DOI:
10.1039/D1RA06416A
(Paper)
RSC Adv., 2021,
11, 31959-31966
Photochemistry of P,N-bidentate rhenium(I) tricarbonyl complexes: reactive species generation and potential application for antibacterial photodynamic therapy†
Received
24th August 2021
, Accepted 14th September 2021
First published on 27th September 2021
Abstract
In this work, we describe the photoisomerization of facial rhenium(I) tricarbonyl complexes bearing P,N-bidentate pyridyl/phosphine ligands with different chelating rings and anions: RePNBr, RePNTfO, and RePNNBr, which are triggered under irradiation at 365 nm in solutions. The apparent photodegradation rate constants (kapp) depend on the coordinating ability of the solvent, being lowest in acetonitrile. The kapp value increases as the temperature rises, suggesting a reactive IL excited state thermally populated from the MLCT excited state involved. Using the Eyring equation, positive activation enthalpies (ΔH≠) accompanied by high negative values for the activation entropy (ΔS≠) were obtained. These results suggest whatever the P,N-ligand or anion, the reaction proceeds through a strongly solvated or a compact transition state, which is compatible with an associative mechanism for the photoisomerization. A 100-fold decrease in the log10
CFU value is observed for E. coli and S. aureus in irradiated solutions of the compounds, which follows the same tendency as their singlet oxygen generation quantum yield: RePNBr > RePNTfO > RePNNBr, while no antibacterial activity is observed in the darkness. This result indicates that the generation of singlet oxygen plays a key role in the antibacterial capacity of these complexes.
Introduction
Rhenium(I) tricarbonyl diimines are attractive compounds due to their unique luminescence properties.1,2 They are easy to prepare, very stable at room temperature, and capable of emitting light in the visible region, and their photophysical properties are modulated by the ligands used.3,4 Owing to these advantages, they have been used in a wide range of applications as follows: as photocatalysts for CO2 reduction,5–7 as biomolecular probes for sensing different microenvironments,8,9 as DNA or protein probes for biolabeling,10,11 as sensitizers for Grätzel-type solar cells,12,13 and as singlet oxygen sensitizers for photodynamic therapy (PDT),14 among others.
It is now very well established that excitation with UV-Vis light of ReI–diimine complexes, [(N,N)Re(CO)3X] where X corresponds to a halide, mainly yields a triplet metal-to-ligand charge transfer (3MLCT) excited state.15–17 This is due to the extremely fast intersystem crossing process from the singlet to the triplet state, taking place normally in a few hundred femtoseconds and having a quantum yield close to unity.18,19 Then, the 3MLCT-excited state decays to the ground state by radiative and/or non-radiative processes or evolves to a photochemical path to yield products. Additionally, when molecular oxygen is present, energy transfer from 3MLCT to oxygen may occur, generating singlet oxygen, O2(1Δg).20–22
The photochemistry of ReI–diimine complexes has been less explored than their photophysical properties. Sato et al. reported that the facial isomer of [(bpy)Re(CO)3Cl] could be easily photo-converted into the meridional one under irradiation at 313 nm in a tetrahydrofuran solution.23 Despite their structural similarities, the photophysical properties of the fac and mer-isomers and their reactivities are different (Scheme 1). Sato and co-workers also demonstrated that fac-[(bpy)Re(CO)3Cl] (bpy = 2,2′-bipyridine) undergoes a photoinduced carbonyl substitution after excitation at 313 nm in an acetonitrile solution, yielding two isomers.24 The same photoreaction carried out in a constrained medium like a metal–organic framework (MOF) allows identifying the reactive intermediates, establishing a mechanism involving the release of one carbon monoxide molecule, CO.25
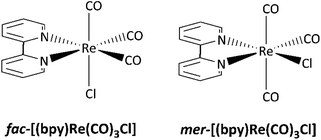 |
| Scheme 1 Facial and meridional isomers of [(bpy)Re(CO)3Cl].23 | |
However, while the halide ligand can be thermally replaced with other ligands (such as pyridine, monodentate phosphorus ligands or even coordinating solvent molecules), via chemical abstraction assisted by a silver salt, the carbonyl substitution only occurs when electronically excited states are involved (photochemical reaction),26–28 or when an auxiliary oxidant reagent like trimethylamine-N-oxide is employed.29 This lack of reactivity is attributed to the significant metal-to-carbonyl electron back-donation.30
Rhenium(I) complexes containing a monodentate phosphine ligand instead of a halide atom such as the cation fac-[(bpy)Re(CO)3(PR3)]+ have been stated to be selectively photoactive.26 There are reports of selective replacement of carbonyl ligands in the trans position relative to the phosphine ligand, with the release of a molecule of carbon monoxide (CO).27
The ability of some molecules to release carbon monoxide (CORMs) under controlled conditions is an interesting property considering the potential therapeutic use of the carbon monoxide against biological targets.31–34 Many transition metal compounds bearing carbonyl ligands generate carbon monoxide after exposure to light (photoCORMs).35 Irradiation with enough energy promotes d to π* transitions, thus increasing the oxidation of the metal and consequently weakening its union to the carbonyl.
Rhenium(I) complexes with hybrid P,N-bidentate ligands such as 2-pyridyl-diphenylphosphine (PN) and N-(diphenylphosphanyl)pyridin-2-amine (PNN) have been prepared for synthetic or catalytic purposes.36,37 We have recently reported the synthesis and photophysical properties of facial rhenium(I) tricarbonyl phosphine-imine compounds RePNBr, RePNNBr and RePNTfO (TfO: triflate, Scheme 2).38–41
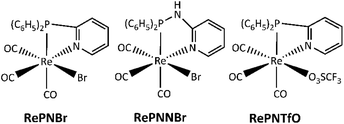 |
| Scheme 2 Structure of facial rhenium(I) complexes with P,N-bidentate ligands. | |
The experimental results indicate that the photophysical properties of these complexes differ from those of their homologs bearing N,N-diimine ligands. The former compounds exhibited lower emission quantum yields and shorter luminescence lifetimes, driven by a non-radiative decay process as the main deactivation path, the result of the higher conformational flexibility of the P,N-ligand.39 Interestingly, these complexes have been found to display dual emission attributed to two emissive MLCT excited states very close in energy:
39 In addition, these three complexes are able to generate singlet oxygen.39–41
Recently, the synergic action of singlet oxygen generation and photoinduced release of carbon monoxide by tricarbonyl metal complexes has been proposed as promising treatment against cancer.42–44 In this framework, rhenium complexes have been classified as good photoCORMs and photosensitizers for singlet oxygen generation, holding also great potential for antibacterial treatments.45
Today, there is an urgent need for novel agents to fight bacterial infections. Some standard antibiotics have no action against certain pathogens such as Gram-positive Staphylococcus aureus and Gram-negative Escherichia coli.46,47 The overuse and/or misuse of antibiotics has provoked pathogen evolution with higher toughness and novel resistance mechanisms to these antimicrobial agents, posing a tremendous threat to human health,48,49 increasing the search or development for new treatments like antibacterial photodynamic therapy (aPDT).50,51
To the best of our knowledge, no reports are available in the literature about the photochemistry of these or related complexes, despite they offer an opportunity to study the synergic action of singlet oxygen generation and photoinduced release of carbon monoxide in biological systems. Keeping this in mind, we decided to explore the relation between the photochemistry/photophysics of the rhenium complexes RePNBr, RePNNBr and RePNTfO and their antibacterial activity against S. aureus and E. coli.
Experimental
Reagents and solvents
Compounds RePNBr (fac-P,N-{(C6H5)2(C5H4N)P}Re(CO)3Br), RePNNBr (fac-P,N-{(C6H5)2(C5H4N)(NH)P}Re(CO)3Br), and RePNTfO (fac-P,N-{(C6H5)2(C5H4N)P}Re(CO)3(TfO) where TfO = O3SCF3), were synthesized as described in the literature.38,40,41 All solvents (Merck) were of spectroscopic or HPLC grade. Water was purified and deionized using a Waters Milli-Q system.
Apparatus and procedures
UV-Vis absorption spectra and steady-state kinetic experiments were performed using an Agilent 8453 diode-array spectrophotometer. Rhenium complexes were dissolved in ethanol (EtOH), dichloromethane (DCM) and acetonitrile (MeCN). The photolysis experiments were performed by irradiating 5 × 10−5 M solutions (3 mL) in a 10 mm fluorescence quartz cell, using a Blak-Ray B-100AP high-intensity mercury UV lamp (100 W) with a 365 nm filter at different temperatures (293 K, 303 K and 313 K). The distance between the quartz cell and the lamp was set at 10.0 cm, with the light source perpendicularly arranged to the sample. The photon flux (Ia) was determined by actinometry using 9,10-dimethylanthracene (DMA) in Freon 113, following the DMA consumption at 334 nm (Ia = 1.39 × 10−9 einstein per s).52 The kinetic parameters of photolysis were measured by recording the UV-Vis spectra at different irradiation times, using an Agilent 8453 diode-array spectrophotometer. Photolysis experiments followed by infrared measurements were also carried out using a Bruker-Vertex 70 FT-IR instrument.
Assuming a global reaction:
, the apparent kinetic rate constants of the photodegradation process (kapp) were obtained following the appearance of the product by monitoring the UV-Vis spectra at different temperatures, and fitting data to eqn (1) (please see ESI† for more details):53
|
Ap = εPl[A]o(1 − e−kappt)
| (1) |
where
AP and
εP correspond to the absorbance and molar extinction coefficient of the product, respectively,
l is the optical path and [
A]
o represents the initial concentration of the complex. It must be stated that the kinetic rate constants for the photochemical reaction are apparent, as they are dependent on the photon flux and quantum yield of the process,
54,55 however under well-controlled steady-state experimental conditions: irradiation with constant light intensity, geometry unaltered, similar extinction coefficients, these experimental rate constants, denoted as apparent, are fully comparable.
The quantum yields for product formation were calculated using eqn (2):56
|
 | (2) |
where d[
P]/d
t is the photoproduct rate formation,
V is the solution volume and
Ia is the photon flux previously determined by actinometry maintaining the geometry constant.
The activation parameters ΔS≠ and ΔH≠ were determined using Eyring eqn (3):57
|
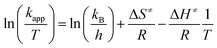 | (3) |
where
kB,
h and
R are the Boltzmann constant, Planck constant, and universal gas constant, respectively.
Antibacterial activity
S. aureus (ATCC 29213) and E. coli (ATCC 25922) strains were used to assess the antibacterial activity of the studied complexes. Bacteria were cultured in 50 mL flasks containing 10 mL Lysogeny Broth (LB) medium consisting of the following (per liter): 10 g tryptone, 5 g yeast extract, and 0.5 g NaCl. The flasks were placed in a rotary shaking (Orbitron, INFORS) set at 200 rpm and 37 °C for 16 h. Then, the same procedure was repeated as described above and after 5 h of bacterial culture, when the cells were in the middle of the exponential phase, 100 μL of culture were mixed with 700 μL fresh LB medium and 200 μL of complex solutions (initial colony forming units 5 × 105 CFU mL−1) for all the assays. The final established concentrations of the studied compounds were 50 μg mL−1 and 300 μg mL−1. Two independent groups of each concentration of rhenium complexes were tested. One group was not irradiated with UV light and the other one irradiated using a Blak-Ray B-100AP high-intensity mercury UV lamp (100 W) with a 365 nm filter for 1 min. Then, the mixture (1 mL of culture) was placed in a 10 mL culture tube and incubated at 37 °C under shaking (200 rpm). After 24 h of culture, the optical density was recorded at 600 nm using a spectrophotometer. Moreover, 100 μL of bacterial suspension was uniformly streaked onto LB agar plates and incubated at 37 °C for 24 h. Finally, the colony forming unit (CFU) was counted for the evaluation of the antimicrobial activity.
Computational details
Geometry optimizations for facial and meridional isomers of RePNBr were performed at the B3LYP/6-31+G(d,p) level of theory using the Gaussian 09 Rev C.01 package of programs (G09)58 starting with RePNBr crystallographic coordinates as input.38 The LANL2DZ basis set was used only for rhenium. Excitations were computed within the time-dependent density functional theory (TD-DFT) methodology, as implemented in G09. Absorption spectra were simulated from the above-mentioned calculations using the GaussSum 3.0 suite of freely available processing tools. A full width at half maximum (FWHM) of the Gaussian curves corresponding to 2500 cm−1 was employed to convolute the spectra.
Results and discussion
Photophysical properties
The facial rhenium tricarbonyl complexes bearing P,N-bidentate ligands, namely, RePNBr, RePNTfO, and RePNNBr (Scheme 2), have been characterized in previous works and their main photophysical properties are summarized in Table 1.39–41 Briefly, they present absorption bands around 315 nm with shoulders at 350 nm, the last, assigned to a metal-to-ligand charge transfer (MLCT) transition, which involves π* orbitals on the pyridine and phenyl rings.
Table 1 Summary of the main photophysical properties of the complexes in DCM at 298 K
Complex |
λabs (nm) |
λem (nm) |
Φem |
τem (ns) |
ΦΔ |
Ref. |
RePNBr |
315; 355(sh) |
550 |
5.0 × 10−4 |
3.8 (87); 39.1 (13) |
0.0640 |
39 |
RePNTfO |
295; 350(sh) |
535 |
1.2 × 10−3 |
2.6 (86); 11.6 (14) |
0.0200 |
40 |
RePNNBr |
300; 350(sh) |
365 |
1.3 × 10−2 |
2.3 (35); 6.4 (65) |
0.0015 |
41 |
Upon excitation at 350 nm, RePNBr and RePNTfO exhibit an emission band at around 540 nm with low emission quantum yield, while RePNNBr displays an intense emission at 365 nm with an emission quantum yield value of 0.013. This marked difference was attributed to the NH bridge's presence between the phosphorous and the pyridyl group, which modifies the energy levels of the ligand centered (IL) and the MLCT excited states, favoring the emissive path from the IL excited state.41 The 3MLCT state of all studied complexes is able to photosensitize singlet oxygen through an energy transfer process, with RePNBr having the highest value of singlet oxygen quantum yield (Table 1, ΦΔ). The short lifetimes and low emission quantum yields were attributed to the conformational flexibility conferred by the P,N-ligand, which could favor the non-radiative pathway.
Photochemical behaviour
Irradiation of the complexes in EtOH, DCM or MeCN solutions, at their respective lowest energy absorption bands, gives rise to photoproduct formation. Fig. 1 shows the spectral changes of RePNBr in EtOH upon irradiation at 365 nm at 20 °C. As can be observed, the absorption band of RePNBr at 315 nm decreases after irradiation, while two distinguishable bands at 267 nm and 400 nm increase. Similar spectral changes were observed in DCM but less pronounced in MeCN for the three complexes (see ESI†).
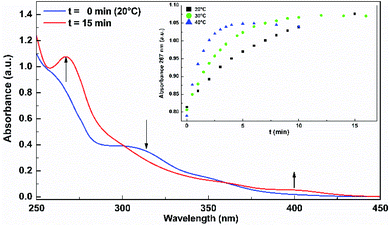 |
| Fig. 1 Spectral changes of RePNBr in EtOH upon irradiation at 365 nm at 20 °C. Inset: increase in absorbance attributed to the product appearance (267 nm) versus the irradiation time. | |
The inset in Fig. 1 exhibits an increase in absorbance attributed to the product appearance (267 nm) versus the irradiation time. Fitting these data with eqn (1) allows us to obtain the apparent photoproduct formation rate constant (kapp). In addition, photodegradation quantum yields (ΦP) were calculated at room temperature using eqn (2). The values of kapp obtained at different temperatures and ΦP in different solvents are included in Table 2.
Table 2 Summary of the apparent photoproduct formation rate constants (kapp) and photodegradation quantum yields (ΦP) for the studied complexesa
Solvent |
T (K) |
RePNBr |
RePNNBr |
RePNTfO |
kapp (10−3 s−1) |
ΦP |
kapp (10−3 s−1) |
ΦP |
kapp (10−3 s−1) |
ΦP |
Errors were lower than 10%. |
EtOH |
293 |
2.40 |
0.155 |
0.67 |
0.035 |
9.91 |
0.380 |
303 |
5.73 |
0.78 |
11.2 |
|
313 |
12.3 |
0.94 |
12.8 |
|
MeCN |
293 |
0.71 |
0.165 |
0.42 |
0.031 |
0.49 |
0.016 |
303 |
1.01 |
0.45 |
0.68 |
|
313 |
1.55 |
0.54 |
0.76 |
|
DCM |
293 |
9.05 |
0.345 |
2.07 |
0.092 |
2.76 |
0.191 |
303 |
12.2 |
2.24 |
3.60 |
|
313 |
15.8 |
2.48 |
5.26 |
|
The lowest apparent rate constant values and photodegradation quantum yields were observed in MeCN for the three complexes, while the highest ones were found for RePNBr in DCM and RePNTfO in EtOH. Similar rate constant values arise following the absorbance increase of the photoproduct absorbing at the high energy (267 nm in EtOH) or at the low energy absorption band (400 nm). The photolysis rate constant values were almost not affected by the presence of molecular oxygen, but to discard any other competitive process, all the samples were de-aerated. Besides, the apparent rate constant values increase with the rise in temperature, which is ascribed to a thermal population of the IL excited state from the MLCT excited state with a triplet character.59 In this context, we apply the transition state theory (Eyring) in order to get information about the transition state.57
Fig. 2 presents the Eyring plot for the photolysis of RePNBr in different solvents. Fitting the observed photodegradation rate constant values with eqn (2) allows us to get the Eyring parameters summarized in Table 3.
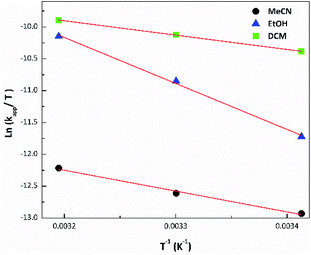 |
| Fig. 2 Eyring plot for the photolysis of RePNBr in different solvents. | |
Table 3 Summary of the activation thermodynamic parameters for the photolysis of the studied complexes
Solvent |
RePNBr |
RePNTfO |
RePNNBr |
ΔH‡ (kJ mol−1) |
ΔS‡ (J mol−1 K−1) |
ΔH‡ (kJ mol−1) |
ΔS‡ (J mol−1 K−1) |
ΔH‡ (kJ mol−1) |
ΔS‡ (J mol−1 K−1) |
EtOH |
60.0 |
−90.0 |
3.39 |
−258.4 |
25.2 |
−269.8 |
MeCN |
27.2 |
−212.3 |
14.2 |
−259.2 |
4.27 |
−288.1 |
DCM |
18.7 |
−219.9 |
31.6 |
−218.8 |
8.45 |
−281.5 |
The activation enthalpy (ΔH≠) values were all positive accompanied by high negative values for the activation entropy (ΔS≠), being the main contribution to ΔG≠. This major entropic factor might indicate a more ordered, compact, and probably highly solvated transition state, which is compatible with a reaction occurring through an associative mechanism (Ia).
Mechanism and theoretical calculations
Fig. 3a shows the IR spectral changes of the CO stretching bands after irradiation of RePNBr in DCM at room temperature. These changes are compatible with the formation of the mer isomer as was reported for other similar complexes.59 Theoretical calculations support this result, suggesting that isomerization slightly shifts the carbonyl stretching frequencies toward higher energy for the advisable mer-isomers (Scheme 3), as shown in Fig. 3b. Indeed, the trans effect of the phosphorous atom would be responsible to address the photoisomerization. Simulation of UV-Vis spectra (Fig. S5†) of each isomer also supports that observed spectral changes are related to an isomerization process, while Fig. S6† shows the DFT-computed vibrational circular dichroism spectra for fac-RePNBr and its mer-isomers.
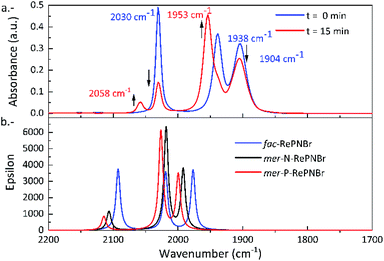 |
| Fig. 3 (a) IR (CO-stretching region) spectra of RePNBr in DCM with 0 min (blue) and 15 min (red) of irradiation (365 nm). (b) DFT-computed vibrational spectra for fac-RePNBr and mer-RePNBr isomers. | |
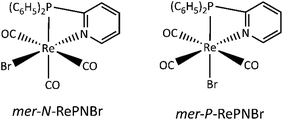 |
| Scheme 3 Possible mer-isomers for RePNBr. | |
As commented above, the negative activation entropy change for each complex in each solvent suggests the associative nature of the rate-determining step. We have previously shown that the replacement of triflate by bromide in RePNTfO follows this pattern, with a negative activation entropy change of about 60 J K−1 mol−1.40
Although coincidence, the largely higher negative (around 230 J K−1 mol−1 in average) values measured for isomerization entropies here are surprising and puzzling. They suggest a rate-determining step where new bonds and/or strong interactions yield an activated complex with less degrees of freedom, and probably also highly constrained in the solvent cage. According to Satos's work,24 a carbonyl loss reaction begins with an isomerization, involving the formation of penta-coordinate species, which isomerizes and then completes its coordination sphere, or by an associative mechanism, a nucleophilic attack of the solvent could yield to a seven-coordinate intermediate, being the last option the most probable for this particular case. However, Mukuta et al.60 suggested a strong solvated or even a coordinated solvent molecule-transition state to yield products via an associative mechanism. Data presented in Tables 2 and 3 also show that the reactions for RePNBr are under each condition significantly faster than that of the analogous complexes RePNTfO and RePNNBr, and that the coordinating solvents lower the isomerization rate. Finally, no release of carbon monoxide was detected in all the experiments performed.
We have previously shown that chelating 2-pyridylphosphine (PN) may be partially thermally decoordinated from RePNBr and replaced with piperidine, leaving [P-{(C6H5)2(C5H5N)P}(C5H11N)Re(CO)3Br].61 Taking account of all these experimental facts, we can propose that the reaction proceeds according the mechanistic sequence shown in Scheme 4.
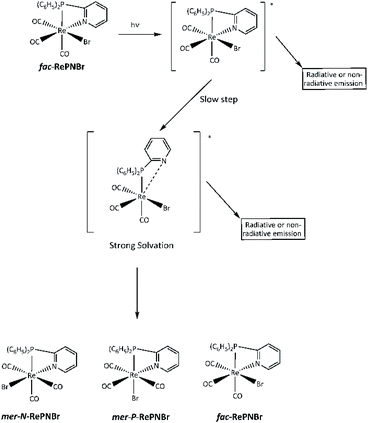 |
| Scheme 4 Proposed isomerization scheme for RePNBr photolysis. | |
The excitation of RePNBr by UV-Vis leads to an electronic redistribution from the metal to the ligand pyridyl group. One may speculate that this excited molecule would evolve to a highly solvated species, where some lengthening of the rhenium to nitrogen bond occurs. This partially de-coordinated species would quickly isomerize to the mer-isomer or re-coordinate the pyridyl arm if radiative emission occurs. The higher reactivity of RePNBr compared to RePNTfO can be attributed to the presence of the bromide ligand instance to the triflate group, which would facilitate the isomerization due to a trans effect that has also been observed for halide ligands.28 In addition, the shorter bit of the P,N-ligand in the case of RePNBr compared to RePNNBr would be the reason for its faster isomerization.
In vitro antibacterial activity
We then assessed the inhibitory effect of RePNBr, RePNTfO, and RePNNBr against two clinically important strains of bacteria, namely, E. coli and S. aureus. As displayed in Fig. 4A and B, the compounds without irradiation did not arrest E. coli growth significantly compared to microbial cultures without adding the complex chemicals. Conversely, S. aureus had a 90% decrease in cell viability when exposed to 300 μg mL−1 of RePNTfO and RePNBr (Fig. 4C). By irradiating the complexes with UV light for 1 min and further mixing it with the cell culture, RePNBr was the most efficient compound at inhibiting cell propagation for both bacterial strains (99,9%, Fig. 4A and C), independently of the used concentration. E. coli was less sensitive to 50 μg mL−1 of RePNTfO than S. aureus. The RePNNBr complex provoked cell arrest only against the Gram-negative bacteria E. coli employing 300 μg mL−1 of the irradiated chemical.
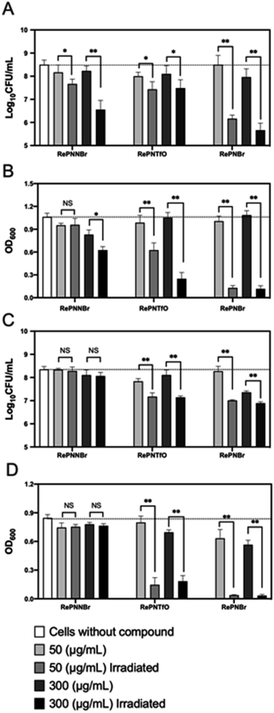 |
| Fig. 4 In vitro antibacterial activity of the complexes against E. coli and S. aureus at 37 °C after 24 h of cultivation. An initial culture of 5 × 105 CFU mL−1 was used. (A) E. coli cell growth (log10 CFU mL−1 versus rhenium complexes). (B) E. coli growth using optical density (OD600) determination. (C) S. aureus cell growth (log10 CFU mL−1 versus rhenium complexes). (D) S. aureus growth using optical density (OD600) determination. Mean values and standard deviations were obtained from three independent experiments. NS not significant, *p < 0.01, **p < 0.001. | |
Despite the multiple mechanisms displayed by Gram-positive and -negative bacteria to overcome oxidative stress, namely, the expression of superoxide dismutase, catalases, and peroxidases,62 or increased levels of glutathione and carotenoids, the results indicate that the pronounced reduction in cell viability—a 100-fold decrease in the log10
CFU value63—correlates properly with their singlet oxygen generation capacities, following the same decreasing order RePNBr > RePNTfO > RePNNBr.
Singlet oxygen being highly reactive with any oxidizable cellular compound, it alters cell functioning and facilitates Fe–S release from proteins inactivating their catalytic capacity and altering gene regulation. Besides, the liberated Fe–S species undergoes Fenton chemistry by reacting with H2O2, generating hydroxyl radicals (OH˙) within the cells and reacting virtually with any macromolecule (DNA, amino acids, RNA, proteins).64 Herein, we demonstrate that neither E. coli nor S. aureus can cope with the cascade of oxidative stresses provoked by the generated singlet oxygen from RePNBr and RePNTfO, making these compounds promising antibacterial agents.
Conclusions
The facial rhenium tricarbonyl complexes bearing P,N-bidentate ligands RePNBr, RePNTfO, and RePNNBr exhibited photoisomerization upon irradiation at 365 nm. The reaction occurs from an intraligand excited state, which is thermally populated from the metal-to-ligand charge transfer (3MLCT) excited state with triplet character. The apparent photodegradation rate constants depend on the coordinative properties of the solvent, with the lowest values being observed in acetonitrile. Fitting the measured photodegradation rate constants at different temperatures to the Eyring equation allows us to get the activation thermodynamic parameters of the transition state. Positive activation enthalpies (ΔH≠) accompanied by high negative values for the activation entropy (ΔS≠) were determined. This result suggests a strongly solvated or compact transition state which is compatible with an associative mechanism for the isomerization. The higher reactivity of RePNBr in comparison to RePNTfO and RePNNBr is due to the shorter bit of the P,N-ligand and to the bromide presence. As no release of carbon monoxide was detected, the antibacterial activity exhibited by the studied complexes is attributed to their singlet oxygen generation capacities, following the same decreasing order RePNBr > RePNTfO > RePNNBr. Because of this result, these compounds can be promising antibacterial agents.
Conflicts of interest
There are no conflicts to declare.
Acknowledgements
Authors acknowledge financial support from Fondecyt 1200903, 1200418, 1160749, 1160546, Conicyt-PIA-Anillo ACT 1404, ANID-PIA-Anillo INACH ACT192057 and UNAB DI-1253-16/R. N. P. thanks to Prof. Russell Schmehl for discussion and facilities at Tulane University.
Notes and references
- A. Kumar, S.-S. Sun and A. J. Lees, in Photophysics of Organometallics, ed. A. J. Lees, Springer Berlin Heidelberg, Berlin, Heidelberg, 2010, pp. 37–71, DOI:10.1007/3418_2009_2.
- D. Gupta and M. Sathiyendiran, ChemistrySelect, 2018, 3, 7439–7458 CrossRef CAS.
- D. J. Stufkens and A. Vlček, Coord. Chem. Rev., 1998, 177, 127–179 CrossRef CAS.
- D. A. Kurtz, K. R. Brereton, K. P. Ruoff, H. M. Tang, G. A. N. Felton, A. J. M. Miller and J. L. Dempsey, Inorg. Chem., 2018, 57, 5389–5399 CrossRef CAS PubMed.
- J. Hawecker, J.-M. Lehn and R. Ziessel, Helv. Chim. Acta, 1986, 69, 1990–2012 CrossRef CAS.
- H. Takeda, M. Ohashi, T. Tani, O. Ishitani and S. Inagaki, Inorg. Chem., 2010, 49, 4554–4559 CrossRef CAS PubMed.
- H. Takeda and O. Ishitani, Coord. Chem. Rev., 2010, 254, 346–354 CrossRef CAS.
- X. Q. Guo, F. N. Castellano, L. Li, H. Szmacinski, J. R. Lakowicz and J. Sipior, Anal. Biochem., 1997, 254, 179–186 CrossRef CAS PubMed.
- L. Li, F. N. Castellano, I. Gryczynski and J. R. Lakowicz, Chem. Phys. Lipids, 1999, 99, 1–9 CrossRef CAS PubMed.
- S. Hostachy, C. Policar and N. Delsuc, Coord. Chem. Rev., 2017, 351, 172–188 CrossRef CAS.
- A. M.-H. Yip and K. K.-W. Lo, Coord. Chem. Rev., 2018, 361, 138–163 CrossRef CAS.
- G. M. Hasselmann and G. J. Meyer, Z. Phys. Chem., 1999, 212, 39–44 CrossRef CAS.
- I. A. Wright, Polyhedron, 2018, 140, 84–98 CrossRef CAS.
- A. Leonidova, V. Pierroz, R. Rubbiani, J. Heier, S. Ferrari and G. Gasser, Dalton Trans., 2014, 43, 4287–4294 RSC.
- L. Sacksteder, A. P. Zipp, E. A. Brown, J. Streich, J. N. Demas and B. A. Degraff, Inorg. Chem., 1990, 29, 4335–4340 CrossRef CAS.
- R. M. Leasure, L. Sacksteder, D. Nesselrodt, G. A. Reitz, J. N. Demas and B. A. Degraff, Inorg. Chem., 1991, 30, 3722–3728 CrossRef CAS.
- L. Wallace and D. P. Rillema, Inorg. Chem., 1993, 32, 3836–3843 CrossRef CAS.
- A. Vlcek, Ultrafast Excited-State Processes in Re(I) Carbonyl-Diimine Complexes: From Excitation to Photochemistry, Springer, Berlin/Heidelberg, 2010 Search PubMed.
- A. El Nahhas, C. Consani, A. M. a. Blanco-Rodríguez, K. M. Lancaster, O. Braem, A. Cannizzo, M. Towrie, I. P. Clark, S. Záliš, M. Chergui and A. n. Vlček, Inorg. Chem., 2011, 50, 2932–2943 CrossRef CAS PubMed.
- A. A. Abdel-Shafi, J. L. Bourdelande and S. S. Ali, Dalton Trans., 2007, 2510–2516, 10.1039/b705524b.
- F. Ragone, H. H. Martinez Saavedra, P. M. David Gara, G. T. Ruiz and E. Wolcan, J. Phys. Chem. A, 2013, 117, 4428–4435 CrossRef CAS PubMed.
- E. Wolcan, Inorg. Chim. Acta, 2020, 509, 119650 CrossRef CAS.
- S. Sato, T. Morimoto and O. Ishitani, Inorg. Chem., 2007, 46, 9051–9053 CrossRef CAS PubMed.
- S. Sato, Y. Matubara, K. Koike, M. Falkenstrom, T. Katayama, Y. Ishibashi, H. Miyasaka, S. Taniguchi, H. Chosrowjan, N. Mataga, N. Fukazawa, S. Koshihara, K. Onda and O. Ishitani, Chem.–Eur. J., 2012, 18, 15722–15734 CrossRef CAS PubMed.
- T. L. Easun, J. Jia, J. A. Calladine, D. L. Blackmore, C. S. Stapleton, K. Q. Vuong, N. R. Champness and M. W. George, Inorg. Chem., 2014, 53, 2606–2612 CrossRef CAS PubMed.
- K. Koike, J. Tanabe, S. Toyama, H. Tsubaki, K. Sakamoto, J. R. Westwell, F. P. A. Johnson, H. Hori, H. Saitoh and O. Ishitani, Inorg. Chem., 2000, 39, 2777–2783 CrossRef CAS PubMed.
- K. Koike, N. Okoshi, H. Hori, K. Takeuchi, O. Ishitani, H. Tsubaki, I. P. Clark, M. W. George, F. P. A. Johnson and J. J. Turner, J. Am. Chem. Soc., 2002, 124, 11448–11455 CrossRef CAS PubMed.
- S. Sato, A. Sekine, Y. Ohashi, O. Ishitani, A. M. Blanco-Rodríguez, A. Vlček, T. Unno and K. Koike, Inorg. Chem., 2007, 46, 3531–3540 CrossRef CAS PubMed.
- D. J. Blumer, K. W. Barnett and T. L. Brown, J. Organomet. Chem., 1979, 173, 71–76 CrossRef CAS.
- C. Housecroft, Inorganic Chemistry, Prentice Hall, 4th edn, 2012 Search PubMed.
- A. F. Tavares, M. R. Parente, M. C. Justino, M. Oleastro, L. S. Nobre and L. M. Saraiva, PLoS One, 2013, 8, e83157 CrossRef PubMed.
- A. F. N. Tavares, M. Teixeira, C. C. Romao, J. D. Seixas, L. S. Nobre and L. M. Saraiva, J. Biol. Chem., 2011, 286, 26708–26717 CrossRef CAS PubMed.
- R. Motterlini and L. E. Otterbein, Nat. Rev. Drug Discovery, 2010, 9, 728–743 CrossRef CAS PubMed.
- I. Chakraborty, S. J. Carrington and P. K. Mascharak, Acc. Chem. Res., 2014, 47, 2603–2611 CrossRef CAS PubMed.
- R. D. Rimmer, A. E. Pierri and P. C. Ford, Coord. Chem. Rev., 2012, 256, 1509–1519 CrossRef CAS.
- B. Machura and R. Kruszynski, Polyhedron, 2006, 25, 1985–1993 CrossRef CAS.
- B. Machura and R. Kruszynski, J. Mol. Struct., 2007, 837, 92–100 CrossRef CAS.
- F. Venegas, N. Pizarro and A. Vega, J. Chil. Chem. Soc., 2011, 56, 823–826 CrossRef CAS.
- N. Pizarro, M. Duque, E. Chamorro, S. Nonell, J. Manzur, J. R. de la Fuente, G. Günther, M. Cepeda-Plaza and A. Vega, J. Phys. Chem. A, 2015, 119, 3929–3935 CrossRef CAS PubMed.
- G. Prado, M. B. Ibañez, A. Acosta, E. Chamorro, P. Hermosilla-Ibáñez, G. Günther, N. Pizarro and A. Vega, Polyhedron, 2017, 137, 222–230 CrossRef CAS.
- P. Mella, J. C. Palma, M. Cepeda-Plaza, P. Aguirre, J. Manzur, G. Günther, N. Pizarro and A. Vega, Polyhedron, 2016, 111, 64–70 CrossRef CAS.
- S. C. Marker, S. N. MacMillan, W. R. Zipfel, Z. Li, P. C. Ford and J. J. Wilson, Inorg. Chem., 2018, 57, 1311–1331 CrossRef CAS PubMed.
- R. N. Pickens, B. J. Neyhouse, D. T. Reed, S. T. Ashton and J. K. White, Inorg. Chem., 2018, 57, 11616–11625 CrossRef CAS PubMed.
- L. Wu, X. Cai, H. Zhu, J. Li, D. Shi, D. Su, D. Yue and Z. Gu, Adv. Funct. Mater., 2018, 28, 1804324 CrossRef.
- L. C.-C. Lee, K.-K. Leung and K. K.-W. Lo, Dalton Trans., 2017, 46, 16357–16380 RSC.
- B. A. Raho and B. Abouni, in The Battle Against Microbial Pathogens: Basic Science, Technological Advances and Educational Programs, ed. A. Méndez-Vilas, Formatex Research Centre, Spain, 2015, vol. I, pp. 637–648 Search PubMed.
- A. r. f. t. N. System, Am. J. Infect. Control, 2004, 32, 470–485 CrossRef.
- F. C. Tenover, Am. J. Med., 2006, 119, S3–S10 CrossRef CAS PubMed.
- M. Baym, L. K. Stone and R. Kishony, Science, 2016, 351, aad3292 CrossRef PubMed.
- Y. Liu, R. Qin, S. A. J. Zaat, E. Breukink and M. Heger, J. Clin. Transl. Res., 2015, 1, 140–167 Search PubMed.
- F. Cieplik, D. Deng, W. Crielaard, W. Buchalla, E. Hellwig, A. Al-Ahmad and T. Maisch, Crit. Rev. Microbiol., 2018, 44, 571–589 CrossRef CAS PubMed.
- H. J. Kuhn, S. E. Braslavsky and R. Schmidt, Pure Appl. Chem., 2004, 76, 2105–2146 CAS.
- M. Hippler, J. Chem. Educ., 2003, 80, 1074 CrossRef CAS.
- S. R. Logan, J. Chem. Educ., 1997, 74, 1303 CrossRef CAS.
- S. Toby, J. Chem. Educ., 2005, 82, 37 CrossRef CAS.
- H. Shaw and S. Toby, J. Chem. Educ., 1966, 43, 408 CrossRef CAS.
- S. Glasstone, K. J. Laidler and H. Eyring, The Theory of Rate Processes, McGraw-Hill, New York, 1941 Search PubMed.
- M. J. T. Frisch, G. W. Trucks, H. B. Schlegel, G. E. Scuseria, M. A. Robb, J. R. Cheeseman, G. Scalmani, V. Barone, B. Mennucci, G. A. Petersson, H. Nakatsuji and M. Caricato, et al., Revision D.01, Gaussian, Inc., Wallingford, CT, 2009 Search PubMed.
- S. Sato and O. Ishitani, Coord. Chem. Rev., 2015, 282–283, 50–59 CrossRef CAS.
- T. Mukuta, P. V. Simpson, J. G. Vaughan, B. W. Skelton, S. Stagni, M. Massi, K. Koike, O. Ishitani and K. Onda, Inorg. Chem., 2017, 56, 3404–3413 CrossRef CAS PubMed.
- F. Palominos, C. Muñoz, P. Oyarzun, M. Saldías and A. Vega, Acta Crystallogr., Sect. E: Crystallogr. Commun., 2019, 75, 1005–1010 CrossRef CAS PubMed.
- R. Gaupp, N. Ledala and G. Somerville, Front. Cell. Infect. Microbiol., 2012, 2, 33 Search PubMed.
- M. Tim, J. Photochem. Photobiol., B, 2015, 150, 2–10 CrossRef CAS PubMed.
- S. Jang and J. A. Imlay, J. Biol. Chem., 2007, 282, 929–937 CrossRef CAS PubMed.
Footnotes |
† Electronic supplementary information (ESI) available. See DOI: 10.1039/d1ra06416a |
‡ Current address: Biosystems Engineering Laboratory, Department of Chemical Engineering, Universidad de Santiago de Chile (USACH), Chile. |
|
This journal is © The Royal Society of Chemistry 2021 |
Click here to see how this site uses Cookies. View our privacy policy here.