct synthesis of DMC.
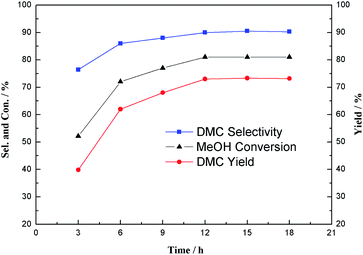 |
| Fig. 1 Effect of the reaction time on the direct synthesis of DMC. Reaction conditions: CH3OH 1.0 mmol, IL 1.0 mmol, DBU 1.5 mmol, CH2Br2 0.5 mL, CO2 initial pressure 0.25 MPa, 60 °C. The yield of DMC was determined by GC using an internal standard technique. | |
3.6 Effect of the loading of CH2Br2 as an additive on the direct synthesis of DMC
In the case of CH2Br2 as the solvent, the effect of CH2Br2 volume on the reaction was investigated (see Table 2). It was found that the smaller the volume, the higher the DMC yield. However, further decreasing the volume of solvent less than 0.5 mL, the reaction mixture was sticky and not conducive to the progress of this reaction. Thus, the volume of solvent was screened to a minimum of 0.5 mL (Table 2, entry 1). In this section, the effect of the loading of CH2Br2 as an additive on this reaction was investigated in detail. The results are shown in Table 5. It can be seen from the results that no DMC was formed without CH2Br2 when 0.5 mL CH3CN was used as a solvent (Table 5, entry 2). The DMC yield increased from 54% to 77% as the loading of CH2Br2 increased from 1.0 to 3.0 mmol (Table 5, entries 3–5). Further increasing the CH2Br2 loading to 6.0 mmol did not lead to an increase in DMC yield (Table 5, entries 6–8). The yield of DMC was slightly reduced in DMF or Toluene (Table 5, entries 9 and 10). The conversion of CH3OH was 76% and the yield of DMC was 65% using 0.1 mL CH3CN in the presence of 3.0 mmol CH2Br2 (Table 5, entry 11). Increasing the volume of CH3CN to 0.3 mL, the CH3OH conversion and the DMC yield were 83% and 81%, respectively (Table 5, entry 12). Further increasing the volume of CH3CN the conversion and the yield did not increase (Table 5, entries 13 and 14). Thus, 0.3 mL of CH3CN and 3.0 mmol of CH2Br2 were selected as the optimal conditions for the synthesis of DMC.
Table 5 Effect of the loading of CH2Br2 as an additivea
Entry |
Solvent (mL) |
CH2Br2 (mmol) |
Con.b (%) |
Yieldc (%) |
Sel. (%) |
Reaction conditions: CH3OH 1.0 mmol, IL 1.0 mmol, DBU 1.5 mmol, CO2 initial pressure 0.25 MPa, 60 °C, 12 h. Conversion of CH3OH. Yield of DMC determined by GC using an internal standard technique. |
1 |
— |
7.1 (0.5 mL) |
81 |
73 |
90 |
2 |
CH3CN (0.5) |
0 |
4 |
0 |
0 |
3 |
CH3CN (0.5) |
1.0 |
56 |
54 |
96 |
4 |
CH3CN (0.5) |
2.0 |
62 |
60 |
97 |
5 |
CH3CN (0.5) |
3.0 |
79 |
77 |
97 |
6 |
CH3CN (0.5) |
4.0 |
78 |
77 |
98 |
7 |
CH3CN (0.5) |
5.0 |
78 |
77 |
98 |
8 |
CH3CN (0.5) |
6.0 |
79 |
76 |
96 |
9 |
DMF (0.5) |
3.0 |
78 |
76 |
97 |
10 |
Toluene (0.5) |
3.0 |
76 |
73 |
96 |
11 |
CH3CN (0.1) |
3.0 |
76 |
65 |
86 |
12 |
CH3CN (0.3) |
3.0 |
83 |
81 |
98 |
13 |
CH3CN (0.7) |
3.0 |
74 |
73 |
98 |
14 |
CH3CN (0.9) |
3.0 |
53 |
51 |
96 |
3.7 Effect of the molecular structures of various ILs on the direct synthesis of DMC
Under the optimal conditions of oil bath temperature 60 °C, initial pressure 0.25 MPa, reaction time 12 h, methanol 1.0 mmol, DBU 1.5 mmol, ILs 1.0 mmol, CH3CN 0.3 mL, and CH2Br2 3.0 mmol, the effects of the molecular structures of various ILs on the direct synthesis of DMC have been investigated and the results are shown in Table 6. The influence of different anions on the reaction was investigated in the case of the same cation, and the IL with hexafluorophosphate was the most effective (Table 6, entries 1–8). The effect of the length of the alkyl chain on the cation was investigated in the case of the same anion, and the longer the carbon chain, the lower the yield of the product (Table 6, entries 7 and 9). The presence of hydroxyl group on the cation increased the methanol conversion but was not conducive to the formation of the product (Table 6, entries 8 and 10). When the anion was hexafluorophosphate, the presence of alkyl or benzyl group in the cation had little effect on the reaction (Table 6, entries 1 and 11).
Table 6 Effect of the molecular structures of ILs on the direct synthesis of DMCa
Entry |
ILs |
Con.b (%) |
Yieldc (%) |
Sel. (%) |
Reaction conditions: CH3OH 1.0 mmol, ILs 1.0 mmol, DBU 1.5 mmol, CH3CN 0.3 mL, CH2Br2 3.0 mmol, CO2 initial pressure 0.25 MPa, 60 °C, 12 h. Conversion of CH3OH. Yield of DMC determined by GC using an internal standard technique. |
1 |
 |
83 |
81 |
98 |
2 |
 |
73 |
67 |
92 |
3 |
 |
78 |
70 |
90 |
4 |
 |
82 |
79 |
96 |
5 |
 |
80 |
69 |
86 |
6 |
 |
82 |
70 |
85 |
7 |
 |
72 |
60 |
83 |
8 |
 |
54 |
50 |
93 |
9 |
 |
59 |
44 |
75 |
10 |
 |
65 |
35 |
54 |
11 |
 |
83 |
80 |
96 |
3.8 Direct synthesis of DMC under balloon pressure
The previous results show that the CH3OH conversion and the DMC yield were 61% and 43%, respectively at 0.15 MPa (Table 3, entry 1). Thus, the direct synthesis of DMC under balloon pressure has been studied and the results are shown in Table 7. Reaction times in the range of 10–15 h have been tested in the presence of IL. The yield of DMC reached a maximum of 62% in 12 h (Table 7, entry 2). On further prolonging the reaction time to 15 h, the yield of DMC kept constant (Table 7, entry 3). The DBU loading was investigated in 2 h without IL. The yield of DMC increased from 18 to 23% in the range of DBU loadings from 1.00 to 1.25 molar equiv (Table 7, entries 4 and 5). Further increasing the DBU loading to 1.50 molar equiv did not lead to an increase in DMC yield (Table 7, entry 6). Reaction times in the range of 1–6 h have been tested in the presence of 1.25 mmol DBU. The DMC yields in 2 h and 1 h were 23% and 16%, respectively (Table 7, entries 5 and 7). On further prolonging the reaction time to 6 h, the yield of DMC increased slightly (Table 7, entries 8 and 9). Considering the issue of energy consumption, a reaction time of 2 h was considered to be the optimal condition for direct synthesis of DMC under balloon pressure without IL.
Table 7 Direct synthesis of DMC under balloon pressurea
Entry |
DBU (mmol) |
Time (h) |
Con.b (%) |
Yieldc (%) |
Sel. (%) |
Reaction conditions: CH3OH 1.0 mmol, CH3CN 0.3 mL, CH2Br2 3.0 mmol, CO2 balloon, 60 °C. Conversion of CH3OH. Yield of DMC determined by GC using an internal standard technique. IL 1.0 mmol. |
1d |
1.50 |
10 |
60 |
58 |
97 |
2d |
1.50 |
12 |
64 |
62 |
97 |
3d |
1.50 |
15 |
64 |
62 |
97 |
4 |
1.00 |
2 |
32 |
18 |
56 |
5 |
1.25 |
2 |
33 |
23 |
70 |
6 |
1.50 |
2 |
33 |
23 |
70 |
7 |
1.25 |
1 |
27 |
16 |
59 |
8 |
1.25 |
4 |
35 |
24 |
69 |
9 |
1.25 |
6 |
37 |
24 |
65 |
3.9 The reusability of DBU under balloon pressure
The reusability of DBU under balloon pressure has been investigated, and the results are shown in Table 8. When the reaction was finished, the acetonitrile, methanol, CH2Br2, DMC and other components in the reaction solution were removed by distillation under reduced pressure. After that, KOH in 3.0 mL ethanol was added to the residue, and the mixture was filtered after stirred at 70 °C for 3 h. Ethanol in the filtrate was removed by vacuum distillation, and the residue containing DBU was directly used in the next run. The DMC yield increased from 6 to 21% in the range of KOH loadings increasing from 0.50 to 1.25 mmol (Table 8, entries 1–4). The DMC yield was 16% in the presence of 1.50 mmol KOH (Table 8, entry 5). Therefore, 1.25 mmol KOH was selected for the subsequent study. The yield of DMC was 20% in the 2nd run (Table 8, entry 6) and decreased slightly to 18% in the 3rd run (Table 8, entry 7). Therefore, DBU is practically reusable from the above results.
Table 8 The reusability of DBU under balloon pressurea
Entry |
Base |
Loading (mmol) |
DMCb (%) |
Reaction conditions: CH3OH 1.0 mmol, DBU 1.25 mmol, CH3CN 0.3 mL, CH2Br2 3.0 mmol, CO2 balloon, 60 °C, 2 h. Yield of DMC determined by GC using an internal standard technique. 2nd run. 3rd run. |
1 |
KOH |
0.50 |
6 |
2 |
KOH |
0.75 |
10 |
3 |
KOH |
1.00 |
13 |
4 |
KOH |
1.25 |
21 |
5 |
KOH |
1.50 |
16 |
6c |
KOH |
1.25 |
20 |
7d |
KOH |
1.25 |
18 |
3.10 The scale-up experiment
The reaction was scaled up under the conditions of oil bath temperature 60 °C, initial pressure 0.25 MPa and reaction time 12 h. The specific steps are as follows: a mixture of 15.0 mmol CH3OH, 15.0 mmol IL, 22.5 mmol DBU, 45.0 mmol CH2Br2 and 4.5 mL CH3CN was added in a 75 mL stainless-steel autoclave, then CO2 was charged to the autoclave up to 0.25 MPa at room temperature. After that, the autoclave was placed in an oil bath preheated to 60 °C. After stirred for 12 h, the autoclave was cooled down to room temperature. The yield of DMC is 79% analyzed by GC using biphenyl as an internal standard. The scale-up experiment for direct synthesis of DMC under balloon pressure without IL was also carried out with a GC yield of 22% in 2 h.
3.11 Substrate scope in carbonate synthesis
We further studied the substrate scope in carbonate synthesis using different alcohols under the conditions as follows: oil bath temperature 60 °C, initial pressure 0.25 MPa, reaction time 12 h, alcohol 1.0 mmol, IL 1.0 mmol, CH2Br2 3.0 mmol, DBU 1.5 mmol and 0.3 mL acetonitrile. The reaction of CO2 with ethanol, 1-butanol or benzyl alcohol has been investigated and the results are shown in Table 9. It is clear that the yields of the corresponding carbonates are 80%, 82% and 79%, respectively.
Table 9 Substrate scope in carbonate synthesisa
Entry |
Alcohol |
Product |
Yieldb (%) |
Reaction conditions: alcohol 1.0 mmol, DBU 1.5 mmol, IL 1.0 mmol, CH3CN 0.3 mL, CH2Br2 3.0 mmol, CO2 initial pressure 0.25 MPa, 60 °C, 12 h. Yield of carbonate determined by GC using an internal standard technique. |
1 |
CH3OH |
 |
81 |
2 |
CH3CH2OH |
 |
80 |
3 |
CH3(CH2)3OH |
 |
82 |
4 |
 |
 |
79 |
3.12 The reactant control experiments
To explore the possible reaction mechanism, a series of substrate control experiments have been carried out and the results are shown in Scheme 1. Taking the process a as an example, the experimental steps are as follows: an appropriate amount of CO2 was charged to a 25 mL autoclave containing a mixture of 1.5 mmol DBU, 1.0 mmol methanol, and 0.3 mL CH3CN at room temperature, the autoclave was placed in an oil bath preheated to 60 °C. After stirred for 2 h, the autoclave was cooled down to room temperature, and the remaining CO2 was removed slowly. 1.0 mmol IL and 3.0 mmol CH2Br2 were added to the autoclave, then the autoclave was placed in the oil bath again. After stirred for 3 h, the autoclave was cooled down to room temperature. Gas chromatography was used to analyze the yield of DMC through a standard curve. It can be seen that the yield of DMC in the process a was 43%. The DMC yield was 22% in process b without IL. Whereas in process c, IL was added in the initial step and no product was observed in the subsequent reaction step. The results indicate that the reactive intermediate should be [DBUH]+[CH3OCOO]−,35–38 and IL acts as a promoter to react with this intermediate to increase the yield of DMC.
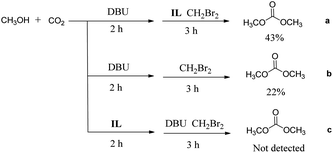 |
| Scheme 1 The reactant control experiments. | |
3.13 Proposed reaction mechanism
After the reaction, the reaction mixture was concentrated under reduced pressure and the reacted DBU and IL were isolated by column chromatography. The isolated DBU and IL were characterized by 1H NMR and HRMS (see Fig. S6–S13 in the ESI†). From these spectra, it can be inferred that mixed salts of protonated DBU, hexafluorophosphate anion, and bromide anion were formed after the reaction. Meanwhile, a part of IL was combined a group with a molecular weight of 31 during the reaction. According to the previously reported,34–40 as well as the results in this study, two plausible mechanism routes for direct synthesis of DMC have been proposed and shown in Scheme 2. The route-1 is that DBU combines with CO2 to form a DBU-CO2 adduct. Subsequently, the adduct is alcoholized by the nucleophilic attack of the O atom on the methanol, and the H atom of the hydroxyl group is transferred to the N atom of the DBU to form an amidinium alkyl carbonate intermediate I [DBUH]+[CH3OCOO]−. Intermediate I forms protonated DBU and intermediate III after being attacked by CH2Br2. The characteristic peak of intermediate III ([M + H]+ 170.81) was detected in the mass spectra of the reaction mixture (see Fig. S14 in the ESI†). Finally, intermediate III is attacked by the nucleophile methanol to form the final product DMC. Besides, DBU is proved to be recyclable after having been treated with KOH in ethanol.
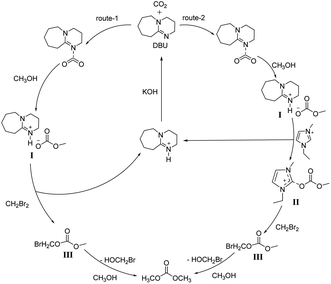 |
| Scheme 2 Proposed mechanism for the direct synthesis of DMC. | |
Intermediate I is formed firstly in the route-2. Then the carbon atom between the two nitrogen atoms of the imidazole cation in the IL combines with the oxygen ion in intermediate I to produce an intermediate II. This unstable intermediate is attacked by CH2Br2 to form intermediate III, then the target product DMC is generated. In view of the fact that IL can promote the production of DMC to increase the yield, we consider that the reaction undergoes both route-1 and route-2 in the presence of IL, while the reaction undergoes route-1 in the absence of IL.
4. Conclusions
In summary, a mild and efficient protocol for direct synthesis of DMC from CO2 and methanol under low initial pressure was developed in the presence of CH2Br2, DBU, and ILs. The reaction conditions were systematically investigated and the optimal conditions were obtained as follows: oil bath temperature 60 °C, initial pressure 0.25 MPa, reaction time 12 h, methanol 1.0 mmol, 1.0 equiv 1-ethyl-3-methylimidazole hexafluorophosphate, 3.0 equiv CH2Br2, 1.5 equiv DBU and 0.3 mL acetonitrile, resulting in an 81% yield of DMC. The reaction can be carried out under balloon pressure using CH2Br2, DBU with or without IL, providing the DMC yields of 62% (12 h) and 23% (2 h), respectively. DBU is proved to be recyclable in the absence of IL.
Conflicts of interest
There are no conflicts to declare.
Acknowledgements
The authors thank the National Natural Science Foundation of China` (21776036) for financial support.
References
- C. K. Ran, X. W. Chen, Y. Y. Gui, J. Liu, L. Song, K. Ren and D. G. Yu, Sci. China: Chem., 2020, 63, 1336–1351 CrossRef CAS.
- X. Y. Wang, T. He, J. H. Hu and M. Liu, Environ. Sci.: Nano, 2021, 8, 890–912 RSC.
- T. Sakakura, J. C. Choi and H. Yasuda, Chem. Rev., 2007, 107, 2365–2387 CrossRef CAS PubMed.
- Y. B. Wang, Y. M. Wang and W. Z. Zhang, J. Am. Chem. Soc., 2013, 135, 11996–12003 CrossRef CAS PubMed.
- B. M. Bhanage, S. I. Fujita and Y. Ikushima, Green Chem., 2003, 5, 71–75 RSC.
- P. Anastas and N. Eghbali, Chem. Soc. Rev., 2010, 39, 301–312 RSC.
- P. Tundo and M. Selva, Acc. Chem. Res., 2002, 35, 706–716 CrossRef CAS PubMed.
- K. Inamoto, C. Hasegawa and K. Hiroya, Chem. Commun., 2012, 48, 2912–2914 RSC.
- M. Aresta, A. Dibenedetto and A. Angelini, Chem. Rev., 2014, 114, 1709–1742 CrossRef CAS PubMed.
- D. Delledonne, F. Rivetti and U. Romano, Appl. Catal., A, 2001, 221, 241–251 CrossRef CAS.
- Y. Pei, J. Zhao, R. Shi, X. Wang, Z. Li and J. Ren, Catal. Lett., 2019, 149, 3184–3193 CrossRef CAS.
- Z. Hou, L. Luo, K. Liu, C. Liu, Y. Wang and L. Dai, Chem. Eng. J., 2014, 236, 415–418 CrossRef CAS.
- J. Wang, J. Leong and Y. Zhang, Green Chem., 2014, 16, 4515–4519 RSC.
- S. Zhang, H. Han, S. R. Yan, M. Y. He, C. X. Miao and L. N. He, Eur. J. Org. Chem., 2019, 6, 1311–1316 CrossRef.
- Q. Wei, G. Zhang, J. Yao, X. J. Chen, G. Y. Wang and X. G. Yang, New J. Chem., 2021, 45, 5540–5550 RSC.
- C. Liu, S. K. Zhang, B. Y. Cai and Z. L. Jin, Chin. J. Catal., 2015, 36, 1136–1141 CrossRef CAS.
- K. Liu and C. Liu, ACS Omega, 2021, 6, 13839–13846 CrossRef CAS PubMed.
- Q. Cai, B. Lu and L. Guo, Catal. Commun., 2009, 10, 605–609 CrossRef CAS.
- J. Kizlink and I. Pastucha, Collect. Czech. Chem. Commun., 1993, 58, 1399–1402 CrossRef CAS.
- Y. D. Chen, Q. Tang, Z. B. Ye, Y. Li, Y. Yang, H. Pu and G. Li, New J. Chem., 2020, 44, 12522–12530 RSC.
- W. Sun, L. Zheng, Y. Q. Wang, D. D. Li, Z. R. Liu, L. Wu, T. Fang and J. Q. Wu, Ind. Eng. Chem. Res., 2020, 59, 4281–4290 CrossRef CAS.
- M. Honda, A. Suzuki, B. Noorjahan, K. Fujimoto, K. Suzuki and K. Tomishige, Chem. Commun., 2009, 30, 4596–4598 RSC.
- M. Honda, S. Kuno, N. Begum, K. Fujimoto, K. Suzuki, Y. Nakagawa and K. Tomishige, Appl. Catal., A, 2010, 384, 165–170 CrossRef CAS.
- M. Honda, S. Kuno, S. Sonehara, K. Fujimoto, K. Suzuki, Y. Nakagawa and K. Tomishige, ChemCatChem, 2011, 3, 365–370 CrossRef CAS.
- M. Honda, M. Tamura, Y. Nakagawa, S. Sonehara, K. Suzuki, K. Fujimoto and K. Tomishige, ChemSusChem, 2013, 6, 1341–1344 CrossRef CAS PubMed.
- M. Honda, M. Tamura, Y. Nakagawa and K. Tomishige, Catal. Sci. Technol., 2014, 4, 2830–2845 RSC.
- M. Honda, M. Tamura, Y. Nakagawa, K. Nakao, K. Suzuki and K. Tomishige, J. Catal., 2014, 318, 95–107 CrossRef CAS.
- A. Bansode and A. Urakawa, ACS Catal., 2014, 4, 3877–3880 CrossRef CAS.
- D. Stoian, F. Medina and A. Urakawa, ACS Catal., 2018, 8, 3181–3193 CrossRef CAS.
- Q. H. Cai, L. Zhang, Y.-K. Shan and M. Y. He, Chin. J. Catal., 2010, 22, 422–424 Search PubMed.
- J. Sun, B. Lu, X. Wang, X. Li, J. Zhao and Q. H. Cai, Fuel Process. Technol., 2013, 115, 233–237 CrossRef CAS.
- A. A. Pawar, D. Lee, W. J. Chung and H. Kim, Chem. Eng. J., 2020, 395, 124970–124971 CrossRef CAS.
- Y. Chen and T. C. Mu, Green Chem., 2019, 21, 2544–2574 RSC.
- A. A. Chaugule, A. H. Tamboli and H. Kim, RSC Adv., 2016, 6, 42279–42287 RSC.
- W. D. McGhee, D. P. Riley, M. E. Christ and K. M. Christ, Organometallics, 1993, 12, 1429–1433 CrossRef CAS.
- D. J. Heldebrant, C. R. Yonker, P. G. Jessop and L. Phan, Energy Environ. Sci., 2008, 1, 487–493 RSC.
- Z. Z. Yang, L. N. He, Y. N. Zhao, B. Li and B. Yu, Energy Environ. Sci., 2011, 4, 3971–3975 RSC.
- Y. Q. Wang, Q. Z. Han and H. Wen, Mol. Simul., 2013, 39, 822–827 CrossRef CAS.
- Y. N. Lim, C. Lee and H. Y. Jang, Eur. J. Org. Chem., 2014, 2014, 1823–1826 CrossRef CAS.
- T. X. Zhao, X. B. Hu, D. S. Wu, R. Li, G. Q. Yang and Y. T. Wu, ChemSusChem, 2017, 10, 2046–2052 CrossRef CAS PubMed.
- Q. Gu, J. Fang and Z. Xu, New J. Chem., 2018, 42, 13054–13064 RSC.
- P. Challa, V. Rao and P. Nagaiah, J. Chem. Sci., 2019, 131, 86–96 CrossRef.
- B. Lu, X. Wang and Y. Li, J. CO2 Util., 2013, 3, 98–101 CrossRef.
Footnote |
† Electronic supplementary information (ESI) available: GC standard curves; the MS and NMR spectra for understanding the mechanism; a list of the literature reported systems for the synthesis of DMC. See DOI: 10.1039/d1ra06676e |
|
This journal is © The Royal Society of Chemistry 2021 |
Click here to see how this site uses Cookies. View our privacy policy here.