DOI:
10.1039/D1RA05888F
(Paper)
RSC Adv., 2021,
11, 34402-34409
Reversible CO2 storage and efficient separation using Ca decorated porphyrin-like porous C24N24 fullerene: a DFT study†
Received
3rd August 2021
, Accepted 13th October 2021
First published on 25th October 2021
Abstract
The search for novel materials for effective storage and separation of CO2 molecules is a critical issue for eliminating or lowering this harmful greenhouse gas. In this paper, we investigate the potential application of a porphyrin-like porous fullerene (C24N24) as a promising material for CO2 storage and separation using thorough density functional theory calculations. The results show that CO2 is physisorbed on bare C24N24, implying that this material cannot be used for efficient CO2 storage. Coating C24N24 with Ca atoms, on the other hand, can greatly improve the adsorption strength of CO2 molecules due to polarization and charge-transfer effects. Furthermore, the average adsorption energy for each of the maximum 24 absorbed CO2 molecules on the fully decorated Ca6C24N24 fullerene is −0.40 eV, which fulfills the requirement needed for efficient CO2 storage (−0.40 to −0.80 eV). The Ca coated C24N24 fullerene also have a strong potential for CO2 separation from CO2/H2, CO2/CH4, and CO2/N2 mixtures.
1. Introduction
The discovery of fullerene C60 in 1985 (ref. 1) and subsequently of related materials like carbon nanotubes (CNTs)2,3 and graphene4,5 marked the beginning of a new era in carbon chemistry. Numerous potential applications of these nanosized carbon structures have been proposed, including hydrogen storage,6–8 microelectronics,9,10 and smart and novel composites.11,12 These structures are also well suited for use as a support in heterogeneous catalysis due to their huge specific area.13–15 The ability to tune the electronic and surface properties of carbon-based nanomaterials by introducing defects or heteroatoms has shed light on their potential use in sensors and energy technologies.16–19 Theoretical calculations20–22 and experimental investigations,23,24 for example, have demonstrated that N atoms may be effectively incorporated in different carbon materials, tailoring their chemical and electronic characteristics. Although the loading of metal atoms on pure carbon nanomaterials usually results in aggregation due to weak metal–surface interactions, previous studies have demonstrated that the doping of N atoms can substantially enhance metal atom dispersion on these systems due to polarized C–N bonds.22,25,26 Furthermore, a variety of porous N-rich carbon nanomaterials including graphitic carbon nitride (g-C3N4)27,28 and porphyrin like graphene29–31 or CNTs32,33 have been prepared using different experimental methods. There is also evidence that N-rich porous carbon fullerenes can provide as active sites for metal atoms by forming strong metal–N covalent bonds.34,35 C24N24, a truncated N-doped C60 fullerene, is the best example of such systems. This fullerene comprises six porphyrin-like N4 cavities, each of which is made up of eight s-triazine rings linked together by C–C bonds.36 Metal atoms may be efficiently trapped by these N4 cavities, and the clustering problem of metal atoms is therefore avoided due to the strong metal–N interactions. In fact, the potential of such single atom catalysts (SACs) based on porous C24N24 has been found in a variety of chemical processes, including N2O37 or O2 reduction,38 and CO oxidation.39
Growing energy demands, along with the ongoing use of fossil fuels in vehicles and power plants, has resulted in serious environmental issues such as emission of greenhouse gases and air pollution. CO2 is the most dangerous and prevalent greenhouse gas that has the potential to contribute to global warming and climate change.40 Given these facts, several strategies have been explored in order to lower the level of CO2 in the atmosphere. The most essential and straightforward method is to switch from fossil fuels to cleaner ones, such as natural gas.41 Natural gas emits less CO2 than other fossil fuels and is inexpensive and widely available. Landfills are a source of natural gas, but they can also contain significant amounts of CO2. As a result, separating CO2 from methane is a critical issue.42 Hydrogen is a clean fuel that emits no CO2 when burned, therefore the separation of CO2 from H2 is also important for practical purposes.43 Another alternative is to look into potential CO2 storage, capture, or transformation technologies.44–47 Recently, there has been a lot of interest in the capture and storage of CO2 on porous materials with high selectivity and capacity, such as zeolites48,49 and covalent organic frameworks.50,51 However, for efficient CO2 storage, these materials generally require either low temperature or high pressure. As a result, it is critical to investigate novel materials capable of capturing CO2 under normal conditions.
CO2 mostly acts as a Lewis acid in supramolecular chemistry by accepting electrons from the host material, but there is evidence that it may also serve as a Lewis base due to the existence of O lone-pair electrons.52 Because N-doped carbon nanostructures such as graphene, fullerenes, and CNTs are electron-rich materials, they may initially be assumed to be effective CO2 adsorbents. However, previous DFT calculations53,54 have shown that CO2 is generally weakly physisorbed on these surfaces due to lack of a permanent dipole moment. Some effective ways for solving the problem have been proposed, such as coating these substrates with atoms such as Ca,55,56 using an external electric field57,58 and charge regulation of the substrate.59 For instance, prior studies have shown that coating carbon fullerenes or their analogues60,61 with Ca atoms can significantly increase the CO2 storage capacity of these systems. The current work aims to investigate CO2 adsorption and storage on bare and Ca coated C24N24 fullerenes using density functional theory (DFT) calculations. It appears that the existence of N4 cavities in C24N24 not only provides active sites for Ca atoms to be located, but also induces a significant positive charge on the Ca, which improves adsorption abilities towards CO2 molecules. Surprisingly, the addition of Ca atoms causes C24N24 to behave as a Lewis acid when it interacts with CO2. Furthermore, the capability of Ca coated C24N24 fullerenes to separate CO2 from various gas mixtures (CO2/N2, CO2/CH4, CO2/H2) is investigated.
2. Computational details
The Perdew–Burke–Ernzerhof (PBE)62 formalism within the DMol3 (ref. 63 and 64) was used to execute spin polarized DFT computations. To get reliable results, an all-electron double-numeric atomic orbital basis set (DNP) was used, which was augmented by d-polarization functions. Fully optimized structures were obtained without the application of symmetry constraints. In this study, the Hirshfeld analysis was used to provide atomic charges and charge-transfer values. The Grimme's PBE + D2 (ref. 65) method was used to account for weak van der Waals interactions. A convergence tolerance of 1 × 10−5 Ha, 0.001 Ha Å−1, and 0.005 Å was used for energy change, maximum force, and maximum displacement, respectively. The porous C24N24 was produced as follows. First, two carbon atoms of C60 fullerene linking the two five-membered rings were deleted, and then four carbon atoms in the closest nearby places were replaced with N atoms. For the computations, a periodic cubic box with dimensions a = b = c = 30 Å was used, the Brillouin zone sampling by the Γ point.
The following equation was used to calculate the adsorption energy per adsorbed CO2 molecule Eads(CO2), on Ca coated C24N24 clusters:
|
Eads(CO2) = 1/n[Ecomplex − Ecluster − nECO2]
| (1) |
where
n is the number of adsorbed CO
2 molecules,
Ecomplex represents the energy of CO
2 adsorbed on the Ca coated C
24N
24 structure,
Ecluster represents the energy of Ca coated C
24N
24, and
ECO2 is the energy of a free CO
2 molecule.
The adsorption of CO2 is supposed to cause a mutual redistribution of electron density on C24N24 as well as on CO2. To demonstrate this, we used the following equation to obtain electron density difference (EDD) maps:
|
EDD = ρ(CO2@C24N24) − ρ(CO2) − ρ(C24N24)
| (2) |
in which
ρ(CO
2@C
24N
24),
ρ(CO
2) and
ρ(C
24N
24) are the electron densities of CO
2 adsorbed on C
24N
24, free CO
2 and C
24N
24, respectively.
The Bader's atoms in molecules (AIM)66 theory was utilized to investigate the nature of the interaction between CO2 molecules and Ca coated C24N24 fullerenes. In summary, the AIM theory is based on the topology of the electron density. In the AIM theory, the nature of the interaction between two interacting atoms is characterized by analyzing electron density (ρBCP) and its Laplacian (∇2ρBCP) at a specific point called the bond critical point (BCP), where the gradient of the electron density is zero.66 For a closed-shell or noncovalent interaction, such as most weak and moderate hydrogen bonds, the electron density at the BCPs is low and the Laplacian is positive. For a chemical bonding, on the other hand, the ρBCP is big and its ∇2ρBCP is negative at the corresponding BCP. In the AIM, another useful indicator for analyzing the nature of the interaction is the total electron energy density (HBCP), whose sign, together with the sign of ∇2ρBCP, may indicate whether the interaction is electrostatic or covalent. That is, ∇2ρBCP < 0 and HBCP < 0 for strong covalent bonds; ∇2ρBCP > 0 and HBCP > 0 for electrostatic interactions; and ∇2ρBCP > 0 and HBCP < 0 for partially covalent interactions.67 To perform the AIM analysis, wavefunctions were first generated at the PBEPBE/6-31G* level using the PBE/DNP optimized geometries by means of Gaussian 09 suite of program.68 The AIM2000 (ref. 69) was then used for topology analysis of the electron densities.
3. Results and discussion
3.1. Bare C24N24
Fig. 1a depicts the optimized structure of bare C24N24 cluster. It was prepared via truncated N-doping of C60 fullerene in accordance with ref. 37. As can be seen, C24N24 is made up of six porphyrine-like N4 cavities, each of which is composed of eight s-triazine rings connected by C–C bonds. The optimized C–N and C–C bond lengths in C24N24 are 1.34 and 1.55 Å, respectively, which are consistent with other DFT studies.36,38,70 The Hirshfeld charges on the N and C atoms are determined to be −0.12 and +0.12|e|, respectively, suggesting that C24N24 has a partially polarized C–N bonds. The formation energy (Eform) of C24N24 is determined to be about −6.0 eV, which is defined as Eform = 1/48[EC24N24 − 24EC − 24EN], where EC24N24 is the energy of C24N24 and EC and EN are the energies of a single C and N atom, respectively. This negative number, which agrees satisfactory with that reported by Srinivasu and Ghosh,36 suggests that the formation of C24N24 is a thermodynamically favorable process.
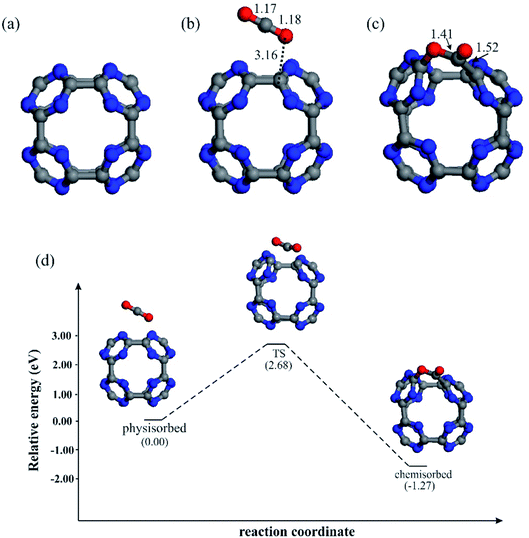 |
| Fig. 1 (a) The optimized structure of C24N24, (b) physisorbed structure of CO2 on C24N24, (c) chemisorbed structure of CO2 on C24N24 and (d) the energy map for the physisorbed-to-chemisorbed transformation of CO2 on of C24N24. The color code of the atoms: O (red); C (gray); N (blue). The values on the optimized structures are bond distances (in Å). | |
To assess the CO2 storage capability of bare C24N24, we first study the adsorption properties of a single CO2 molecule on this system. Fig. 1b and c depict the most stable geometries of a CO2 molecule adsorbed on C24N24. Consistent with other DFT studies,71,72 two stable configurations were located for CO2, namely the physisorbed and chemisorbed. In the physisorbed configuration, CO2 is nearly parallel to the C–C bond of fullerene, and the minimum binding distance between CO2 and C24N24 is 3.16 Å. The adsorption energy (Eads) is −0.14 eV, which is much smaller than the minimum target for an efficient storage of CO2 molecule (−0.40 eV).73 Meanwhile, this low adsorption energy and negligible charge transfer (0.02|e|) owing to CO2 adsorption suggest that bare C24N24 cannot efficiently adsorb CO2. Our DFT results, on the other hand, show that CO2 may be strongly chemisorbed on the C24N24, with an adsorption energy of −1.42 eV. This Eads value is much larger than that of on the B80 (ref. 71) and B40 (ref. 72) clusters, suggesting that CO2 is strongly bound to the C24N24. However, unlike the physisorbed structure, the formation of the chemisorbed structure on C24N24 requires the passing of an activation barrier. To investigate the mechanism of CO2 chemisorption, the potential energy profile for the physisorption-to-chemisorption conversion of CO2 molecule is investigated (Fig. 1d). Our results demonstrate that, while the conversion of physisorbed-to-chemisorbed configuration on C24N24 is exothermic, a significant activation barrier must be overcome for this process to occur. As a result, C24N24 cannot be used as an efficient CO2 storage material at room temperature.
3.2. Ca coated C24N24
The interaction of a Ca atom with C24N24 is then considered. The most stable atomic configuration for a Ca atom adsorbed on the C24N24 is shown in Fig. 2a. The Ca atom, like transition metals,38,74–76 prefers to reside in the N4 cavity, with an Eads value of −6.85 eV. The distance between the Ca atom and the adjacent N atoms is 2.33 Å. Furthermore, the presence of the Ca atom alters slightly the geometry of C24N24, as evidenced by the elongation of adjacent C–N bond lengths from 1.34 Å in bare C24N24 to 1.38 Å in CaC24N24. The decorated Ca atom is positively charged by 0.74|e|, whereas the nitrogen atoms around it are negatively charged by 0.17|e|, according to the Hirshfeld analysis. Due to Coulomb interactions, such a large charge transfer might be helpful to the stability of Ca atoms coated on C24N24. Furthermore, the significant localization of positive charge on the Ca atom may generate an electric field that polarizes the adsorbed CO2 molecules, fascinating their adsorption. Fig. 2a also shows the associated EDD map, which is used to evaluate the degree of electron density redistribution induced by Ca adsorption. A significant electron density loss region emerges on the Ca atom as a result of the formation of the CaC24N24 complex, demonstrating the ability of this site to interact with the electron donating species. Meanwhile, electron density gain regions exist between the Ca and N atoms, confirming the formation of Ca–N covalent bonds in CaC24N24. The PDOS analysis in Fig. 2a, on the other hand, clearly shows that the electronic states of the Ca and its surrounding N atoms are substantially mixed above and below the Fermi level (E = 0). As a consequence, it is reasonable to conclude that charge-transfer effects, in addition to electrostatic and polarization interactions between Ca and N atoms, are driving forces in the formation of Ca–N bonds.
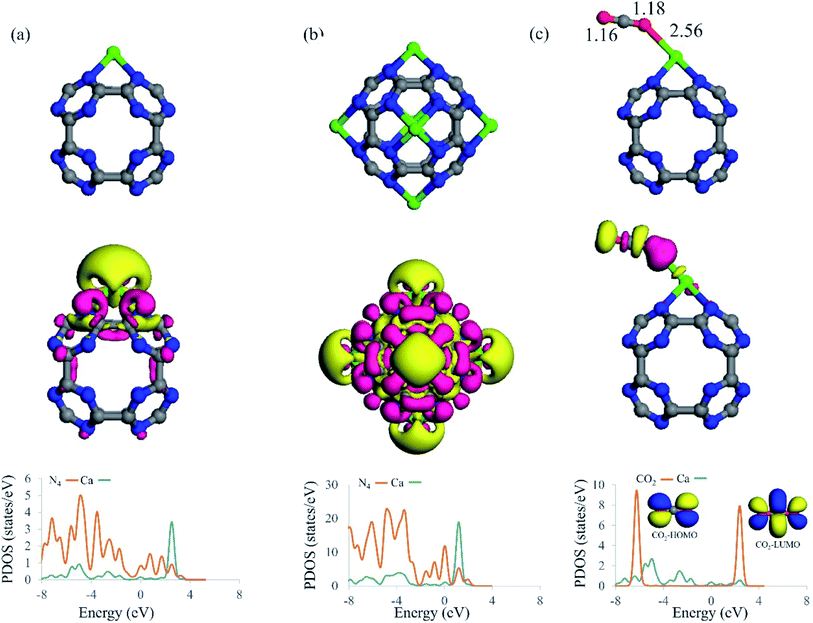 |
| Fig. 2 The optimized structure (above), corresponding EDD (middle, isovalue = 0.002 au) and the PDOS plots of (a) CaC24N24, (b) Ca6C24N24 and (c) CO2@CaC24N24. In the EDD plots, the electron gain and loss regions are denoted by pink and yellow colors, respectively. The Fermi level in the PDOS plots is set to zero. The color code of the atoms: Ca (green); O (red); C (gray); N (blue). | |
Fig. 1b shows the optimized structure of Ca6C24N24 cluster, in which one Ca atom is placed on each N4 cavity of C24N24. According to the calculations, the Eads value per Ca atom in this system is −6.31 eV, which is less negative than that of CaC24N24, partly due to repulsion between the decorated Ca atoms. To clarify this, we removed C24N24 from Ca6C24N24 and calculated the single-point energy of the remaining 6 Ca atoms. The average energy of the leftover 6 Ca atoms was determined to be 0.17 eV higher than the energy of a single Ca atom. On the other hand, as the number of the incorporated Ca atoms increases, it is natural to expect that the tendency of the cluster to accept electrons from the Ca atoms decreases. Hence, we believe that the lower average Eads value of Ca atom compared to the Eads value of CaC24N24 can be attributed to the latter two effects. This is supported by the Hirshfeld analysis, which shows that the average positive charge (0.64|e|) on the Ca atoms in Ca6C24N24 is likewise less than the positive charge on the Ca atom in CaC24N24 (0.74|e|). Furthermore, the significant electron density shifts caused by Ca atoms adsorption on C24N24, as well as the strong coupling between the electronic states in Fig. 2b, demonstrate that the Ca atoms are firmly bonded to the N atoms. As previously stated, the aggregation of coated Ca atoms may have a detrimental influence on CO2 storage. To address this issue, we compared the average Eads value of Ca atoms in Ca6C24N24 to the cohesive energy of bulk Ca (−1.82 eV per Ca atom). It is revealed that, due to higher average Eads, Ca atoms are firmly adsorbed on the N4 cavities of C24N24, avoiding the formation of Ca clusters on this cluster. This is also seen in Fig. 3, where the formation of a Ca6 cluster on C24N24 from Ca6C24N24 is highly endothermic by 23.01 eV. Such a result can be validated further by ab initio molecular dynamic (MD) simulations. The final structure of Ca6C24N24 following MD simulation at 500 K and for 2ps is depicted in Fig. S1 of the ESI.† The Ca atoms are clearly shown to be still located on the hollow sites of C24N24, and therefore the Ca6C24N24 stays stable after the MD simulations. As a result, Ca6C24N24 is stable enough to be employed as a CO2 storage material at ambient or even high temperatures.
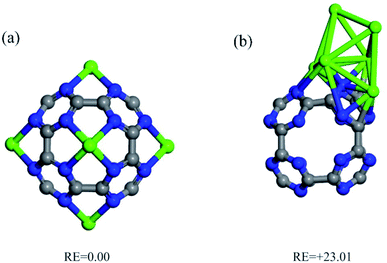 |
| Fig. 3 Two stable isomers and correspond relative energy (RE, in eV) of Ca6C24N24: (a) Ca atoms are disperse on N4 cavities of C24N24 and (b) Ca atoms form a Ca6 cluster on C24N24. | |
We investigated the adsorption of CO2 molecules on Ca coated clusters to examine if the Ca coating improves CO2 adsorption energy and storage on C24N24. First, consider CO2 molecule adsorption on the single Ca atom coated C24N24. Fig. 2c depicts the most stable geometry for CO2 adsorption on CaC24N24. Table 1 shows the associated adsorption energy (Eads), net charge transfer (QCT) and Hirshfeld charge on the Ca atom (QCa). The CO2 molecule is end-on on CaC24N24, with one of its O facing the Ca atom. The binding distance between the O atom of CO2 and Ca is 2.56 Å, and the associated adsorption energy is −0.48 eV. This low adsorption energy implies that the formed CO2@CaC24N24 complex is stable and that CO2 adsorption on CaC24N24 would be reversible. The adsorbed CO2 molecule, according to the Hirshfeld analysis, serves as a Lewis base since it supplies 0.17|e| to the nanocage. This is followed by a reduction in the atomic charge of the Ca from 0.74|e| in the bare CaC24N24 to 0.61|e| in the CO2@CaC24N24. This means that the transferred charge from CO2 is primarily concentrated on the Ca atom. Despite this, the EDD map in Fig. 2c indicates that the positively charged Ca atom polarizes the electron density on the interacting O atom of CO2, as indicated by a significant electron density gain region (pink color). According to the PDOS analysis, the highest occupied molecular orbital (HOMO) of CO2 is substantially coupled with the electronic states of Ca atom below the Fermi level, confirming our claim that CO2 acts as a Lewis base on CaC24N24.
Table 1 Calculated average adsorption energy (Eads, eV), binding distance (RCa–O, Å), net charge-transfer (QCT, electrons), Hirshfeld atomic charge on the Ca atom (QCa, |e|) of CO2 adsorbed configurations and the electron density (ρBCP, a.u.), its Laplacian (∇2ρBCP, a.u.) and total electronic energy density (HBCP, a.u.) at the Ca⋯OCO BCPs
Complex |
Eads |
RCa–O |
QCTa |
QCa |
ρBCP |
∇2ρBCP |
HBCP |
The QCT > 0 values show charge transfer from the CO2 to nanocage. |
1CO2@CaC24N24 |
−0.48 |
2.56 |
0.17 |
0.61 |
0.020 |
0.095 |
0.003 |
2CO2@CaC24N24 |
−0.47 |
2.58 |
0.15 |
0.51 |
0.019 |
0.093 |
0.003 |
3CO2@CaC24N24 |
−0.45 |
2.59 |
0.13 |
0.40 |
0.018 |
0.088 |
0.003 |
4CO2@CaC24N24 |
−0.42 |
2.65 |
0.12 |
0.37 |
0.015 |
0.072 |
0.003 |
5CO2@CaC24N24 |
−0.40 |
2.76 |
0.10 |
0.36 |
0.013 |
0.060 |
0.002 |
24CO2@Ca6C24N24 |
−0.40 |
2.73 |
0.02 |
0.39 |
0.014 |
0.063 |
0.002 |
To determine the maximum adsorption capacity of CaC24N24, more CO2 molecules are introduced to this cluster until saturation is reached. The relaxed structures of two, three, four and five CO2 molecules attached on the Ca atom of CaC24N24 are shown in Fig. 4. It is seen that all the newly added CO2 molecules adopt an end-on configuration on the Ca atom, but the corresponding average binding distance between the CO2 molecules and Ca atom becomes larger and larger as the number of the CO2 molecules increases (Table 1). Accordingly, the adsorption energy per CO2 molecule in 2CO2@CaC24N24, 3CO2@CaC24N24, 4CO2@CaC24N24 and 5CO2@CaC24N24 complexes is −0.47, −0.45, −0.42 and −0.40 eV, respectively. It is worth noting that the amount of change in adsorption energies caused by successive addition of CO2 molecules on CaC24N24 is less than that seen on CaC60.60 The reasons for the latter observation will be addressed latter. The average charge-transfer value and atomic charge on the Ca atoms also become smaller as the number of the CO2 molecules increases on the CaC24N24 cluster. Since CO2 molecules are positively charged as a result of donating electrons to the cluster, they repel each other, resulting in decreasing Eads and QCT values as the number of CO2 molecules increases. Furthermore, the average Hirshfeld charge on the Ca atom decreases progressively due to CO2 molecules addition. When the sixth CO2 molecule is added, we found that the average Eads value of CO2 molecule decreased to −0.33 eV, indicating that the maximum capacity for the adsorption of CO2 molecule on CaC24N24 is five.
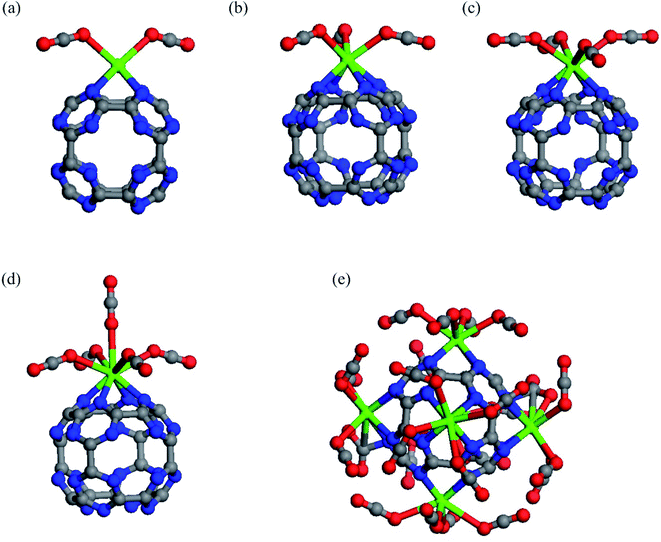 |
| Fig. 4 The optimized structure of the most stable configuration of (a) 2CO2@CaC24N24, (b) 3CO2@CaC24N24, (c) 4CO2@CaC24N24, (d) 5CO2@CaC24N24 and (e) 24CO2@Ca6C24N24 complexes. The color code of the atoms: Ca (green); O (red); C (gray); N (blue). | |
Based on these findings, we allowed five CO2 molecules to adsorb on each Ca atom of Ca6C24N24. Following the geometry optimizations, we found that each Ca atom of Ca6C24N24 can adsorb up to four CO2 molecules. According to Table 1, the Eads value per CO2 molecule in the resulting 24@Ca6C24N24 complexes is −0.40 eV, which is 17% less than the Eads value of a CO2 value on Ca@C24N24. The average charge-transfer value from Ca6C24N24 to CO2 molecules is 0.08|e|, indicating that CO2 molecules are activated on this cluster. Furthermore, the average Eads value of a CO2 molecule in the obtained 24@Ca6C24N24 (−0.40 eV) is within the range suggested for an ideal CO2 adsorbent (from −0.40 to −0.80 eV).73
3.3. Nature of Ca⋯CO2 interactions
Further information on the nature of the Ca⋯CO2 interactions may be gained by topological analysis of the electron density. The calculated ρBCP, ∇2ρBCP and HBCP values at the Ca⋯CO2 BCPs of different CO2 adsorbed complexes are listed in Table 1. Fig. S2† depicts the corresponding molecular graphs. The ρBCP values for all examined systems are small, indicating that the Ca⋯CO2 interactions in these systems are moderate. For the CaC24N24 fullerene, the tendency of electrons to localize at the Ca⋯CO2 BCPs decreases as the number of CO2 molecules on the Ca atom increases. For example, the average ρBCP value at the Ca⋯CO2 BCPs of 5CO2@CaC24N24 is 0.013 a.u., which is about 30% less than the value at 1CO2@CaC24N24. Given the linear relationship between bond strengths and ρBCP values at respective BCP,77 the Ca⋯CO2 interactions weaken as the number of CO2 molecules on the Ca atom increases. Remarkably, the molecular graphs of the complexes 4CO2@CaC24N24 and 5CO2@CaC24N24 exhibit BCPs between the C atoms of CO2 molecules and the C atoms of the fullerene, implying the formation of side interactions in these systems (Fig. S2†). This explains why the average adsorption energy of these systems decreases slowly as the number of CO2 molecules increases.
The ∇2ρBCP and HBCP values at the Ca⋯CO2 BCPs are positive in all complexes studied, indicating that CO2 adsorption is mainly mediated by electrostatic effects. This finding is explained by the coulombic interaction of positively charged Ca atoms and negative O atom in CO2. However, when the number of CO2 molecules increases over the Ca atom, the ∇2ρBCP and HBCP values decrease, suggesting that the contribution of electrostatic interactions in these systems are decreasing.
3.4. Selectivity
In addition to high capacity, a CO2 storage material should have high selectivity in order to separate CO2 molecules from other gases such as CH4, H2 and N2 present in natural gas or flue gas mixtures. So, in order to assess the selectivity of the Ca coated C24N24 cluster, we investigated the adsorption properties of CH4, H2, and N2 molecules. Fig. 5 depicts the most stable geometries of these molecules on CaC24N24, as well as their associated binding distances. Table 2 also summarizes the Eads and QCT values of these molecules. According to the results, all of the CH4, H2, and N2 molecules are weakly bound to the CaC24N24, as shown by their low Eads and QCT values. This means that when the Ca coated C24N24 is exposed to CO2/H2, CO2/CH4 and CO2/N2 mixtures, the CO2 molecules are preferentially adsorbed on the Ca atoms. As a result, Ca coated C24N24 can be considered as a selective adsorbent for CO2 separation from the aforementioned gas mixtures.
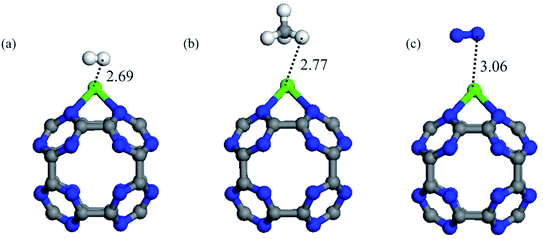 |
| Fig. 5 The optimized structure and relevant bond distances (in Å) of the most stable configuration of (a) H2, (b) CH4 and (c) N2 adsorbed on CaC24N24. | |
Table 2 Calculated adsorption energy (Eads, eV), net charge-transfer (QCT, electrons) and Hirshfeld atomic charge on the Ca atom (QCa, |e|) of H2, CH4 and N2 molecules adsorbed on CaC24N24
Complex |
Eads |
QCTa |
QCa |
The QCT > 0 values show charge transfer from the CO2 to nanocage. |
H2@CaC24N24 |
−0.14 |
0.08 |
0.67 |
CH4@CaC24N24 |
−0.34 |
0.17 |
0.60 |
N2@CaC24N24 |
−0.07 |
0.09 |
0.62 |
4. Conclusions
To summarize, the effect of Ca coating on the CO2 adsorption behaviors of porphyrin-like porous fullerene (C24N24) was thoroughly studied using the DFT calculations. A CO2 molecule may be reversibly adsorbed on Ca coated C24N24 with an adsorption energy of −0.48 eV, as opposed to bare C24N24. The Ca atoms are located on the center of the N4 cavity of C24N24 due to strong electrostatic interactions as well as charge transfer from the Ca atoms to the fullerene. Ca atoms do not aggregate and cluster on C24N24 because to the strong Ca–N interactions. Each Ca atom in the fully coated Ca6C24N24 fullerene may adsorb up to four CO2 molecules with an Eads value of −0.40 eV per CO2. This is within the recommended range for an ideal CO2 storage material. Furthermore, Ca coated C24N24 has a high potential for separating CO2 gas from flue gas or natural gas mixtures. These findings might inspire intensive experimental attempts to develop high-efficiency CO2 storage media.
Conflicts of interest
There are no conflicts to declare.
References
- H. W. Kroto, J. R. Heath, S. C. O'Brien, R. F. Curl and R. E. Smalley, Nature, 1985, 318, 162–163 CrossRef CAS.
- S. Iijima and T. Ichihashi, Nature, 1993, 363, 603–605 CrossRef CAS.
- S. Iijima, Phys. B, 2002, 323, 1–5 CrossRef CAS.
- K. S. Novoselov, A. K. Geim, S. V. Morozov, D.-e. Jiang, Y. Zhang, S. V. Dubonos, I. V. Grigorieva and A. A. Firsov, Science, 2004, 306, 666–669 CrossRef CAS PubMed.
- C. Berger, Z. Song, T. Li, X. Li, A. Y. Ogbazghi, R. Feng, Z. Dai, A. N. Marchenkov, E. H. Conrad and P. N. First, J. Phys. Chem. B, 2004, 108, 19912–19916 CrossRef CAS.
- A. E. Mahdy, Mol. Phys., 2015, 113, 3531–3544 CrossRef.
- C. Ramos-Castillo, J. Reveles, R. Zope and R. De Coss, J. Phys. Chem. C, 2015, 119, 8402–8409 CrossRef CAS.
- C. Ramos-Castillo, J. Reveles, M. Cifuentes-Quintal, R. Zope and R. de Coss, J. Phys. Chem. C, 2016, 120, 5001–5009 CrossRef CAS.
- F. Bottacchi, L. Petti, F. Späth, I. Namal, G. Tröster, T. Hertel and T. D. Anthopoulos, Appl. Phys. Lett., 2015, 106, 51–52 CrossRef.
- X. Wang, G. Li, G. Hong, Q. Guo and X. Zhang, ACS Appl. Mater. Interfaces, 2017, 9, 41323–41331 CrossRef CAS PubMed.
- T. Ye, Y. Sun, X. Zhao, B. Lin, H. Yang, X. Zhang and L. Guo, J. Mater. Chem. A, 2018, 6, 18994–19003 RSC.
- L. Bai, Y. Lei, H. Huang and Y. Xiang, ACS Appl. Mater. Interfaces, 2020, 12, 33139–33151 CrossRef CAS PubMed.
- P. Su, K. Iwase, S. Nakanishi, K. Hashimoto and K. Kamiya, Small, 2016, 12, 6083–6089 CrossRef CAS PubMed.
- I. H. Lin, Y. H. Lu and H. T. Chen, J. Comput. Chem., 2017, 38, 2041–2046 CrossRef CAS PubMed.
- M. D. Esrafili, Chem. Phys. Lett., 2018, 705, 44–49 CrossRef CAS.
- Y. Zhang, D. Zhang and C. Liu, J. Phys. Chem. B, 2006, 110, 4671–4674 CrossRef CAS PubMed.
- Y. Chen, B. Gao, J.-X. Zhao, Q.-H. Cai and H.-G. Fu, J. Mol. Model., 2012, 18, 2043–2054 CrossRef CAS PubMed.
- J. N. Gavgani, A. Hasani, M. Nouri, M. Mahyari and A. Salehi, Sens. Actuators, B, 2016, 229, 239–248 CrossRef CAS.
- L.-F. Velázquez-López, S.-M. Pacheco-Ortin, R. Mejía-Olvera and E. Agacino-Valdés, J. Mol. Model., 2019, 25, 1–11 CrossRef PubMed.
- L. Ferrighi, M. I. Trioni and C. Di Valentin, J. Phys. Chem. C, 2015, 119, 6056–6064 CrossRef CAS.
- H. Miao, S. Li, Z. Wang, S. Sun, M. Kuang, Z. Liu and J. Yuan, Int. J. Hydrogen Energy, 2017, 42, 28298–28308 CrossRef CAS.
- M. D. Esrafili and S. Asadollahi, Appl. Surf. Sci., 2018, 463, 526–534 CrossRef.
- P. Sun, H. Liu, M. Feng, Z. Zhai, Y. Fang, X. Zhang and V. K. Sharma, Appl. Catal., B, 2020, 272, 119005 CrossRef CAS.
- J. Guo, S. Zhang, M. Zheng, J. Tang, L. Liu, J. Chen and X. Wang, Int. J. Hydrogen Energy, 2020, 45, 32402–32412 CrossRef CAS.
- X. Zhang, Z. Lu and Z. Yang, J. Mol. Catal. A: Chem., 2016, 417, 28–35 CrossRef CAS.
- M. Wang and Z. Wang, RSC Adv., 2017, 7, 48819–48824 RSC.
- X. Zhang, X. Xie, H. Wang, J. Zhang, B. Pan and Y. Xie, J. Am. Chem. Soc., 2013, 135, 18–21 CrossRef CAS PubMed.
- Z. Jin and L. Zhang, J. Mater. Sci. Technol., 2020, 49, 144–156 CrossRef.
- V. Tripkovic, M. Vanin, M. Karamad, M. r. E. Björketun, K. W. Jacobsen, K. S. Thygesen and J. Rossmeisl, J. Phys. Chem. C, 2013, 117, 9187–9195 CrossRef CAS.
- Z. Wang, J. Zhao and Q. Cai, Phys. Chem. Chem. Phys., 2017, 19, 23113–23121 RSC.
- E. Ashori, F. Nazari and F. Illas, Phys. Chem. Chem. Phys., 2017, 19, 3201–3213 RSC.
- D. H. Lee, W. J. Lee, W. J. Lee, S. O. Kim and Y.-H. Kim, Phys. Rev. Lett., 2011, 106, 175502 CrossRef PubMed.
- J. Zeng and K.-Q. Chen, Appl. Phys. Lett., 2014, 104, 033104 CrossRef.
- H. Hamadi, E. Shakerzadeh and M. D. Esrafili, Chem. Phys. Lett., 2019, 724, 80–85 CrossRef CAS.
- E. Shakerzadeh, H. Hamadi and M. D. Esrafili, Inorg. Chem. Commun., 2019, 106, 190–196 CrossRef CAS.
- K. Srinivasu and S. K. Ghosh, J. Phys. Chem. C, 2012, 116, 25184–25189 CrossRef CAS.
- M. D. Esrafili and B. Nejadebrahimi, Chem. Phys. Lett., 2019, 716, 11–16 CrossRef CAS.
- B. Modak, K. Srinivasu and S. K. Ghosh, Int. J. Hydrogen Energy, 2017, 42, 2278–2287 CrossRef CAS.
- M. D. Esrafili and H. Hamadi, Mol. Phys., 2020, 118, e1797919 CrossRef.
- A. Tatar, A. Barati-Harooni, A. Najafi-Marghmaleki, A. Mohebbi, M. M. Ghiasi, A. H. Mohammadi and A. Hajinezhad, Int. J. Greenhouse Gas Control, 2016, 53, 85–972016 CrossRef CAS.
- I. G. Wilson and I. Staffell, Nat. Energy, 2018, 3, 365–372 CrossRef CAS.
- T. Montanari, E. Finocchio, E. Salvatore, G. Garuti, A. Giordano, C. Pistarino and G. Busca, Energy, 2011, 36, 314–319 CrossRef CAS.
- J. O. Abe, A. Popoola, E. Ajenifuja and O. Popoola, Int. J. Hydrogen Energy, 2019, 44, 15072–15086 CrossRef CAS.
- J. Bradshaw, S. Bachu, D. Bonijoly, R. Burruss, S. Holloway, N. P. Christensen and O. M. Mathiassen, Int. J. Greenhouse Gas Control, 2007, 1, 62–68 CrossRef CAS.
- F. D. Meylan, V. Moreau and S. Erkman, J. CO2 Util., 2015, 12, 101–108 CrossRef CAS.
- M. S. Duyar, S. Wang, M. A. Arellano-Trevino and R. J. Farrauto, J. CO2 Util., 2016, 15, 65–71 CrossRef CAS.
- P. Kelemen, S. M. Benson, H. Pilorgé, P. Psarras and J. Wilcox, Front. Clim., 2019, 1, 9 CrossRef.
- F. Su, C. Lu, S.-C. Kuo and W. Zeng, Energy Fuels, 2010, 24, 1441–1448 CrossRef CAS.
- S. Kumar, R. Srivastava and J. Koh, J. CO2 Util., 2020, 41, 101251 CrossRef CAS.
- R. Babarao and J. Jiang, Energy Environ. Sci., 2008, 1, 139–143 RSC.
- Y. Zeng, R. Zou and Y. Zhao, Adv. Mater., 2016, 28, 2855–2873 CrossRef CAS PubMed.
- W. Leitner, Coord. Chem. Rev., 1996, 153, 257–284 CrossRef CAS.
- G. Qin, Q. Cui, W. Wang, P. Li, A. Du and Q. Sun, ChemPhysChem, 2018, 19, 2788–2795 CrossRef CAS PubMed.
- N. Sathishkumar, S.-Y. Wu and H.-T. Chen, Chem. Eng. J., 2020, 391, 123577 CrossRef CAS.
- A. Liu, J. Long, S. Yuan, W. Cen and J. Li, Phys. Chem. Chem. Phys., 2019, 21, 5133–5141 RSC.
- C. Chen, K. Xu, X. Ji, L. Miao and J. Jiang, Phys. Chem. Chem. Phys., 2014, 16, 11031–11036 RSC.
- M. D. Esrafili, J. Mol. Graphics Modell., 2019, 90, 192–198 CrossRef CAS PubMed.
- N. Sathishkumar, S.-Y. Wu and H.-T. Chen, Chem. Eng. J., 2021, 407, 127194 CrossRef CAS.
- C. He, R. Wang, D. Xiang, X. Li, L. Fu, Z. Jian, J. Huo and S. Li, Appl. Surf. Sci., 2020, 509, 145392 CrossRef CAS.
- B. Gao, J.-x. Zhao, Q.-h. Cai, X.-g. Wang and X.-z. Wang, J. Phys. Chem. A, 2011, 115, 9969–9976 CrossRef CAS PubMed.
- G. Gao, F. Ma, Y. Jiao, Q. Sun, Y. Jiao, E. Waclawik and A. Du, Comput. Mater. Sci., 2015, 108, 38–41 CrossRef CAS.
- J. P. Perdew, K. Burke and M. Ernzerhof, Phys. Rev. Lett., 1996, 77, 3865–3868 CrossRef CAS PubMed.
- B. Delley, J. Chem. Phys., 1990, 92, 508–517 CrossRef CAS.
- B. Delley, J. Chem. Phys., 2000, 113, 7756–7764 CrossRef CAS.
- S. Grimme, J. Comput. Chem., 2006, 27, 1787–1799 CrossRef CAS PubMed.
- R. Bader, Atoms in Molecules: A Quantum Theory, Clarendon Press, Oxford, 1990 Search PubMed.
- I. Rozas, I. Alkorta and J. Elguero, J. Am. Chem. Soc., 2000, 122, 11154–11161 CrossRef CAS.
- M. Frisch, G. Trucks, H. Schlegel, G. Scuseria, M. Robb, J. Cheeseman, G. Scalmani, V. Barone, B. Mennucci and G. Petersson, Gaussian Inc., Wallingford CT, 2009.
- F. Biegler-König and J. Schönbohm, J. Comput. Chem., 2002, 23, 1489–1494 CrossRef PubMed.
- E. Shakerzadeh, Z. Mashak Shabavi and E. C. Anota, Appl. Organomet. Chem., 2020, 34, e5694 CrossRef CAS.
- Q. Sun, M. Wang, Z. Li, A. Du and D. J. Searles, J. Phys. Chem. C, 2014, 118, 2170–2177 CrossRef CAS.
- H. Dong, B. Lin, K. Gilmore, T. Hou, S.-T. Lee and Y. Li, Curr. Appl. Phys., 2015, 15, 1084–1089 CrossRef.
- S. Chu and A. Majumdar, Nature, 2012, 488, 294 CrossRef CAS PubMed.
- D. C. Camacho-Mojica, E. Muñoz-Sandoval and F. López-Urías, Carbon, 2017, 116, 381–390 CrossRef.
- E. Shakerzadeh, H. Hamadi and M. D. Esrafili, Inorg. Chem. Commun., 2019, 106, 190–196 CrossRef CAS.
- P. Mousavian and M. D. Esrafili, Inorg. Chem. Commun., 2020, 122, 108317 CrossRef CAS.
- M. A. Spackman, Cryst. Growth Des., 2015, 15, 5624–5628 CrossRef CAS.
Footnote |
† Electronic supplementary information (ESI) available. See DOI: 10.1039/d1ra05888f |
|
This journal is © The Royal Society of Chemistry 2021 |