DOI:
10.1039/D1RA06314F
(Paper)
RSC Adv., 2021,
11, 38219-38234
Enhanced exopolysaccharide yield and antioxidant activities of Schizophyllum commune fermented products by the addition of Radix Puerariae†
Received
20th August 2021
, Accepted 9th November 2021
First published on 26th November 2021
Abstract
To increase the production of exopolysaccharides (EPS) and expand the application of Schizophyllum commune (S. commune) fermentation liquid, the traditional Chinese medicine Radix Puerariae (RP) with outstanding biological activity was selected as a culture additive to improve the EPS yield and enhance the antioxidant activity of fermented products from S. commune. The effects of three independent factors: A: initial pH (5.0–6.0), B: concentration of RP (10–14 g L−1), and C: inoculum size (8–12%, v/v) on the EPS yield were evaluated. The results of response surface methodology (RSM) showed that the optimal fermentation conditions were: A: 5.40, B: 12.80 g L−1, and C: 10.0%. The optimal yield of EPS was 8.41 ± 0.12 mg mL−1, which showed an insignificant (p > 0.05) difference with the predicted value (8.45 mg mL−1). The fermented supernatants cultured from RP-supplemented medium (SC-RP) or regular medium (SC) were collected for further study. FT-IR analysis of EPS-1 (purified from SC) and EPS-2 (purified from SC-RP) showed that their structures were consistent, indicating that the addition of RP did not affect the structure of schizophyllan (SPG). In addition, compared with SC, the in vitro antioxidant activities of SC-RP were significantly improved with ORAC values and FRAP values increasing by 11.56-fold and 14.69-fold, respectively. There was a significant correlation among the phenolic compounds, flavonoids, and antioxidant activity of SC-RP in this study. Besides, SC-RP was detected to contain more than 25 bioactive ingredients compared with that of SC, which may play a key role in its antioxidant activities. Thus, these results indicated that RP enhanced the yield of SPG and improved the antioxidant activity of the fermented products by S. commune. Accordingly, the fermentation liquid of S. commune with the addition of RP may have potential application in food, cosmetics, and pharmaceutical industries.
1. Introduction
Recently, there have been increasing research interests in the antioxidant and antiaging effects of natural active ingredients such as polysaccharides, polyphenols, and flavonoids. β-Glucans are a family of glucopyranose polysaccharides mainly having a 1,3-β-glycosidic structure, which is abundant in the cell walls of bacteria, while β-1,4-branch linkage and β-1,6-side chain β-glucans are mostly found in cereals, yeasts, and mushrooms.1–3 Schizophyllan (SPG) is a polysaccharide produced by Schizophyllum commune (S. commune), a white-rot fungus and ubiquitous mushroom.4 The molecular structure of the main chain of SPG is a 1→3-β-D-glucopyranosyl unit with a (1→6)-branched β-D-glucopyranosyl substituent in every third unit.5,6 It is well known that several fungal polysaccharides with (l→3)-linked β-D-glucopyranose substituted at O-6 with single-unit β-D-glucopyranosyl side chains display host-mediated antitumor activity and immunomodulatory potential, which include lentinan from Lentinus edodes, SPG from S. commune, scleroglucan from Sclerotium glucanicum, tylopilan from Tylopilus felleus, grifolan-7N from Grifola frondosa, and hyaluronic acid β-glucan from Pleurotus ostreatus.7 SPG has been used in vaccines and anticancer therapies, as a bioactive cosmetic ingredient, in oxygen-impermeable films or antibacterial hydrogel for food preservation, and enhanced petroleum recovery.8–10 Furthermore, SPG has been registered as an anticancer drug in Japan, and its clinical efficacy has been demonstrated in patients with lung, cervical, and gastric cancers.11 SPG is also used as a promising topical delivery carrier for immunomodulation and vaccinations by hybridization with methacrylate or stearic acid.12,13 Furthermore, a study by D. Lee et al. showed that dietary SPG may offer a potential new role in regulating mitochondrial activity to achieve protective immunity in hosts.14 However, the long fermentation time (>3 d) and limited production rate (1.30 ± 0.02 to 4.20 ± 0.10 mg mL−1) of polysaccharides lead to high production costs, limiting the industrial production and commercialization of SPG.15,16
Radix Puerariae (RP), which is obtained from the root of Pueraria lobate (Willd.) Ohwi, is widely known as “Gegen” in traditional Chinese medicine17 and has a long history as herbal medicine and functional food in China.18 Isoflavonoids are plant polyphenolic antioxidants primarily found in medicinal and edible plants, which can be used as antipyretic, diaphoretic, and antiemetic agents. As is well known, the essential and major active ingredients of RP are isoflavones,19 which are a group of compounds having the aromatic heterocyclic skeleton of puerarin, genistein, and daidzein. Puerarin and some typical isoflavones from RP have shown beneficial effects on arteriosclerosis, hypertension, and diabetes mellitus, and also endow good antioxidant capacity to RP.20,21
Some studies have shown that RP shows good antioxidant activity in the fermentation of yeast and Monascus purpureus.22,23 Fermentation process may affect the properties of the substrate, leading to a notable promotion of the product characteristics, thus producing more excellent valuable compounds with bioactivities. RP is rich in starch, and thus can also act as a carbon source for fermentation; consequently, RP has potential to be an excellent substrate of fungi for their fermentation. When fermented by Aspergillus niger, the antioxidant activity of RP in vitro and its blood lipid-lowering effect in the body were improved.24 Also, S. commune can biotransform sophoricoside to synthesize new active substances with outstanding biological activities.25 Furthermore, selecting RP as the fermentation substrate of S. commune can remarkably improve the antioxidant activity of the fermentation liquid. However, there is a lack of studies on the liquid fermentation of S. commune by adding RP. Thus, RP was selected as the fermentation plant substrate of S. commune in the present work, and the objective was not only to optimize the fermentation conditions of SPG but also to obtain a fermentation product with outstanding antioxidant capacity.
2. Methods and materials
2.1 Materials and chemicals
Schizophyllum commune 5.43 was purchased from Guangdong Institute of Microbiology. RP was purchased from Good Wanjia Pharmacy Co. Ltd (Guangdong, China). Folin–Ciocalteu reagent, 2′,7′-dichlorodihydrofluorescein diacetate, gallic acid, chloranil, and catechin hydrate were purchased from Sigma Chemical Co. (St. Louis, MO, USA). All other chemicals and reagents were of analytical grade and purchased from local suppliers.
2.2 Culture and fermentation procedure
The seed medium (regular medium) for S. commune 5.43: 30.0 g glucose, 1.0 g KH2PO4, 0.5 g MgSO4·7H2O, and 3.0 g yeast extract were dissolved in 1000 mL deionized water at natural pH (about 6.0).6 The medium was then sterilized at 121 °C for 20 min.
RP-supplemented medium: a certain amount of RP powder was weighed, and the concentration of glucose, KH2PO4, MgSO4·7H2O, and yeast extract was the same as that for the seed medium. Hydrochloric acid (0.1 mol L−1) and sodium hydroxide (0.1 mol L−1) were used to adjust the pH, and the pH values were measured using a pH meter (Seven Excellence, Mettler Toledo, Switzerland). The medium was then sterilized at 121 °C for 20 min.
The S. commune strain was initially cultured in the seed medium as fermented seed at 28 °C for 3 d. The resulting seed culture was inoculated with a 5% inoculum size (v/v) in the fermentation medium and cultivation was carried out on a rotary shaker (ZAZY-78BN, ZHICHU, China) at 160 rpm and 28 °C. After 6 d fermentation, the fungus and supernatants were isolated by centrifugation, and then the fermented supernatants cultured from the RP-supplemented medium (SC-RP) or regular medium (SC) were collected for further study. After drying at 60 °C, the precipitate was screened through a 40-mesh sieve to remove the rest of the RP, and then dried at 60 °C to a constant weight. Finally, the biomass was measured using an electronic scale.
The crude exopolysaccharide (EPS) mixture obtained was extracted using the ethanol precipitation method described by Wang et al.,26 and then deproteinized to remove the proteins through the Sevag method.27 Subsequently, the total sugar content was estimated using the phenol-sulfuric acid method.28 The content of reducing sugar in the fermentation system was determined by the DNS method. The yield of EPS was equivalent to the total sugar content minus the reducing sugar content.29
2.3 Optimization for EPS production
2.3.1 Preliminary screening for medium components and culture conditions. Preliminary screening for the maximum EPS production was carried out by investigating the influence of initial pH (4.5, 5.0, 5.5, 6.0, 6.5 and 7.0), RP contents (6.0, 8.0, 10.0, 12.0, 14.0, and 16.0 g L−1), inoculum size (2.5%, 5%, 7.5%, 10%, 12.5% and 15.0%, v/v), and fermentation period (3.0, 4.0, 5.0, 6.0, 7.0, and 8.0 d). The experiments were conducted in 250 mL conical flasks with 100 mL medium. The yield of EPS was measured and calculated using the method described above. The results of the preliminary screening provided the required information for the response surface methodology (RSM).
2.3.2 Box–Behnken design and optimization process by RSM. Selecting an experimental design instead of a complete factorial design eliminates a large number of additional time-consuming laboratory experiments.30 RSM is an effective statistical technique that is useful for determining and modeling optimal conditions. The Box–Behnken design (BBD) is one of the most important RSM designs for determining the optimal condition. The Box–Behnken design provides spherical designs and requires the experiments to be performed at only three levels. This makes the design effective, thereby reducing the number of time-consuming and laborious experiments required to obtain sufficient data.The optimization of fermentation conditions, especially physical and chemical parameters, are paramount in the development of any fermentation process. A BBD with 3 factors and 3 levels was used to optimize the different process parameters for the optimization study. This design is suitable for the quadratic response surface and generates a second-order polynomial regression model. Initial pH (A), RP content (B), and inoculum size (C) were chosen as the independent variables. A (5.0, 5.5, and 6.0), B (10.0, 12.0, and 14.0 g L−1), and C (8%, 10%, and 12%) were determined to be appropriate levels for further testing. The complete design consisted of 17 combinations including 5 replicates at the central point. Each combination was repeated 3 times. Besides, the 3-D graphic plots obtained using software will illustrate the reciprocal interactions between each significant factor.
2.4 Isolation and purification of EPS
To investigate the influence of the addition or absence of RP in the medium on the EPS structure, SC-RP or SC were purified and isolated, respectively. Then the purified EPS from SC (EPS-1) or SC-RP (EPS-2) was stored in a dry state for further study.
2.5 Fourier transform infrared (FT-IR)
The FTIR spectra of the samples were obtained using a Nicolet iS20 FT-IR spectrometer (Thermo Fisher, Shanghai, China). The dried polysaccharides were mixed and ground with spectroscopic-grade potassium bromide (KBr) powder (1
:
100).31 Then the mixtures were pressed into KBr pellets, and FTIR determinations were conducted in the scanning range of 4000–400 cm−1 with a band resolution of 4 cm−1 and 8 cumulative scans. The FT-IR profile was obtained using the Origin 9.0 software.
2.6 Determination of total phenolics
The phenolic contents were determined using the modified Folin–Ciocalteu colorimetric method reported in a previous study.32 Briefly, the sample or gallic acid was mixed with Folin–Ciocalteu reagent for 10 min, after which sodium carbonate was added to the test tube and allowed to stand for 60 min in the dark after thorough mixing. The absorbance was detected at 760 nm and the result was expressed as milligrams of gallic acid equivalents per 100 g fresh weight sample (mg GAE/100 g, FW).
2.7 Determination of total flavonoids
The flavonoid contents were determined using the sodium borohydride/chloranil assay (SBC).33 The theory of the SBC is that flavonoids and vanillin can form a complex and appear orange under acidic conditions, which can be measured at 490 nm using a spectrophotometer (UV2600, SHIMADZU, Japan). Catechin was used as the standard. In brief, the sample (1 mL) was dried under nitrogen gas, and then the sample was reconstituted to 1 mL with THF/EtOH (1
:
1, v/v). Subsequently, 0.5 mL of 50 mM NaBH4 solution was added with 0.5 mL of 74.6 mM AlCl3 solution. The sample was shaken at room temperature for 30 min. Next, another 0.5 mL of 50 mM NaBH4 solution was added to the test tube before shaking for a while. After the addition of 2.0 mL of cold 0.8 M acetic acid solution, the sample was kept away from the light for 15 min on a shaker. Then, 1 mL of 20.0 mM chloranil was added, and the mixture was kept in an oil bath at 99 °C for 60 min. Finally, after the mixture was cooled to room temperature, methanol was added until all the samples reached 4.0 mL. Next, 1.0 mL of 16% vanillin solution in methanol and 2.0 mL HCl were added and well mixed, and the resulting mixture was kept in the dark for 15 min. The supernatant was obtained after centrifugation, and the absorbance was measured at 490 nm using a spectrophotometer. The flavonoid content was expressed as milligram catechin equivalents per 100 g in fresh weight (mg CE/100 g, FW).
2.8 Determination of total protein
The total protein of SC-RP and SC was tested using the BCA protein assay kit (Biyuntian Biotechnology Co., Ltd.). Briefly, 20 μL of standard or a sample from the supernatant of mixture preparation was added to 200 μL of BCA working reagent in a 96-well microplate. After 30 s agitation on a plate shaker, the plate was incubated at 37 °C for 30 min. Then, the plate was cooled to room temperature and the absorbance was measured at 562 nm using a microplate reader (SPARK 10M, TECAN, Switzerland).
2.9 Fraction of fermented supernatants cultured from RP-supplemented medium
SC-RP with different Mw was separated using a hollow fiber membrane (Mw cut-off of 100
000, 10
000, and 1000 Da) (Thermo Fisher Scientific, Shanghai, China), which was conducted under the following conditions: effective area of membrane used 50 cm2, temperature 25 °C, and operating pressure 1.5 bar (the former separation), temperature 40 °C, and operating pressure 1.7–2.0 bar (the latter separation). SC-RP with four Mw segments of >100
000, 10
000–100
000, 1000–10
000, and <1000 Da were denoted as SC-RP-1, SC-RP-2, SC-RP-3 and SC-RP-4, respectively.
2.10 Antioxidant activity assays
2.10.1 Hydroxyl radical scavenging activity. The hydroxyl radical-scavenging activity method was determined using the Fenton system, as described by Wang et al.34 with slight modifications. An FeSO4 solution (60 μL, 2 mM), salicylic acid–ethanol solution, (60 μL, 6 mM) and sample (20 μL) were mixed, and H2O2 (60 μL, 0.03% v/v) was used to initiate the reaction of the Fenton system. After incubation at 37 °C for 60 min, the absorbance of the resulting solution was measured at 510 nm using a microplate reader and distilled water as the blank control.The hydroxyl radical-scavenging activity was calculated as follows:
|
Scavenging activity (%) = [1 − (A1 − A2)/A0] × 100%.
| (1) |
where
A1 is the absorbance of the SC-RP or SC solution,
A2 is the absorbance of in distilled water instead of H
2O
2 (negative control), and
A0 is the absorbance of distilled water alone (blank control).
2.10.2 Assay of ABTS+ radical scavenging capacity. The antioxidant activity of SC-RP and SC was assay based on the radical scavenging capacity of ABTS + radical with slight modification, as reported by Li et al.35 The ABTS stock solution was produced by the reaction of 7 mM ABTS+ in water with 2.45 mM potassium persulfate, and then stored in the dark at room temperature for 12–16 h. The ABTS stock solution was then diluted with phosphoric acid buffer (pH = 7.4, 10 mM) to the absorbance of 0.700 ± 0.02 at 734 nm before use. Then, 10 μL of SC-RP or SC was added to 190 μL ABTS working solution and the mixture was incubated for 5 min in the dark at room temperature. The absorbance of the mixture solution was determined at 734 nm using a microplate reader, and Trolox was used as a positive control, where the antioxidant capacity is positively correlated with the TEAC value (Trolox equivalent antioxidant capacity).
2.10.3 Ferric reducing antioxidant power (FRAP) assay. The total antioxidant capacity of the samples was determined using a modification of the FRAP assay of Wootton-Beard et al.36 Briefly, FRAP reagent was prepared from 300 mM acetate and glacial acetic acid buffer (pH 3.6), 20 mM ferric chloride, and 10 mM 4,6-tripryridyl-s-triazine (TPTZ) made up in 40 mM HCl. All three solutions were mixed in a ratio of 10
:
1
:
1. The FRAP assay was performed by warming 25 μL of dH2O to 37 °C before adding 5 μL of SC-RP or SC and 170 μL of reagent and incubating at 37 °C for 10 min. The absorbance at 593 nm was determined relative to a reagent blank, which also incubated at 37 °C. Standard solutions of Trolox (0.25–20 nM) were used for the calibration curve and the results were expressed as FRAP value (μmol Trolox/100 g FW). The standard curve equation was: y = 0.0972x+0.0042 (R2 = 0.9997). The antioxidant capacity is positively correlated with the FRAP values.
2.10.4 Assay for oxygen radical absorption capacity (ORAC). The total antioxidant activities of SC-RP and SC were measured using the ORAC assay, as previously reported.37 SC-RP and SC were appropriately diluted with potassium phosphate buffer (pH 7.4). 20 μL of Trolox solution (6.25, 12.5, 25, 50, and 100 μmoL L−1) or sample solutions together with 200 μL of fluorescein were placed in a 96-well black microplate. The solutions were mixed automatically in a microplate reader and incubated at 37 °C for 10 min. Subsequently, 20 μL AAPH (119.4 mM) solution added and the absorbance recorded under the wavelengths of 485 nm (excitation) and 535 nm (emission) using a fluorescence spectrophotometer every 3.5 min, 35 times at 37 °C. The standard curve was obtained based on the area of the fluorescence quenched in different concentrations of Trolox standard solution. The ORAC values were expressed as μmol of Trolox equivalent per gram of sample (μmol Trolox/g FW).
2.11 Liquid chromatogram mass spectrometry (LC-MS) analysis
Briefly, 100 μL of SC-RP or SC was freeze-dried. Then, 500 μL of 80% methanol-aqueous solution was used to dissolve the freeze-dried products and centrifuged for 20 min (15000 rpm, 4 °C). The supernatant was diluted with a certain amount of supernatant with mass spectrometry-grade water to a methanol content of 53%. Then it was placed in a centrifuge tube at 15
000 g and centrifuged at 4 °C for 10 min. The supernatant was collected and injected into LC-MS/MS for analysis. The LC-MS/MS analysis was commissioned to Novogene Bioinformatics Technology Co., Ltd. (Beijing, China). LC-MS analyses were performed using a Vanquish UHPLC system (Thermo Fisher Scientific) coupled with a Q Exactive HF-X mass spectrometer (Thermo Fisher Scientific) in both positive and negative modes. The LC-MS/MS instrument was equipped with a C18 column (Hyperil gold C18 column, 100 mm × 2.1 mm, 1.9 μm). Each supernatant sample was analyzed as follows: (1) elution with a C18 column in positive-ion mode with a water/methanol gradient mobile phase containing 0.1% formic acid and (2) elution with a separate C18 column in negative-ion mode with a water/methanol gradient mobile phase containing 5 mM ammonium acetate, pH 9.0, with a flow rate of 0.2 mL min−1. The eluent was analyzed using a mass spectrometer (Thermo Fisher Scientific), and the scan range covered 100–1500 m/z. The raw data generated from the LC-MS analysis were processed using Compound Discoverer 3.0 (CD 3.0, Thermo Fisher) to perform peak alignment, peak picking, and quantitation for each metabolite. Subsequently, the peak intensities were normalized to the total spectral intensity. The normalized data were used to predict the molecular formula based on additive ions, molecular ion peaks, and fragment ions. Then, peaks were matched with the mzCloud and ChemSpider databases to obtain accurate qualitative and relative quantitative results.
2.12 Statistical analysis
The data obtained were analyzed by using Statistical Product IBM SPSS 25.0, and then recorded as the mean ± SD. Statistically significant differences among groups were determined by one-way analysis of variance (ANOVA) followed by LSD-tests when uniform variance of the result was identified or followed by Dunnett's-tests. p < 0.05 was considered statistically significant.
3. Results and discussion
3.1 RP does not affect the structure of schizophyllan
SC and SC-RP were further purified according to the process shown in Fig. 1 and the purified EPS was referred to as EPS-1 and EPS-2, respectively. Fig. 2 shows the FT-IR spectra of EPS-1 and EPS-2. Both EPS-1 and EPS-2 had the characteristic peak of polysaccharides, but there are some different absorption peaks between EPS-1 and EPS-2. According to a previous study, SPG is the main fermentation product of S. commune, which exhibited a broad band between 3600∼3200 cm−1, characteristic of the O–H stretching in hydrogen bonds. In this research, EPS-1 had broad band at 3316 cm−1 and EPS-2 had a wider and stronger absorption peak at 3348 cm−1, which means EPS-2 had a stronger O–H stretching vibration and this was derived from the intermolecular vibration. The weak characteristic peaks at 3000–2800 cm−1 are attributed to the C–H stretching vibration from the sugar ring.38 Next, two peaks were the characteristic absorption peaks of the saccharide compounds. Specifically, EPS-1 and EPS-2 had absorption peaks at 2810 cm−1 and 2884 cm−1, respectively, which are attributed to the sugar ring. In addition, AREF et al.39 also found that SPG had an absorption peak at 2887 cm−1. Both EPS-1 and EPS-2 had absorption peaks at 1631 cm−1 and this peak was related to the C–C stretching. The weak absorption band at 1400–1200 cm−1 corresponds to the out-of-plane bending vibration of C–H. In the present study, EPS-1 and EPS-2 showed an absorption peak at 1350 cm−1 and 1351 cm−1, respectively, indicating that they both contained the out-of-plane bending vibration of C–H. Meanwhile, the absorption band in the range of 1200 cm−1 to 1000 cm−1 was related to the C–O–H or the symmetrical stretching vibration of C–O–C from the sugar rings. Moreover, the absorption at 1081 cm−1 and 1109 cm−1 in each EPS indicates that these polysaccharides have pyranose rings, respectively.40 In addition, the bands at 891 cm−1 and 888 cm−1 were assigned to the C–H variable angle vibration of β-pyranoside, indicating that EPS-1 and EPS-2 are typical β-glucans, respectively. The above-mentioned bands indicate that EPS-1 and EPS-2 have β-glycosidic bands and pyranose rings, as expected for Schizophyllan.41 The EPS-1 and EPS-2 fractions were scanned at 190–700 nm and their UV spectra are depicted in Fig. S1,† where there were no characteristic absorption peaks at 260 nm or 280 nm, indicating that the protein and nucleic acid contents of the samples were relatively low. Thus, these results provide strong support that the addition of RP in a submerged medium does not affect the structure of SPG.
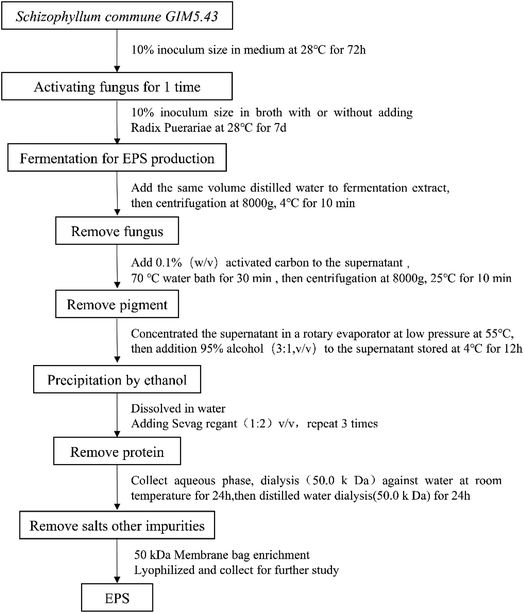 |
| Fig. 1 Schematic representation of the preparation of EPS from the fermented supernatants cultured from RP-supplemented medium (SC-RP) or regular medium (SC). | |
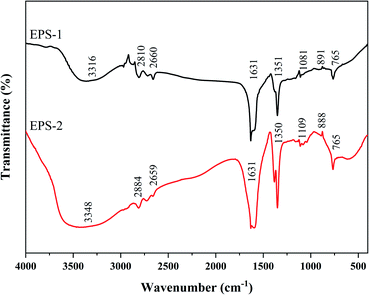 |
| Fig. 2 Infrared spectra of EPS purified from fermented supernatants cultured from regular medium (EPS-1) and RP-supplemented medium (EPS-2). | |
3.2 Exopolysaccharide yield of S. commune in liquid fermentation based on experimental findings
3.2.1 Effects of initial pH in culture medium. The effectiveness of the initial pH for the fermentation was studied by varying the pH from 4.5 to 7.0. The other parameters were set as follows: RP content 10.0 g L−1, fermentation period 6.0 d, and inoculum size 5.0% (v/v). The results obtained are displayed in Fig. 3(a). A high yield of SPG was observed at the pH value of 5.5 (7.88 ± 0.22 mg mL−1) for S. commune. Meanwhile, the biomass of S. commune also reached the maximum (15.29 ± 0.27 g L−1) at the pH value of 5.5, displaying that the growth of S. commune is closely related to the secretion of EPS in a weakly acidic environment. In addition, when the culture medium initial pH was at a higher level (pH = 7.0) or a lower level (pH = 4.5), the yield of SPG declined to 5.87 ± 0.23 mg mL−1 and 5.26 ± 0.09 mg mL−1, respectively. The biomass showed an identical tendency in this condition. The extreme condition negatively affected the growth of S. commune, and then the yield of SPG decreased. The results showed that the initial pH in the fermentation medium has a profound impact on the growth of S. commune and the yield of SPG. Hence, we set the initial pH as 5.5 for all subsequent experiments.
 |
| Fig. 3 Effect of each variable factor on the yield of EPS and biomass: (a) initial pH; (b) Radix Puerariae content; (c) inoculum size; and (d) fermentation period. Data is expressed as mean ± SD, n = 3. a Values with no letters in common are significantly different (p < 0.05). | |
3.2.2 Effect of RP contents. The RP contents in the culture medium play an important role in the fermentation system. The SPG yield and biomass of S. commune were studied at different contents of RP (6.0–16.0 g L−1). The results obtained are depicted in Fig. 3(b). Between 6.0 and 12.0 g L−1 of RP content, a rapid increase in EPS was observed from 4.86 ± 0.11 to 7.93 ± 0.12 mg mL−1. When the RP content increased from 12.0 to 16.0 g L−1, SPG declined from 7.93 ± 0.12 mg mL−1 to 7.47 ± 0.22 mg mL−1. In addition, we also found that the biomass of S. commune exhibited the same tendency as SPG, where the biomass reached 18.34 ± 0.41 g L−1 at the RP content of 12.0 g L−1. This result corroborates that a certain amount of RP can enhance the growth of the fungus, and then promotes SPG production. Therefore, the RP content was selected as 12.0 g L−1 to ensure a better SPG yield in the fermentation system.
3.2.3 Effect of inoculum size. The variation in the inoculum size of the culture system was studied at different concentrations (2.5–15.0%, v/v). As shown in Fig. 3(c), the yield of SPG increased with an inoculum size in the range of 2.5% to 10.0%. The yield of SPG reached 8.18 ± 0.10 mg mL−1 at the inoculum size of 10.0%. With the inoculum size increased further, there was a significant reduction in the SPG yield. In the case of the biomass, there was a slow increase with the inoculum size in the range of 2.5% to 7.5%. The results in Fig. 3(c) also show an insignificant (p < 0.05) growth in biomass when the inoculum size increased further. In the present study, RP was selected as a nutrition substance of the culture medium, and the results indicate that RP powder not only enhanced the SPG yield but also promoted the distribution of mycelium during fermentation, thus increasing the biomass. Thus, the final inoculation was selected as 10.0% to improve the production of SPG and biomass as much as possible.The culture time is an essential parameter for strain growth, which should be kept in balance with SPG production.42 In the present study, the effects of the culture time (3.0 d–8.0 d) on the yield of SPG was also studied, and the results are depicted in Fig. 3(d). An increase in the fermentation period seemed to have a significant effect (p < 0.05) on both the yield of SPG and biomass. It was shown that a fermentation period of 7.0 d was the optimized condition. Under this condition, the yield of SPG increased to 7.81 ± 0.21 mg mL−1. Simultaneously, there was a steady rise in the yield of SPG in a short period (3.0–6.0 d); however, with a further increase in this period, the yield of SPG began to decrease, and there was an insignificant (p > 0.05) difference between 6.0 d and 8.0 d. In the case of the biomass, it increased in the fermentation period range of 3.0 d to 7.0 d, which indicates that S. commune can grow well with an increase in the fermentation period. Therefore, we carried out the following experiments with a fermentation period of 7.0 d. Overall, these observations showed that an optimum value exists for each of the studied variables, which was subsequently determined by response surface methodology (RSM).
3.3 RSM model fitting and optimization
3.3.1 Model fitting. The ‘value of response’ (yield of SPG) at different experimental combinations for the various types of variables including their interactions is shown in Table 1. The analysis of variance (ANOVA) results presented in Table 2 shows a significant data combination, with model F-values of 20.97 and p-values less than 0.05 for SPG yield. This model implies that there is a significant factor and only a 0.01% chance that an F-value this large can occur due to noise. The small p-values obtained indicate that the quadratic model is statistically significant to track the design domain and can be used to predict the yield of SPG.
Table 1 The matrix of the Box–Behnken design and corresponding yields of EPS
Run |
Initial pH A |
Radix Puerariae (g L−1) B |
Inoculum size (%) C |
Exopolysaccharides (g L−1) |
1 |
−1 (5.0) |
0 (12) |
−1 (8) |
7.63 |
2 |
−1 |
−1 (10) |
0 (10) |
6.99 |
3 |
0 (5.5) |
1 (14) |
−1 |
7.64 |
4 |
−1 |
0 |
1 (12) |
7.86 |
5 |
0 |
0 |
0 |
8.33 |
6 |
0 |
−1 |
1 |
7.23 |
7 |
0 |
−1 |
−1 |
7.99 |
8 |
0 |
0 |
0 |
8.51 |
9 |
0 |
1 |
1 |
8.13 |
10 |
0 |
0 |
0 |
8.29 |
11 |
1 (6.0) |
0 |
1 |
7.26 |
12 |
1 |
1 |
0 |
7.42 |
13 |
1 |
−1 |
0 |
7.20 |
14 |
−1 |
1 |
0 |
7.89 |
15 |
0 |
0 |
0 |
8.46 |
16 |
1 |
0 |
−1 |
7.35 |
17 |
0 |
0 |
0 |
8.38 |
Table 2 ANOVA of the fitted polynomial quadratic model
Source |
Sum of squares |
df |
Mean square |
F value |
p-Valuea |
Significanceb |
Source: ANOVA using design-expert 8.0.6. *Significant at 0.01 < p < 0.05, **significant at p < 0.01, and ***significant at p < 0.001. |
Model |
3.845 |
9 |
0.427 |
20.97 |
0.0003 |
*** |
A |
0.162 |
1 |
0.162 |
7.97 |
0.0256 |
* |
B |
0.349 |
1 |
0.349 |
17.11 |
0.0044 |
** |
C |
2.112 × 10−3 |
1 |
2.112 × 10−3 |
0.10 |
0.7568 |
— |
AB |
0.116 |
1 |
0.116 |
5.67 |
0.0487 |
* |
AC |
0.026 |
1 |
0.026 |
1.26 |
0.2993 |
— |
BC |
0.391 |
1 |
0.391 |
19.18 |
0.0032 |
*** |
A2 |
1.622 |
1 |
1.622 |
79.65 |
<0.0001 |
*** |
B2 |
0.668 |
1 |
0.668 |
32.78 |
0.0007 |
*** |
C2 |
0.259 |
1 |
0.259 |
12.74 |
0.0091 |
*** |
Residual |
0.143 |
7 |
0.020 |
— |
— |
— |
Lack of fit |
0.110 |
3 |
0.037 |
4.44 |
0.0919 |
— |
Pure error |
0.033 |
4 |
8.230 × 10−3 |
— |
— |
— |
Cor total |
3.988 |
16 |
— |
— |
— |
— |
R2 |
0.9642 |
|
Adj R2 |
0.9183 |
|
C.V.% = 1.83 |
The model reduction was applied to eliminate some of the noisy terms that are insignificant, and thus improve the model performance. The experimental data were analyzed using the multiple regression analysis, and the response variables and the test variables were related using the following second-order polynomial equation:
|
Yield of SPG (mg mL−1) = −93.109 + 29.868A + 2.648B + 0.736C − 0.170AB − 0.080AC + 0.078BC − 2.483A2 − 0.099B2 − 0.062C2.
| (2) |
where A: initial pH; B: RP content (g L
−1); and C: inoculum size (%).
The coefficient of determination (R2) and the adjusted determination coefficient (Radj2) were 0.9642 and 0.9183, respectively. These results signify that the fitted model explained 96.42% of the entire variability. Besides, the model had a good fit with the experimental data and the theoretical values of the yield of SPG. This is better described by the scatter plots in Fig. 4(a) and (b), which show a significant correlation and adequate estimation between the actual and predicted variables. Additionally, the lack of fit was not significant (p > 0.05), indicating that the model is suitable to represent that experimental data. In addition, the results support the good fit. There was a relatively low value of the coefficient of variation (C.V. = 1.83%), reflecting the high reproducibility and reliability of the experimental values.
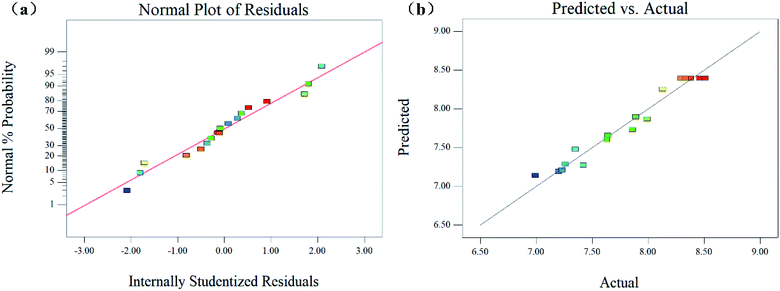 |
| Fig. 4 Normal probability plot of residuals of the predictable values (a), and the predicted and actual response slope (b) of EPS yield from Schizophyllum commune. | |
3.3.2 Response surface analysis. As displayed in Table 2, the linear coefficients (A, B, and C), cross-product coefficients (AB and BC), and quadratic term coefficients (A2, B2, and C2) were significant (p < 0.05), whereas the other terms coefficients were not significant (p > 0.05). The F values confirmed that the order of factors influencing the yield of SPG was initial pH > RP contents > inoculum size, and the order of the interaction effects was BC > AB > AC.To visualize the effects of the independent variables on the response, 3-dimensional response surface plots of the model were prepared to quantify the optimal production to obtain the optimum yield of SPG. The 3-dimensional graphs of the RSM plots are in strong agreement with the experimental findings. Besides, the steepness of the response surface indicated the degree of interaction influences of the 2 experimental variables. The effect between the initial pH, RP content, and inoculum size on the yield of SPG is shown in Fig. 5. Fig. 5(a) and (b) show the effect of the interaction between initial pH and RP content on the production of SPG. It can be observed that a low-level pH value at 5.5 favored the production of SPG. Below this pH level, increasing the RP content from 10.0 g L−1 to 13 g L−1 led to a remarkable improvement in the SPG production. Above 13.0 g L−1, the production decreased slowly. Likewise, the effect of the interaction between initial pH and inoculation size on the SPG production is shown in Fig. 5(c) and (d). It can be observed that the SPG yield increased steeply at low inoculation (8.0–10.0%), and then declined with a further rise in inoculation, as explained earlier in the preliminary experiments. In addition, Fig. 5(e) and (f) show the interaction between the RP content and inoculation. There was a profound interaction effect when the RP content ranged from 12.0 to 14.0 g L−1 and inoculation was around 9.0% to 11.0%. Meanwhile, the results also indicated that the combination of RP contents and inoculum size favored high production efficiency. Thus, these results imply that the initial pH, RP content, and inoculum size have a critical impact in the production of SPG. All the observations from the response surface are in agreement with the preliminary results discussed earlier.
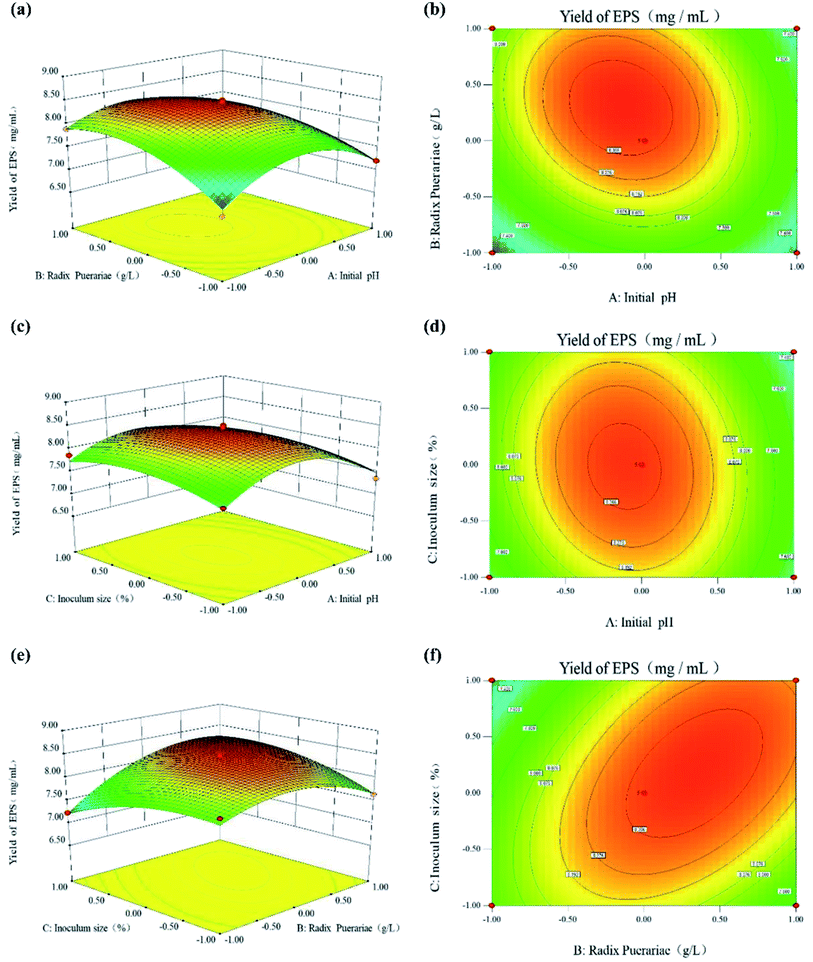 |
| Fig. 5 Three-dimensional plots and corresponding contour plots of the three variables (A: initial pH; B: Radix Puerariae contents; and C: inoculum size) on the response of EPS yield. | |
3.3.3 Model verification. The optimal conditions for maximizing the yield of SPG were predicted from the model as follows: initial pH: 5.41, RP content: 12.80 g L−1, and inoculum size 10.62%. The predicted point was experimentally verified under the actual optimum conditions as follows: initial pH: 5.40, RP content: 12.80 g L−1, and inoculum size 10.00%. Under these conditions, the yield of SPG was 8.41 ± 0.12 mg mL−1, without a significant difference from the predicted value of 8.45 mg mL−1 (p > 0.05). These results show that the model used in this study is valid. In addition, the concentration of SPG in SC was also studied based on the optimal fermentation conditions, and the results are shown in Table 3. It can be observed that SPG yield is 6.76 ± 0.52 mg mL−1, indicating that the addition of RP can significantly (p < 0.05) increase the yield of SPG, and the production increased by 1.24-fold.
3.4 The bioactive ingredients of fermented supernatants
3.4.1 SC-RP and SC contain a certain amount of total proteins and SPG. The fermentation system is a complex process, and the culture products normally contain an abundance of biologically active ingredients.43 Thus, to facilitate the analysis of the active substances in the fermentation products, this study discussed the differences between the active ingredients using the same concentration of SPG. SC-RP was appropriately diluted to achieve the SPG concentration of 6.76 ± 0.47 mg mL−1, the same as SC. Then, a full analysis of the active ingredients of SC or SC-RP was conducted, as shown in Table 3. The results show that the addition of RP significantly enhanced the total protein contents of the fermented supernatants. Compared with that of SC of 1.05 ± 0.03 mg mL−1, the total proteins of SC-RP increased to 4.66 ± 0.05 mg mL−1, which represents an increase in protein production by 4.44-fold. These results indicate that RP supplementation significantly improved the protein contents of the liquid culture of S. commune, which may be due to the promoting effect of RP to S. commune fermentation and the introduction of protein from RP. Additionally, the specific components of these proteins need further studies to prove their existence.
Table 3 The composition from the fermented supernatants cultured from RP-supplemented medium (SC-RP) or regular medium (SC)
Group |
Exopolysaccharides (mg mL−1) |
Total protein (mg mL−1) |
Total phenolics (mg GAE/100 g, FW) |
Total flavonoids (mg CE/100 g, FW) |
Values with no letters in common are significantly different (p < 0.05). |
SC-RP |
6.76 ± 0.47 |
4.66 ± 0.05a |
3731.56 ± 54.07a |
27.97 ± 0.41a |
SC |
6.76 ± 0.52a |
1.05 ± 0.03b |
288.35 ± 27.87b |
8.47 ± 0.25b |
3.4.2 The introduction of RP makes fermented supernatants of S. commune rich in total phenolics and flavonoids. The contents of total phenolics for the fermented products SC and SC-RP are shown in Table 3. Compared with the total phenolics of SC (288.35 ± 27.87 mg GAE/100 g, FW), the total phenolics of SC-RP increased to 3731.56 ± 54.07 mg GAE/100 g, FW, which increased by 11.94-folds. Besides, the total flavonoid contents in SC-RP and SC were 27.97 ± 0.41 mg CE/100g, FW and 8.47 ± 0.25 mg GAE/100g, FW, respectively. Also, the total flavonoids contents of SC-RP increased to 3.30-fold by adding RP. Numerous reports have indicated that plant or fruit-derived extracts such as Rhodiola, orange, and apple peel contains a considerable amount of phenolics and flavonoids.32,44–46 According to relevant research, RP is also rich in phenolics and flavonoids.47 Comparing the phenolics (460.4 ± 3.3 mg GAE/100 g, FW) and flavonoids (257.4 ± 16.2 mg CE/100 g, FW) to that in blueberry,48 SC-RP showed a much higher capacity to enrich biologically active ingredients. Thus, the results may be closely related to the characteristics of RP. Furthermore, the complex fermentation system may also play a crucial role in the present study. There were abundant enzymes including lignocellulase, xylanase, and cellulose during the fermentation of S. commune.49 A previous study illustrated that S. commune can biotransform sophoricoside in Fructus sophorae to synthesize new active substances with outstanding toxicity toward MCF-7 cells, thus revealing that S. commune has the potential to enrich the active ingredients of the fermented products and enhance the antioxidant, anti-aging and biological properties of phytochemicals in fermentation substrates.25 Therefore, the total phenolics and flavonoids of SC-RP may be derived from the RP components from the culture or the biological ingredients of RP, which stem from the transformation through the submerged culture system.To further investigate the distribution of total phenolics and flavonoids in the different molecular components of SC-RP, the content of total phenolics was determined after ultrafiltration. The contribution of SC-RP-1, SC-RP-2, SC-RP-3, and SC-RP-4 to the total phenolics of SC-RP ranged from 6.30% to 60.01%, and the highest total phenolic extract was SC-RP-1 (2239.25 ± 32.45 mg GAE/100 g, FW). In addition, SC-RP-1 to SC-RP-4 contributed the total flavonoids of SC-RP in the range of 2.83% to 71.92% (Table 4), and SC-RP-1 also exhibited the highest content of total flavonoids. Interestingly, we observed that the contents of phenolics, flavonoids, and SPG were closely related and ultrafiltration could not thoroughly separate them. SC-RP-1 had the richest contents of total phenolics and total flavonoids, and their contents were proportional to the amount of SPG. According to a previous study, Biological macromolecules and small molecules with specific biological activity can be combined by electrostatic, hydrophobic, hydrogen bonding, and other forces. Also, this combination has the characteristics of high specificity and reversibility, such as polysaccharides, proteins, and phenolics.50 Besides, studies have shown that the stability of the water solubility of polyphenol compounds such as quercetin, resveratrol, and ferulic acid embedded in polysaccharides is significantly improved.51–53 Therefore, these results indicate that the submerged culture promoted the effective combination of SPG, phenolics, flavonoids, and protein in a stable system, and it is difficult to separate this system by physical function.
Table 4 The compositions with different molecular weights from the fermented supernatants cultured from RP-supplemented medium (SC-RP) or regular medium (SC)
Group |
Exopolysaccharides (mg mL−1) |
Total protein (mg mL−1) |
Total phenolics (mg GAE/100 g, FW) |
Total flavonoids (mg CE/100 g, FW) |
Values with no letters in common are significantly different (p < 0.05). Values in parentheses indicate percentage contribution to the total. |
SC-RP |
6.76 ± 0.47a |
4.66 ± 0.05a |
3731.56 ± 54.07a |
27.97 ± 0.41a |
SC-RP-1 |
5.91 ± 0.38 (87.39) |
3.71 ± 0.04b (79.58) |
2239.25 ± 32.45b (60.01) |
20.12 ± 0.29b (71.92) |
SC-RP-2 |
0.26 ± 0.02c (3.87) |
0.36 ± 0.01c (7.79) |
639.70 ± 9.27c (17.14) |
4.12 ± 0.06c (14.73) |
SC-RP-3 |
0.37 ± 0.02c (5.32) |
0.33 ± 0.00c (7.05) |
617.68 ± 8.95c,d (16.55) |
2.94 ± 0.04d (10.52) |
SC-RP-4 |
0.23 ± 0.01c (3.42) |
0.26 ± 0.01d (5.07) |
234.93 ± 3.40e (6.30) |
0.79 ± 0.01e (2.83) |
3.5 RP enhanced antioxidant capacity of fermented supernatants of S. commune
RP contains active ingredients such as phenolics and flavonoids, which are the main source of biological activities such as antioxidant, anti-aging, and pharmacological activities. Furthermore, as one of the earliest fungal polysaccharides used in clinical cancer treatment,54,55 SPG also has excellent moisturizing activity, antioxidant capacity, and anti-inflammatory properties. According to the above-mentioned results, the addition of RP can increase the production of SPG, while increasing the content of active ingredients like phenolics and flavonoids. Moreover, numerous studies have shown that fermentation has the ability to increase the content of bioactive phenolic compounds in plants, thus enhancing their antioxidant activity.56–58 Hence, whether RP can affect the antioxidant activity of the culture from submerged fermentation to improve the biological activity of SC-RP was determined in the present study. In addition, different compounds may act through multiple mechanisms against oxidizing agents.59 Therefore, one isolated method can hardly completely evaluate the antioxidant capacity of complex samples. In this research, several methods for the determination of antioxidant activity were used to reflect the antioxidant capacity of SC-RP or SC.
Normally, free radicals regulate intercellular signaling and cell growth, inhibiting viruses and bacteria. However, too many free radicals in the body can damage cells, tissues, and organs, and even induce many disorders and physical diseases, such as inflammation, cancer, aging, and radiation damage. Hence, polysaccharide and phenolic compounds have been considered as the main antioxidants from plant substrates because of their effective elimination of excess free radicals in the body.28 The antioxidant activities in vitro of total SC and SC-RP were studied with hydroxyl radical scavenging in the Fenton system, ABTS + radical scavenging, FRAP, and ORAC experiments. As a common active oxygen generator, hydroxyl radicals can react with biomolecules in living cells and cause severe damage.60 Fig. 6(a) depicts that SC and SC-RP exhibited hydroxyl radical scavenging activity. The scavenging rate of SC-RP on hydroxyl radicals reached 62.23% ± 4.52% and showed a significant improvement compared to SC, which only scavenged 14.74% ± 0.79% hydroxyl radicals. The results demonstrate that SC-RP has outstanding capacity to scavenge hydroxyl radicals.
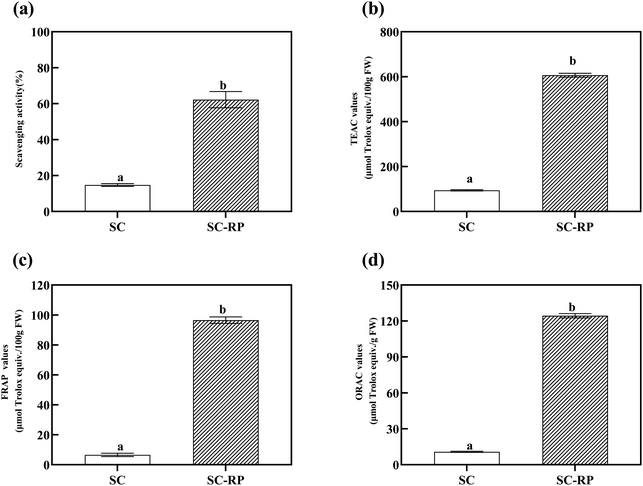 |
| Fig. 6 In vitro antioxidant capability of the fermented supernatants cultured from RP-supplemented medium (SC-RP) or regular medium (SC). (a: Hydroxyl radical scavenging activities; b: ABTS radical scavenging activities; c: FRAP values; and d: ORAC values). Data is expressed as mean ± SD, n = 3. a Values with no letters in common are significantly different (p < 0.05). | |
ABTS + radical scavenging assays are usually used to evaluate the total antioxidant capacity of natural products. In our previous study, both SC and SC-RP had the ability to scavenging free radicals of ABTS+, and we choose Trolox as a positive control. As shown in Fig. 6(b), the TEAC value of SC-RP was 606.46 ± 8.65 μmol Trolox/100 g, FW, with a significant difference to SC (p < 0.001). Compared with the hydroxyl radical scavenging assay, the results indicated that SC or SC-RP exerted a stronger ability to scavenge ABTS + radical, which may be due to the different properties of the radical model.
The total antioxidant activities were also measured using FRAP and ORAC assays. In the case of ORAC, its higher antioxidant activity is related to its better capacity to quench AAPH radicals, whereas in the case of FRAP, lower FRAP values indicate a small capacity to reduce ferric ions.61 The antioxidant capacity of SC and SC-RP as determined by the FRAP and ORAC assay is summarized in Fig. 6(c) and (d), respectively. Both the ORAC value and the FRAP value indicated that the antioxidant activity of SC-RP significantly increased compared to that of SC. The ORAC values and FRAP values of SC-RP both increased by 11.56-fold and 14.69-fold, respectively. Consequently, the results of the in vitro antioxidant activity analysis suggest that SC-RP possessed powerful capacity in the scavenging of hydroxyls and ABTS + free radicals. Furthermore, the FRAP values and ORAC values indicate that SC-RP exhibited its antioxidant activity via multiple mechanisms. A previous study showed that Schizophyllum commune glucan possessed an ORAC value of only 0.148 μmol Trolox/g, which is much lower compared to that of SC-RP (124.29 ± 1.80 μmol Trolox/g, FW).62 Moreover, the ORAC value of SC-RP was 1.84-fold that of blueberry extracts (67.47 ± 1.41 μmol Trolox/g, FW),49 explaining its excellent antioxidant capacity. Therefore, this shows that SC-RP had a great potential to be developed as a natural antioxidant.
Overall, we introduced the content of bioactive ingredients in SC and SC-RP, as well as the content of SC-RP ingredients with different molecular weights by ultrafiltration. Next, we also investigated the antioxidant activity of SC-RP with different molecular weights, as depicted in Fig. 7. The free hydroxyl radical scavenging activity of SC-RP with molecular weights is summarized in Fig. 8(a), where its free hydroxyl radical scavenging ranged from 43.47% ± 0.95% to 72.78% ± 0.47%. SC-RP-1 showed the poorest antioxidant capacity in this Fenton system. However, SC-RP-2, SC-RP-3, and SC-RP-4 showed excellent free hydroxyl radical scavenging. In the case of the antioxidant system of ABTS + radicals (Fig. 8(b)), SC-RP displayed the highest antioxidant activity and the TEAC value of 606.46 ± 8.65 μmol Trolox/100 g FW. The TEAC values of SC-RP-1 to SC-RP-4 ranged from 350.92 ± 3.85 to 547.35 ± 3.54 μmol Trolox/100 g FW, and the TEAC values in descending order were SC-RP-1, SC-RP-2, SC-RP-3, and SC-RP-4, which is similar to the pattern of the total phenolic contents and SPG contents.
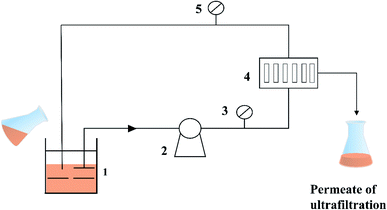 |
| Fig. 7 Schematic diagram of the ultrafiltration membrane separation process. (1-Beaker; 2-circulating pump; 3-pressure gage; 4-membrane module; and 5-pressure gage.) | |
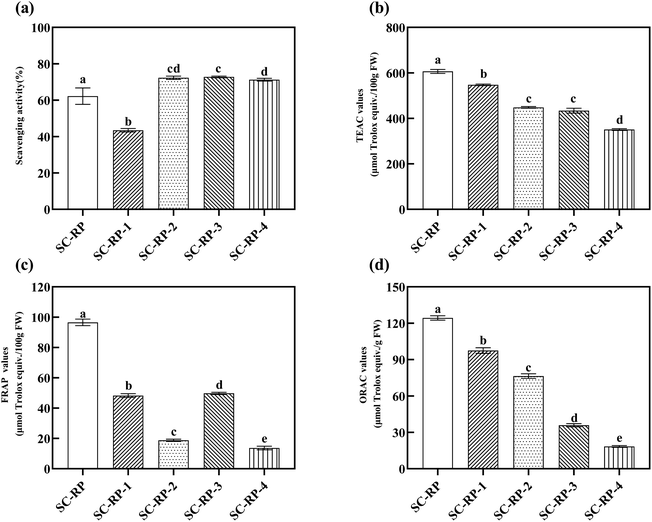 |
| Fig. 8 In vitro antioxidant capacity of different molecular weights of the fermented supernatants cultured from RP-supplemented medium (SC-RP) or regular medium (SC). (a: Hydroxyl radical scavenging activities; b: ABTS radical scavenging activities; c: FRAP values; and d: ORAC values). Data is expressed as mean ± SD, n = 3. a Values with no letters in common are significantly different (p < 0.05). | |
The FRAP and ORAC assays are considered to be effective antioxidant activity assays given that they utilize the biologically relevant free radicals, peroxyl radicals.63 The values of FRAP and ORAC of SC-RP-1 to SC-RP-4 are shown in Fig. 8(c) and (d), respectively. In the antioxidant system of FRAP, the results showed that the FRAP values of SC-RP-1 to SC-RP-4 were lower than SC-RP, in which the FRAP values of SC-RP were higher at 96.51 ± 2.15 μmol Trolox/100 g FW. The FRAP values of SC-RP-1 to SC-RP-4 ranged from 11.75 ± 0.51 to 49.72 ± 0.70 μmol Trolox/100 g FW, and that of SC-RP-3, SC-RP-1, SC-RP-4, and SC-RP-2 decreased sequentially. In addition, the ORAC values gradually decreased from SC-RP-1, SC-RP-2, SC-RP-3, and SC-RP-4, where that of SC-RP-1 to SC-RP-4 ranged from 97.48 ± 2.34 to18.40 ± 0.73 μmol Trolox/g, FW. Thus, the ORAC values of SC-RP showed the same tendency as the ABTS free radical scavenging system.
Different compounds may act through different mechanisms against oxidizing agents, and thus we analyzed the correlation between the components of SC-RP and antioxidant activity, and the results are shown in Table 5. Pearson's correlation analysis was conducted to analyze the relationship among the antioxidant hydroxyl radical activities (Fenton system), TEAC values, FRAP values, ORAC values, and active ingredients contents including total phenolics, total flavonoids, SPG, and total protein. As shown in Table 5, there were significant correlations between ABTS + free radical scavenging activity and total phenolics (r = 0.954, p = 0.012), total flavonoids (r = 0.960, p = 0.010), SPG (r = 0.923, p = 0.026), and total protein (r = 0.933, p = 0.021). The FRAP values and total phenolic had a brilliant correlation (r = 0.904, p = 0.035). Besides, the ORAC values showed a significant correlation with the total phenolic (r = 0.904, p = 0.035) and total flavonoids (r = 0.915, p = 0.029), respectively. However, the hydroxyl radical activities and the active ingredients showed no significant correlations (p > 0.05). Thus, the antioxidant activity determined by the ABTS, FRAP, ORAC assay shows a positive correlation with the bioactive contents of SC-RP. Further work can be conducted in cell or animal antioxidant models for a comprehensive understanding of the antioxidant activity of SC-RP in vivo.
Table 5 Correlation of total phenolic, total flavonoids, exopolysaccharides, total protein, and antioxidant activity
|
Total phenolics |
Total flavonoids |
SPG |
Total protein |
Fenton system |
TEAC values |
FRAP values |
ORAC values |
Total phenolic |
1.000 |
0.990 |
0.955 |
0.973 |
−0.589 |
0.954 |
0.904 |
0.904 |
Total flavonoids |
|
1.000 |
0.985 |
0.994 |
−0.694 |
0.960 |
0.854 |
0.915 |
SPG |
|
|
1.000 |
0.997 |
−0.797 |
0.923 |
0.794 |
0.863 |
Total protein |
|
|
|
1.000 |
−0.751 |
0.933 |
0.821 |
0.879 |
Fenton system |
|
|
|
|
1.000 |
−0.640 |
−0.342 |
−0.591 |
TEAC values |
|
|
|
|
|
1.000 |
0.860 |
0.959 |
FRAP values |
|
|
|
|
|
|
1.000 |
0.727 |
ORAC values |
|
|
|
|
|
|
|
1.000 |
3.6 The compositions of SC-RP antioxidant fractions
LC/MS was used to reveal the compositions of SC-RP and SC, and 25 different components were detected in SC-RP compared to SC, including phenolics (24.00%), acids (28.00%), flavonoids (16.00%), and other types (32.00%) (Table 6). According to correlation analysis in the present study, puerarin, resveratrol, genistin, isorhapontigenin, genistein and coumestrol were the main antioxidant components in SC-RP. Genistin, genistein, puerarin, and coumestrol were the common bioactive substances of Radix Puerariae. A previous study showed that fermentation can enhance the antioxidant capacity, the total phenolics of RP, and red yeast rice by Monascus purpureus.22 Wang et al. reported that solid-state fermentation with Lactobacillus plantarum enhanced the total phenolic contents and antioxidant activities of rice and wheat bran.64 Thus, these results reveal that the addition of RP not only contributes bioactive ingredients to the fermentation system but also enhances the antioxidant ability of the fermented products. Furthermore, these results indicate that the components of SC-RP contain resveratrol, which was not detected in the fermentation medium containing RP. This may be due to the enzymes of the S. commune fermentation system such as lignocellulase, xylanase, and cellulose transforming some bioactive ingredients of RP to resveratrol or other compounds. These results illustrate that S. commune is possibly a favorable biotransformation platform for introducing bioactive substances from plants and generating some new antioxidant components in the fermentation product. In addition, the components with good antioxidant properties, such as 3-hydroxy-3-methylpentanedioic acid, pyrrole-2-carboxylic acid, sorbic acid, 4-hydroxy-5, 8-dimethylquinoline-3-carboxylic acid, kynurenic acid, and glycitin were found in the SC-RP fermentation broth. SC-RP also contained some flavonoids such as esculetin, kaempferol-3-O-β-glucopyranosyl-7-O-α-rhamnopyranoside, turmerone, and diosmetin, and there is no report to date that esculetin exists in Radix Puerariae. These components endowed a variety of antioxidant properties to SC-RP. However, the contribution and detail mechanism of these complex components on its antioxidant activity need further investigation in cell or animal antioxidant models in vivo.
Table 6 Characterization of the bioactive component constituents of SC-RP by UPLC-MS
No. |
Rt (min) |
[M–H]− (m/z) |
Formulate |
Identification |
Peak area |
Phenolic |
1 |
8.001 |
432.1164 |
C21H20O10 |
Puerarin |
75 816 621 103 ± 2 099 008 708 |
2 |
8.638 |
229.0853 |
C14H12O3 |
Resveratrol |
33 816 995 ± 6 065 904 |
3 |
9.309 |
443.1119 |
C21H20O10 |
Genistin |
273 146 315 ± 38 738 670 |
4 |
10.335 |
259.096 |
C15H14O4 |
Isorhapontigenin |
134 255 340 ± 23 833 827 |
5 |
10.994 |
271.0595 |
C15H10O5 |
Genistein |
342 470 527 ± 15 076 155 |
6 |
11.223 |
269.0438 |
C15H8O5 |
Coumestrol |
33 196 125 ± 5 999 602 |
![[thin space (1/6-em)]](https://www.rsc.org/images/entities/char_2009.gif) |
Acids |
1 |
1.397 |
180.0863 |
C6H10O5 |
3-Hydroxy-3-methylpentanedioic acid |
1 143 148 873 ± 1 672 163 |
2 |
2.355 |
112.0391 |
C5H5NO2 |
Pyrrole-2-carboxylic acid |
149 882 432 ± 25 741 286 |
3 |
6.709 |
113.0596 |
C6H8O2 |
Sorbic acid |
24 782 657 ± 1 109 412 |
4 |
6.779 |
155.0337 |
C7H6O4 |
2,4-Dihydroxybenzoic acid |
42 992 578 ± 1 386 845 |
5 |
7.704 |
218.0806 |
C12H11NO3 |
4-Hydroxy-5,8-dimethylquinoline-3-carboxylic acid |
13 659 482 ± 1 415 355 |
6 |
7.882 |
190.0497 |
C10H7NO3 |
Kynurenic acid |
56 828 333 ± 867 472 |
7 |
8.66 |
447.1273 |
C22H22O10 |
Glycitin |
17 703 891 ± 1 189 855 |
![[thin space (1/6-em)]](https://www.rsc.org/images/entities/char_2009.gif) |
Flavonoids |
1 |
6.462 |
179.0336 |
C9H6O4 |
Esculetin |
77 573 412 ± 3 548 581 |
2 |
7.506 |
595.165 |
C27H30O15 |
Kaempferol-3-O-β-glucopyranosyl-7-O-α-rhamnopyranoside |
193 217 219 ± 31 403 519 |
3 |
8.118 |
217.1582 |
C15H20O |
(+)-ar-Turmerone |
21 340 376 ± 3 945 005 |
4 |
8.27 |
301.0699 |
C16H12O6 |
Diosmetin |
261 211 160 ± 31 749 776 |
![[thin space (1/6-em)]](https://www.rsc.org/images/entities/char_2009.gif) |
Others |
1 |
1.548 |
186.1123 |
C9H15NO3 |
Ecgonine |
407 653 751 ± 3 174 877 |
2 |
7.344 |
130.0648 |
C9H7N |
Isoquinoline |
35 321 772 ± 17 525 537 |
3 |
7.899 |
579.1685 |
C27H30O14 |
Rhoifolin |
5 514 305 219 ± 240 509 018 |
4 |
8.478 |
219.174 |
C15H22O |
Nootkatone |
39 854 309 ± 3 595 485 |
5 |
9.129 |
239.0696 |
C15H10O3 |
7-Hydroxy-3-phenyl-4H-chromen-4-one |
63 159 987 ± 7 630 202 |
6 |
10.042 |
199.0863 |
C12H10N2O |
4-Nitrosodiphenylamine |
31 385 625 ± 4 596 871 |
7 |
10.395 |
153.1272 |
C10H16O |
Citral |
132 843 854 ± 17 525 537 |
8 |
13.483 |
337.1061 |
C20H16O5 |
Psoralidin |
27 363 092 ± 1 058 9130 |
4. Conclusions
In the present study, the FT-IR analysis of EPS-1 and EPS-2 showed that they were no differences and the addition of RP did not affect the structure of SPG. The yield of SPG was significantly improved from 6.76 ± 0.52 to 8.41 ± 0.12 mg mL−1 through a submerged culture under the optimal conditions (initial pH: 5.40, RP content: 12.80 g L−1 and inoculum size 10.00%). In addition, the antioxidant activities of SC-RP and SC were evaluated using four different antioxidant mechanisms, where SC-RP displayed stronger antioxidant activity. Also, the antioxidant capacity of different Mw of SC-RP was further studied. Interestingly, a significant correlation among the phenolic compounds, flavonoids, and antioxidant activity of SC-RP was found in the present study. Furthermore, more than 25 distinctive bioactive ingredients were detected in SC-RP compared to that in SC, which may play key factors in its antioxidant activities. The results indicated that RP could enhance the yield of SPG and improve the antioxidant activity of fermented products in S. commune. In general, SC-RP has profound potential in the development of functional foods and bioactive antioxidants in the cosmetics industry. Therefore, further studies on the mechanisms of action of the antioxidants and antiaging activities in vivo of SC-RP will be needed in the future.
Abbreviations
EPS | Exopolysaccharides |
RSM | Response surface methodology |
RP | Radix Puerariae |
SC-RP | Fermented supernatants cultured from RP-supplemented medium |
SC | Fermented supernatants cultured from regular medium |
SPG | Schizophyllan |
S. commune | Schizophyllum commune |
BBD | Box–Behnken design |
FT-IR | Fourier transform infrared |
KBr | Potassium bromide |
ABTS+ | 2,2′-Azino-di-(3-ethylbenzothiazoline)-6-sulfonic acid |
FRAP | Ferric reducing antioxidant power |
ORAC | Oxygen radical absorption capacity |
LC-MS | Liquid chromatogram mass spectrometry |
ANOVA | One-way analysis of variance |
Author contributions
Yong-fei Deng: responsible for the design, experiment, and draft of the manuscript. Qian Huang: FTIR measurement analysis and assisted testing; Lu Hu: analysis summary and discussion; Tao Liu: assisted testing; Bi-sheng Zheng: summary of the data; Deng-jun Lu & Chao-wan Guo: supervision. Lin Zhou: organized, supervised, and guided the whole work.
Conflicts of interest
The authors declare that they have no known competing financial interests or personal relationships that could have appeared to influence the work reported in this paper.
Acknowledgements
The authors are grateful for the financial support from the Guangdong Pharmaceutical University Research Project (43255098) and Guangzhou Science and Technology Plan Project Fund (202103000055).
References
- A. K. Rai, A. Pandey and D. Sahoo, Trends Food Sci. Technol., 2019, 83, 129–137, DOI:10.1016/j.tifs.2018.11.016.
- D. K. Meena, P. Das, S. Kumar, S. C. Mandal, A. K. Prusty, S. K. Singh, M. S. Akhtar, B. K. Behera, K. Kumar, A. K. Pal and S. C. Mukherjee, Fish Physiol. Biochem., 2013, 39, 431–457, DOI:10.1007/s10695-012-9710-5.
- N. A. Mohd Fauziee, L. S. Chang, W. A. Wan Mustapha, A. R. Md Nor and S. J. Lim, Int. J. Biol. Macromol., 2021, 167, 1135–1145, DOI:10.1016/j.ijbiomac.2020.11.067.
- N. Sutivisedsak, T. D. Leathers, K. M. Bischoff, M. S. Nunnally and S. W. Peterson, Enzyme Microb. Technol., 2013, 52, 203–210, DOI:10.1016/j.enzmictec.2012.12.002.
- T. Kiho, M. Sakushima, S. R. Wang, K. Nagai and S. Ukai, Chem. Pharm. Bull., 1991, 39, 798–800, DOI:10.1248/cpb.39.798.
- U. Rau, in Production of Schizophyllan, Carbohydrate biotechnology protocols, ed. C. Bucke, Totowa, Humana Press, 1999, DOI: DOI:10.1007/978-1-59259-261-6_4.
- M. O. Contour-Galcera, Y. Ding, C. Ortiz-Mellet and J. Defaye, Carbohydr. Res., 1996, 281, 119–128, DOI:10.1016/0008-6215(95)00334-7.
- M. Shoaib, S. M. R. Quadri, O. B. Wani, E. Bobicki, G. I. Garrido, A. Elkamel and A. Abdala, Carbohydr. Polym., 2020, 240, 116263, DOI:10.1016/j.carbpol.2020.116263.
- L. Kubala, J. Ruzickova, K. Nickova, J. Sandula, M. Ciz and A. Lojek, Carbohydr. Res., 2003, 338, 2835–2840, DOI:10.1016/j.carres.2003.09.007.
- S. Hamedi, S. A. Shojaosadati, V. Najafi and V. Alizadeh, Carbohydr. Polym., 2020, 229, 115383, DOI:10.1016/j.carbpol.2019.115383.
- Y. Ding, S. S. Alkan, G. Baschang and J. Defaye, Carbohydr. Res., 2000, 328, 71–76, DOI:10.1016/S0008-6215(00)00082-3.
- H. Kim, S. Lee and C. S. Ki, Carbohydr. Polym., 2021, 252, 117132, DOI:10.1016/j.carbpol.2020.117132.
- Z. Negahbana, S. A. Shojaosadatia and S. Hamedib, Colloids and Surfaces B: Biointerfaces, 2020. DOI: DOI:10.1016/j.colsurfb.2020.111524.
- D. Lee, Y. Kim, J. Kim, D. Kim, S. Kim, S. Y. Kim, K. Jang, J. Lee and C. Yang, Arch. Pharmacal Res., 2020, 43, 449–461, DOI:10.1007/s12272-020-01231-4.
- M. K. Singh, M. Kumar and I. S. Thakur, Bioresour. Technol., 2017, 236, 29–36, DOI:10.1016/j.biortech.2017.03.170.
- C. Shu and H. Hsu, J. Taiwan Inst. Chem. Eng., 2011, 42, 387–393, DOI:10.1016/j.jtice.2010.08.009.
- Y. Zhou, H. Zhang and C. Peng, Phytother. Res., 2014, 28, 961–975, DOI:10.1002/ptr.5083.
- Y. Liu, M. Yuan, C. Zhang, H. Liu, J. Liu, A. Wei, Q. Ye, B. Zeng, M. Li, Y. Guo and L. Guo, Biomed. Pharmacother., 2021, 134, 111121, DOI:10.1016/j.biopha.2020.111121.
- X. Yao, J. Yin, Y. Xia, Z. Wei, Y. Luo, M. Liu, C. Feleder and Y. Dai, J. Ethnopharmacol., 2012, 141, 322–330, DOI:10.1016/j.jep.2012.02.038.
- X. Zhang, Q. Liu, C. Zhang, J. Sheng, S. Li, W. Li, X. Yang, X. Wang, S. He, J. Bai and D. Zhu, J. Pharmacol. Sci., 2019, 141, 97–105, DOI:10.1016/j.jphs.2019.09.010.
- Y. Wenli, Z. Yaping and S. Bo, Food Chem., 2004, 86, 525–529, DOI:10.1016/j.foodchem.2003.09.005.
- Q. Huang, H. Zhang and D. Xue, Food Chem., 2017, 226, 89–94, DOI:10.1016/j.foodchem.2017.01.021..
- L. Zhang, A. K. Pan Siu, G. Lin and Z. Zuo, Chin. Med.-UK, 2011, 6, 41, DOI:10.1186/1749-8546-6-41.
- Q. Huang, H. Zhang and D. Xue, Food Sci. Biotechnol., 2019, 28, 1117–1124, DOI:10.1007/s10068-018-0540-9.
- J. Wu, X. Yang, J. Ge, Y. Zhang, L. Wu, J. Liu and X. Zhang, Bioresour. Technol., 2012, 111, 496–499, DOI:10.1016/j.biortech.2012.02.038.
- N. Wang, X. Zhang, S. Wang, Q. Guo, Z. Li, H. Liu and C. Wang, Carbohydr. Polym., 2020, 227, 115314, DOI:10.1016/j.carbpol.2019.115314.
- G. Sathiyanarayanan, D. Yi, S. K. Bhatia, J. Kim, H. M. Seo, Y. Kim, S. Park, D. Jeong, S. Jung, J. Jung, Y. K. Lee and Y. Yang, RSC Adv., 2015, 5, 84492–84502, 10.1039/c5ra14978a.
- S. Chen, H. Huang and G. Huang, Int. J. Biol. Macromol., 2019, 140, 1047–1053, DOI:10.1016/j.ijbiomac.2019.08.203.
- F. Huang, R. Hong, Y. Yi, Y. Bai, L. Dong, X. Jia, R. Zhang, G. Wang, M. Zhang and J. Wu, Int. J. Biol. Macromol., 2020, 147, 363–368, DOI:10.1016/j.ijbiomac.2020.01.059.
- H. Song, H. Chung and K. Nam, Environ. Pollut., 2021, 274, 116577, DOI:10.1016/j.envpol.2021.116577.
- C. Ma, J. Bai, C. Shao, J. Liu, Y. Zhang, X. Li, Y. Yang, Y. Xu and L. Wang, Food Res. Int., 2021, 143, 110281, DOI:10.1016/j.foodres.2021.110281.
- X. Lin, L. Zhou, T. Li, C. Brennan, X. Fu and R. H. Liu, RSC Adv., 2017, 7, 5751–5758, 10.1039/c6ra26596k.
- H. Wang, J. Wang, X. Guo, C. S. Brennan, T. Li, X. Fu, G. Chen and R. H. Liu, Food Chem., 2016, 205, 170–177, DOI:10.1016/j.foodchem.2016.03.001.
- X. Wang, C. Shao, L. Liu, X. Guo, Y. Xu and X. Lü, Int. J. Biol. Macromol., 2017, 103, 1173–1184, DOI:10.1016/j.ijbiomac.2017.05.118.
- G. Li, P. Chen, Y. Zhao, Q. Zeng, S. Ou, Y. Zhang, P. Wang, N. Chen and J. Ou, Carbohydr. Polym., 2021, 267, 118194, DOI:10.1016/j.carbpol.2021.118194.
- P. C. Wootton-Beard, A. Moran and L. Ryan, Food Res. Int., 2011, 44, 217–224, DOI:10.1016/j.foodres.2010.10.033.
- L. Zhou, X. Lin, A. M. Abbasi and B. Zheng, Biomed Res. Int., 2016, 2016, 1–9, DOI:10.1155/2016/8495630.
- M. Wang, P. Zhu, S. Zhao, C. Nie, N. Wang, X. Du and Y. Zhou, Int. J. Biol. Macromol., 2017, 95, 809–817, DOI:10.1016/j.ijbiomac.2016.12.010.
- A. Mohammadi, S. A. Shojaosadati, H. J. Tehrani, S. M. Mousavi, T. Saleh and A. C. Khorasani, Ann. Microbiol., 2018, 68, 47–62, DOI:10.1007/s13213-017-1316-9.
- J. Yan, L. Wu, Z. Qiao, W. Cai and H. Ma, Food Chem., 2019, 271, 588–596, DOI:10.1016/j.foodchem.2018.08.012.
- J. Yan, Z. Ding, X. Gao, Y. Wang, Y. Yang, D. Wu and H. Zhang, Carbohydr. Polym., 2018, 193, 373–382, DOI:10.1016/j.carbpol.2018.04.019.
- W. A. A. Q. Wan-Mohtar, R. Abd Malek, L. M. Harvey and B. McNeil, Biocatal. Agric. Biotechnol., 2016, 8, 24–31, DOI:10.1016/j.bcab.2016.08.002.
- M. Papagianni, Biotechnol. Adv., 2004, 22, 189–259, DOI:10.1016/j.biotechadv.2003.09.005.
- S. Jiang, N. Deng, B. Zheng, T. Li and R. H. Liu, Food Funct., 2021, 12, 4471–4483, 10.1039/d0fo02974b.
- M. G. Shehata, T. S. Awad, D. Asker, S. A. El Sohaimy, N. M. Abd El- Aziz and M. M. Youssef, Curr. Res. Food Sci., 2021, 4, 326–335, DOI:10.1016/j.crfs.2021.05.001.
- B. Song, H. Wang, W. Xia, B. Zheng, T. Li and R. H. Liu, Food Funct., 2020, 11, 617–6185, 10.1039/d0fo00718h.
- R. Jiang, K. Lau, H. Lam, W. Yam, L. Leung, K. Choi, M. M. Y. Waye, T. C. W. Mak, K. Woo and K. Fung, J. Ethnopharmacol., 2005, 96, 133–138, DOI:10.1016/j.jep.2004.08.029.
- H. Wang, X. Guo, X. Hu, T. Li, X. Fu and R. H. Liu, Food Chem., 2017, 217, 773–781, DOI:10.1016/j.foodchem.2016.09.002.
- E. Metreveli, E. Kachlishvili, S. W. Singer and V. Elisashvili, Bioresour. Technol., 2017, 241, 652–660, DOI:10.1016/j.biortech.2017.05.148.
- H. T. Simonsen, M. S. Nielsen, N. J. Christensen, U. Christensen, T. V. La Cour, M. S. Motawia, B. P. M. Jespersen, S. B. Engelsen and B. L. Møller, J. Agric. Food Chem., 2009, 57, 2056–2064, DOI:10.1021/jf802057v.
- Z. Lu, B. Cheng, Y. Hu, Y. Zhang and G. Zou, Food Chem., 2009, 113, 17–20, DOI:10.1016/j.foodchem.2008.04.042.
- J. Wang, Y. Cao, B. Sun and C. Wang, Food Chem., 2011, 124, 1069–1075, DOI:10.1016/j.foodchem.2010.07.080.
- C. Jullian, L. Moyano, C. Yañez and C. Olea-Azar, Spectrochim. Acta, Part A, 2007, 67, 230–234, DOI:10.1016/j.saa.2006.07.006.
- L. Wu, J. Zhao, X. Zhang, S. Liu and C. Zhao, Int. J. Biol. Macromol., 2021, 179, 116–124, DOI:10.1016/j.ijbiomac.2021.02.207.
- X. Meng, H. Liang and L. Luo, Carbohydr. Res., 2016, 424, 30–41, DOI:10.1016/j.carres.2016.02.008.
- S. J. Hur, S. Y. Lee, Y. Kim, I. Choi and G. Kim, Food Chem., 2014, 160, 346–356, DOI:10.1016/j.foodchem.2014.03.112.
- I. Lee, Y. Hung and C. Chou, Int. J. Food Microbiol., 2008, 121, 150–156, DOI:10.1016/j.ijfoodmicro.2007.09.008.
- M. I. Torino, R. I. Limón, C. Martínez-Villaluenga, S. Mäkinen, A. Pihlanto, C. Vidal-Valverde and J. Frias, Food Chem., 2013, 136, 1030–1037, DOI:10.1016/j.foodchem.2012.09.015.
- S. Dudonné, X. Vitrac, P. Coutière, M. Woillez and J. Mérillon, J. Agric. Food Chem., 2009, 57, 1768–1774, DOI:10.1021/jf803011r.
- H. J. Forman, K. J. A. Davies and F. Ursini, Free Radicals Biol. Med., 2014, 66, 24–35, DOI:10.1016/j.freeradbiomed.2013.05.045.
- J. A. Rufián-Henares and F. J. Morales, J. Agric. Food Chem., 2007, 55, 10016–10021, DOI:10.1021/jf0718291.
- B. Du and B. Xu, Bioact. Carbohydr. Diet. Fibre, 2014, 3, 11–16, DOI:10.1016/j.bcdf.2013.12.001.
- E. Niki, Free Radicals Biol. Med., 2010, 49, 503–515, DOI:10.1016/j.freeradbiomed.2010.04.016.
- M. Wang, M. Lei, N. Samina, L. Chen, C. Liu, T. Yin, X. Yan, C. Wu, H. He and C. Yi, Food Chem., 2020, 330, 127156, DOI:10.1016/j.foodchem.2020.127156.
Footnote |
† Electronic supplementary information (ESI) available. See DOI: 10.1039/d1ra06314f |
|
This journal is © The Royal Society of Chemistry 2021 |