DOI:
10.1039/D1RA05914A
(Paper)
RSC Adv., 2021,
11, 27782-27786
Visible-light synthesis of 4-substituted-chroman-2-ones and 2-substituted-chroman-4-ones via doubly decarboxylative Giese reaction†
Received
4th August 2021
, Accepted 6th August 2021
First published on 16th August 2021
Abstract
Doubly decarboxylative, photoredox synthesis of 4-substituted-chroman-2-ones and 2-substituted-chroman-4-ones is described. The reaction involves two independent decarboxylation processes: the first one initiating the cycle and the second completing the process. Visible light, photoredox catalyst, base, anhydrous solvent and inert atmosphere constitute the key parameters for the success of the developed transformation. The protocol proved applicable for coumarin-3-carboxylic acids and chromone-3-carboxylic acids as well as N-(acyloxy)phthalimide which served as precursors of the corresponding alkyl radicals.
Chroman-2-ones and their derivatives constitute privileged structural motifs present in various natural products (Scheme 1).1 Similarly, the chroman-4-one ring system can be found in various bioactive molecules relevant for the life-science industry.2 Representative examples of both groups of compounds are shown in Scheme 1. Soulamarin, isolated from stem bark has found application in folk medicine to treat rheumatism, varicose veins, haemorrhoids and ulcers.3 Recedensolide shows activity against human cervical epitheloid carcinoma.4 Flavanoids, such as eriodictyol and pinocembrin, are associated with reducing risk of certain chronic diseases.5 Natural flavanones isolated from flowers of Chromolaena odorata such as 4′-hydroxy-5,6,7-trimethoxyflavanone are reported to have antimycobacterial activity.6
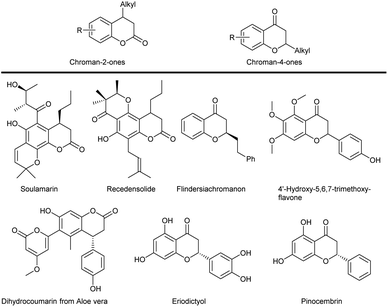 |
| Scheme 1 The importance of chroman-2-ones and chroman-4-ones. | |
The addition of free radicals to electron-deficient olefins is known in the literature as Giese reaction (Scheme 2).7 Recently, owing to the development of photocatalysis, the synthetic potential of this and related reactions has been vastly expanded.8 Advancements in this field arises from the development of photo-initiated methods allowing for free radical formation under mild and non-toxic conditions. An interesting way to generate free radicals involves the usage of N-(acyloxy)phthalimides.9 The formation of free radical is initiated by one-electron reduction with subsequent decarboxylation. Recently, the potential of this method has been confirmed in the Giese reaction with various electron-poor olefins.10
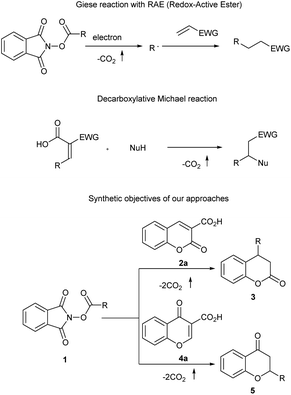 |
| Scheme 2 The importance of decarboxylative approaches in organic synthesis and the synthetic objectives of our study. | |
Decarboxylative Michael reaction that involves the addition to carboxylic-acid-activated olefins followed by decarboxylation reaction constitutes a powerful synthetic tool.11 Coumarin-3-carboxylic acids 2 and chromone-3-carboxylic acids 4 are useful reactants participating in this reaction opening access to biologically relevant chroman-2-ones 3 and chroman-4-ones 5.12,13 Recently, doubly decarboxylative reactions involving these reagents have also been developed.14 Surprisingly, decarboxylative Giese reaction with carboxylic-acid-activated olefins has not been a subject of studies so far. Herein, we report the first photocatalytic, doubly decarboxylative Giese reaction which is applicable to electron poor carboxylic acids. The developed strategy benefits from mild reaction conditions.
Optimization studies were performed using coumarin-3-carboxylic acid 2a and 1,3-dioxoisoindolin-2-yl cyclohexane carboxylate 1a (NHPI esters) as model substrates (Table 1). Initial experiments were performed in CH2Cl2 in the presence of DIPEA as a donor of electron and base the corresponding photoredox catalyst under LED irradiation (with the light source of suitable wavelength) under inert atmosphere. In the first part of the optimization studies, the catalytic activity of eight different photoredox catalysts was tested (Table 1, entries 1–8). When 9-mesityl-10-methylacridinium tetrafluoroborate 6a, chloranil 6b or 6h were used, the formation of target product 3aa was not observed (Table 1, entries 1 and 2). The best yield was obtained in the presence of Ru(bpy3)2(PF6)2 6f (Table 1, entry 6). In the course of further studies, the effect of the solvent on the reaction outcome was evaluated (Table 1, entries 9–13). The use of CHCl3 did not ensure the product formation (Table 1, entry 9). Similar effect was observed in THF and toluene (Table 1, entries 10 and 11). The desired reaction took place also in acetonitrile and DMF, however, lower yield than in the case of CH2Cl2 was obtained (Table 1, entries 12 and 13). Subsequently, the effect of base on the reaction outcome was evaluated (Table 1, entries 14 and 15). When triethylamine was used, the yield of the reaction decreased (Table 1, entry 14) and the application of quinine did not result in the product 3aa formation (Table 1, entry 15). In the course of further studies control experiments were performed (Table 1, entries 16–20). The reaction did not proceed in the absence of neither photoredox catalysts (Table 1, entry 16, presumably electron donor–acceptor complex of DIPEA with NHPI esters was not efficiently formed in this case), nor a base (Table 1, entry 17).9a Similar effect was observed when the transformation was attempted in the dark (Table 1, entry 18), thus confirming the crucial effect of photocatalyst and the source of light on the reaction outcome. The amount of the photocatalyst 6f was possible to be lowered to 5 mol% resulting in decrease of yield to 53% after 24 hours (Table 1, entry 19). On the other hand, it was possible to perform the reaction with DIPEA (1.2 equiv.) but the yield of the reaction decreased (Table 1, entry 20). Noteworthily, the reactivity was quenched in the presence of TEMPO confirming the radical mechanism of the process (Table 1, entry 21).
Table 1 Doubly decarboxylative Giese reaction – optimization studies involving coumarin-3-carboxylic acid 2aaa
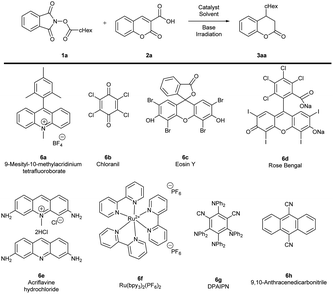
|
Entry |
Cat |
Solvent |
Base |
Cat. mol% |
Yield [%] |
All reactions were performed in a 0.20 mmol scale using 1a (1.2 equiv.) and 2a (1.0 equiv.) in the presence of the corresponding photoredox catalyst 6 (10 mol%) and the corresponding base (2 equiv.) in the solvent (3 mL). Reaction performed under irradiation with the blue light. Reaction performed under irradiation with the violet light. Reaction performed under irradiation with the green light. Reaction performed without catalyst. Reaction performed in the dark. The reaction performed in the presence of DIPEA (1.2 equiv.). Reaction performed in the presence of TEMPO (1 equiv.). |
1b |
6a |
CH2Cl2 |
DIPEA |
10 |
— |
2c |
6b |
CH2Cl2 |
DIPEA |
10 |
— |
3d |
6c |
CH2Cl2 |
DIPEA |
10 |
56 |
4d |
6d |
CH2Cl2 |
DIPEA |
10 |
63 |
5b |
6e |
CH2Cl2 |
DIPEA |
10 |
26 |
6b |
6f |
CH2Cl2 |
DIPEA |
10 |
82 |
7b |
6g |
CH2Cl2 |
DIPEA |
10 |
42 |
8b |
6h |
CH2Cl2 |
DIPEA |
10 |
— |
9b |
6f |
CHCl3 |
DIPEA |
10 |
— |
10b |
6f |
THF |
DIPEA |
10 |
— |
11b |
6f |
PhMe |
DIPEA |
10 |
— |
12b |
6f |
DMF |
DIPEA |
10 |
61 |
13b |
6f |
MeCN |
DIPEA |
10 |
58 |
14b |
6f |
CH2Cl2 |
TEA |
10 |
47 |
15b |
6f |
CH2Cl2 |
Quinine |
10 |
— |
16e |
— |
CH2Cl2 |
DIPEA |
— |
— |
17b |
6f |
CH2Cl2 |
— |
10 |
— |
18f |
6f |
CH2Cl2 |
DIPEA |
10 |
— |
19b |
6f |
CH2Cl2 |
DIPEA |
5 |
53 |
20g |
6f |
CH2Cl2 |
DIPEA |
10 |
35 |
21h |
6f |
CH2Cl2 |
DIPEA |
10 |
— |
With the optimized reaction conditions in hand (Table 1, entry 6), the applicability of the developed methodology was studied (Schemes 3 and 4). Initially, various coumarin-3-carboxylic acids 2a–i were tested (Scheme 3). Acids 2b–d bearing electron-donating groups on the aromatic ring provided products 3ab–ad with higher yields. Similarly, carboxylic acids 2e–i with electron-withdrawing groups also provided products with good yields. The lowest yield was obtained for coumarin-3-carboxylic acid 2h with chlorine substituent in the 8-position. In the second stage of the scope studies various N-(acyloxy)phthalimides 1a–e were investigated (Scheme 3). N-(Acyloxy)phthalimides 1a–e that served as precursors of secondary and tertiary alkyl radicals turned out to be suitable components in the developed reaction. Target products 3ba–ea were efficiently formed. However, the use of primary radicals turned out to be problematic. In the case of benzylic radical precursor, a dimerization of the corresponding radical was faster than the addition to the electrophile 2a.
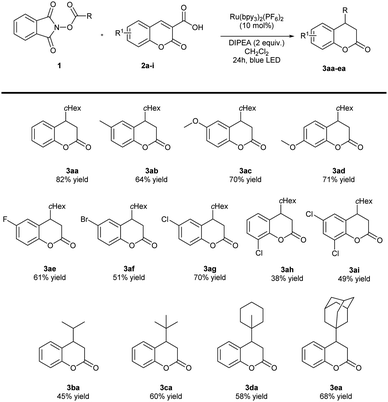 |
| Scheme 3 Doubly decarboxylative Giese reaction – reaction involving coumarin-3-carboxylic acids 2. | |
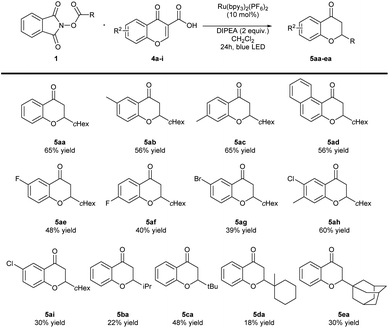 |
| Scheme 4 Doubly decarboxylative Giese reaction – reaction involving chromone-3-carboxylic acids 4. | |
In the second part of our studies, chromone-3-carboxylic acids 4 were tested as acceptors in the doubly decarboxylative Giese reaction employing the same conditions that were used in the case of coumarine-3-carboxylic acids 2 (Scheme 4). The use of 4b–d bearing electron-donating groups provided 5ab, 5ac, 5ad with good results. On the other hand, the reaction of chromone-3-carboxylic acids 4e–i with electron-withdrawing groups provided products 5ae–5ai with slightly lower efficiency. Chromone-3-carboxylic acid 4h bearing two substituents with opposite electronic effects was also well-tolerated. Other alkyl radical precursors 1b–e were also utilized in the reaction with chromone-3-carboxylic acid 4a. The tert-butyl substituted product 5ca was obtained with the highest yield in this part of the study. Unfortunately, the rest of examples showed lower responsiveness to participate in the reaction.
The postulated mechanism of both developed reactions is similar and begins with photocatalyst excitation (Scheme 5). Then the electron transfer from the amine to the photocatalyst takes place. Fluorescence quenching and cyclic voltammetry experiments confirmed the lack of quenching in the case of acids 2a or 4a as well as N-(acyloxy)phthalimide 1a (for details see ESI†). Subsequently, Ru(I) species acts as reductant of the N-(acyloxy)phthalimide 6. In turn, 7 undergoes decarboxylative degradation with the formation of an alkyl radical 9. The newly formed radical 9 undergoes Giese reaction with the acceptor 10 or 11. Transfer of the hydrogen atom from the radical cation originating from amine and subsequent decarboxylative protonation terminates the reaction affording 3 or 5 as target products.10a
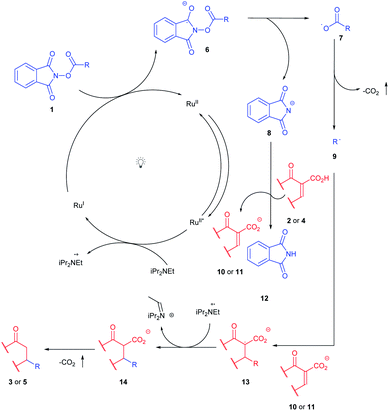 |
| Scheme 5 Doubly decarboxylative Giese reaction – mechanism. | |
Conclusions
In conclusion, we have developed a doubly decarboxylative photocatalytic Giese reaction. It exemplifies the unique application of a free carboxylic-acid-activated olefins in a radical transformation. The reaction was applicable to a carboxylic acids as various coumarin-3-carboxylic acids 2a–i and chromone-3-carboxylic acids 4a–i served as effective Giese acceptors. The reaction can be described as doubly decarboxylation process with the first decarboxylation initiating the cycle and the second completing the process. Target, biologically relevant 4-substituted-chroman-2-ones 3aa–ea and 2-substituted-chroman-4-ones 5aa–ea were obtained in good to high yields under mild reaction conditions.
Conflicts of interest
There are no conflicts to declare.
Acknowledgements
This work was financially supported by Ministry of Science and Higher Education Poland within the Diamond grant programme realized in the period 2018–2022, project number: 0016/DIA/2018/47. Thanks are expressed to Angelika Artelska and Adam Sikora (Faculty of Chemistry, Lodz University of Technology) for performing fluorescence quenching. This contribution has been completed while the second author (EK) was the Doctoral Candidate in the Interdisciplinary Doctoral School of Lodz University of Technology, Poland.
References
-
(a) D. P. Kamat, S. G. Tilve, V. P. Kamat and J. K. Kirtany, Syntheses and Biological Activities of Chroman-2-ones, Org. Prep. Proc. Int., 2015, 47, 1 CrossRef CAS;
(b) T. Okamoto, T. Kobayashi and S. Yoshida, Chemical aspects of coumarin compounds for the prevention of hepatocellular carcinomas, Curr. Med. Chem., 2005, 5, 47 CAS;
(c) F. Asai, M. Iinuma, T. Tanaka and M. Mizuno, Complex flavonoids in farinose exudate from Pityrogramma calomelanos, Phytochemistry, 1991, 30, 3091 CrossRef CAS.
-
(a) Ø. M. Andersen and K. R. Markham, Flavonoids: Chemistry, Biochemistry and Applications. CRC, Taylor & Francis, Boca Raton, FL, 2006 Search PubMed;
(b) S. Emami and Z. Ghanbarimasir, Recent advances of chroman-4-one derivatives: synthetic approaches and bioactivities, Eur. J. Med. Chem., 2015, 93, 539 CrossRef CAS PubMed;
(c) K.-S. Masters and S. Bräse, Xanthones from fungi, lichens, and bacteria: the natural products and their synthesis, Chem. Rev., 2012, 112, 3717 CrossRef CAS PubMed;
(d) A. D. Patil, A. J. Freyer, D. S. Eggleston, R. C. Haltiwanger, M. F. Bean, P. B. Taylor, M. J. Caranfa, A. L. Breen, H. R. Bartus and R. K. Johnson, The inophyllums, novel inhibitors of HIV-1 reverse transcriptase isolated from the Malaysian tree, Calophyllum inophyllum Linn, J. Med. Chem., 1993, 36, 4131 CrossRef CAS PubMed;
(e) D. L. Galinis, R. W. Fuller, T. C. McKee, J. H. II Cardellina, R. J. Gulakowski, J. B. McMahon and M. R. Boyd, Structure-Activity Modifications of the HIV-1 Inhibitors (+)-Calanolide A and (−)-Calanolide B1, J. Med. Chem., 1996, 39, 4507 CrossRef CAS PubMed.
- G. C. L. S. Ee, H. Mah, S. S. Teh, M. Rahmani, R. Go and Y. H. Taufiq-Yap, Soulamarin, a new coumarin from Stem Bark of Calophyllum soulattri, Molecules, 2011, 16, 9721 CrossRef CAS PubMed.
- Y. Shen, L. Wang, A. Khalil and Y. Kuo, Chromanones and dihydrocoumarins from Calophyllum blancoi, Chem. Pharm. Bull., 2004, 52, 402 CrossRef CAS PubMed.
- D. Genovese, C. Conti, P. Tomae, N. Desideri, M. Setin, S. Catone and L. Flore, Effect of chloro-, cyano-, and amidino-substituted flavanoids on enterovirus infection in vitro, Antiviral Res., 1995, 27, 123 CrossRef CAS PubMed.
- A. Suksamrarn, A. Chotipong, T. Suavansri, S. Boongird, P. Tirnsuksai, S. Vimuttipong and A. Chuaynugu, Antimycobacterial Activity and Cytotoxicity of Flavonoids from the Flowers of Chromolaena odorata., Arch. Pharmacal Res., 2004, 27, 507 CrossRef CAS PubMed.
-
(a) B. Giese, J. A. Gonzalez-Gomez and T. Witzel, The Scope of Radical CC-Coupling by the “Tin Method”, Angew. Chem., Int. Ed., 1984, 23, 69 CrossRef;
(b) L. Chu, C. Ohta, Z. Zuo and D. W. C. MacMillan, Carboxylic Acids as A Traceless Activation Group for Conjugate Additions: A Three-Step Synthesis of (±)-Pregabalin, J. Am. Chem. Soc., 2014, 136, 10886 CrossRef CAS PubMed;
(c) J. Schwarz and B. König, Metal-Free, Visible-Light-Mediated, Decarboxylative Alkylation of Biomass-Derived Compounds, Green Chem., 2016, 18, 4743 RSC;
(d) N. P. Ramirez and J. C. Gonzalez-Gomez, Decarboxylative Giese-Type Reaction of Carboxylic Acids Promoted by Visible Light: A Sustainable and Photoredox-Neutral Protocol, Eur. J. Org. Chem., 2017, 2154 CrossRef CAS;
(e) J. Rostoll-Berenguer, G. Blay, J. R. Pedro and C. Vila, Photocatalytic Giese Addition of 1,4-Dihydroquinoxalin-2-ones to Electron-Poor Alkenes Using Visible Light, Org. Lett., 2020, 22, 8012 CrossRef CAS PubMed;
(f) F. El-Hage, C. Schöll and J. Pospech, Photo-Mediated Decarboxylative Giese-Type Reaction Using Organic Pyrimidopteridine Photoredox Catalysts, J. Org. Chem., 2020, 85, 13853 CrossRef CAS PubMed.
-
(a) R. Brimioulle, D. Lenhart, M. M. Maturi and T. Bach, Enantioselective Catalysis of Photochemical Reactions, Angew. Chem., Int. Ed., 2015, 54, 3872 CrossRef CAS PubMed;
(b) L. Buzzetti, G. E. M. Crisenza and P. Melchiorre, Mechanistic Studies in Photocatalysis, Angew. Chem., Int. Ed., 2019, 58, 3730 CrossRef CAS PubMed;
(c) A. Vega-Peñaloza, J. Mateos, X. Companyó, M. Escudero-Caso and L. Dell'Amico, A Rational Aproach to Organo-Photocatalysis Novel Designs and Structure-Property Relationships, Angew. Chem., Int. Ed., 2021, 60, 1082 CrossRef PubMed;
(d) N. A. Romero and D. A. Nicewicz, Organic Photoredox Catalysis, Chem. Rev., 2016, 17, 10075 CrossRef PubMed;
(e) M.-C. Fu, R. Shang, B. Zhao, B. Wang and Y. Fu, Photocatalytic decarboxylative alkylations mediated by triphenylphosphine and sodium iodide, Science, 2019, 363, 1429 CrossRef CAS PubMed.
-
(a) S. K. Parida, T. Mandal, S. Das, S. K. Hota, S. De Sarkar and S. Murarka, Single Electron Transfer-Induced Redox Processes Involving N-(Acyloxy)phthalimides, ACS Catal., 2021, 11, 1640 CrossRef CAS;
(b) S. Shibutani, K. Nagao and H. Ohmiya, Organophotoredox-Catalyzed Three-Component Coupling of Heteroatom Nucleophiles, Alkenes, and Aliphatic Redox Active Esters, Org. Lett., 2021, 23, 1798 CrossRef CAS;
(c) Y. Dong, P. Ji, Y. Zhang, Ch. Wang, X. Meng and W. Wang, Organophotoredox-Catalyzed Formation of Alkyl-Aryl And -Alkyl C-S/Se Bonds from Coupling of Redox-Active Esters with Thio/Selenosulfonates, Org. Lett., 2020, 22, 9562 CrossRef CAS PubMed;
(d) T. Saget and B. König, Photocatalytic Synthesis of Polycyclic Indolones, Chem. Eur. J., 2020, 26, 7004 CrossRef CAS PubMed;
(e) C. Zheng, G.-Z. Wang and R. Shang, Catalyst-free Decarboxylation and Decarboxylative Giese Additions of Alkyl Carboxylates through Photoactivation of Electron Donor-Acceptor Complex, Adv. Synth. Catal., 2019, 361, 4500 CrossRef CAS;
(f) K. Okada, K. Okamoto, N. Morita, K. Okubo and M. Oda, Photosensitized decarboxylative Michael addition through N-(acyloxy)phthalimides via an electron-transfer mechanism, J. Am. Chem. Soc., 1991, 113, 9401 CrossRef CAS;
(g) G.-Z. Wang, M.-C. Fu, B. Zhao and R. Shang, Photocatalytic decarboxylative alkylations of C(sp3)–H and C(sp2)–H bonds enabled by ammonium iodide in amide solvent, Sci. China Chem., 2021, 64, 439 CrossRef CAS;
(h) M. Tian, Y. Wang, X. Bu, Y. Wang and X. Yang, An ultrastable olefin-linked covalent organic framework for photocatalytic decarboxylative alkylations under highly acidic conditions, Catal. Sci. Technol., 2021, 11, 4272 RSC.
-
(a) K. N. Tripathi, Md. Belal and R. P. Singh, Organo Photoinduced Decarboxylative Alkylation of Coumarins with N-(Acyloxy)phthalimide, J. Org. Chem., 2020, 85, 1193 CrossRef CAS PubMed;
(b) S. Das, S. Kumar Parida, T. Mandal, S. K. Hota, L. Roy, S. D. Sarkar and S. Murarka, An organophotoredox-catalyzed redox-neutral cascade involving N-(acyloxy)phthalimides and maleimides, Org. Chem. Front., 2021, 8, 2256 RSC.
-
(a) J.-J. Zhang, J.-C. Yang, L.-N. Guo and X.-H. Duan, Visible-Light-Mediated Dual Decarboxylative Coupling of Redox-Active Esters with α,β-Unsaturated Carboxylic Acids, Chem.–Eur. J., 2017, 23, 10259 CrossRef CAS PubMed;
(b) K. Xu, Z. Tan, H. Zhang, J. Liu, S. Zhang and Z. Wang, Photoredox catalysis enabled alkylation of alkenyl carboxylic acids with N-(acyloxy)phthalimide via dual decarboxylation, Chem. Commun., 2017, 53, 10719 RSC ; for reviews on decarboxylative strategies, see: ;
(c) Y. Pan and C.-H. Tan, Catalytic Decarboxylative Reactions: Biomimetic Approaches Inspired by Polyketide Biosynthesis, Synthesis, 2011, 2044 CAS;
(d) Z.-L. Wang, Recent Advances in Catalytic Asymmetric Decarboxylative Addition Reactions, Adv. Synth. Catal., 2013, 355, 2745 CrossRef CAS;
(e) S. Nakamura, Catalytic enantioselective decarboxylative reactions using organocatalysts, Org. Biomol. Chem., 2014, 12, 394 RSC;
(f) J. Bojanowski and A. Albrecht, Carboxylic-Acid-Activated Olefins in Decarboxylative Reactions, Asian J. Org. Chem., 2019, 8, 746 CrossRef CAS.
-
(a) L. Xu, Z. Shao, L. Wang and J. Xiao, Tandem sp3 C-H Functionalization/Decarboxylation of 2-Alkylazaarenes with Coumarin-3-carboxylic Acids, Org. Lett., 2014, 16, 796 CrossRef CAS PubMed;
(b) F. Han, S. Xun, L. Jia, Y. Zhang, L. Zou and L. Hu, Traceless-Activation Strategy for Rh-Catalyzed Csp2 −H Arylation of Coumarins, Org. Lett., 2019, 21, 5907 CrossRef CAS PubMed.
-
(a) Z. Shao, L. Wang, L. Xu, H. Zhao and J. Xiao, Facile synthesis of azaarenes-2-substituted chromanone derivatives via tandem sp3 C–H functionalization/decarboxylation of azaarenes with 4-oxo-4H- chromene-3-carboxylic acid, RSC Adv., 2014, 4, 53188 RSC;
(b) A. G. Neo, J. Diaz, S. Marcaccinib and C. F. Marcos, Conjugate addition of isocyanides to chromone 3-carboxylic acid: an efficient one-pot synthesis of chroman-4-one 2-carboxamides, Org. Biomol. Chem., 2012, 10, 3406 RSC.
-
(a) S. Nakamura, A. Toda, M. Sano, T. Hatanaka and Y. Funahashi, Organocatalytic Enantioselective Conjugate Addition of Malonic Acid Half Thioesters to Coumarin-3-carboxylic Acids Using N-Heteroarenesulfonyl Cinchona Alkaloid Amide, Adv. Synth. Catal., 2016, 358, 1029 CrossRef CAS;
(b) S. Peng, L. Wang, H. Guo, S. Sunb and J. Wang, Facile synthesis of 4-substituted 3,4-dihydrocoumarins via an organocatalytic double decarboxylation process, Org. Biomol. Chem., 2012, 10, 2537 RSC;
(c) A. Albrecht, Utilization of Chromone-3-Carboxylic Acids as Acceptors in the Michael-Type Decarboxylative Addition, Eur. J. Org. Chem., 2018, 648 Search PubMed.
Footnote |
† Electronic supplementary information (ESI) available: See DOI: 10.1039/d1ra05914a |
|
This journal is © The Royal Society of Chemistry 2021 |