DOI:
10.1039/D1RA05030C
(Paper)
RSC Adv., 2021,
11, 32119-32125
Hybrid compound based on diethylenetriaminecopper(II) cations and scarce V-monosubstituted β-octamolybdate as water oxidation catalyst†
Received
29th June 2021
, Accepted 8th September 2021
First published on 29th September 2021
Abstract
Herein, we report on a new hybrid compound (NH4){[Cu(dien)(H2O)2]2[β-VMo7O26]}·1.5H2O (1), where dien = diethylenetriamine, containing an extremely rare mixed-metal pseudo-octamolybdate cluster. An ex situ EPR spectroscopy provided insights into the formation of paramagnetic species in reaction mixture and in solution of 1. The magneto-structural correlations revealed weak antiferromagnetic exchange interactions between the [Cu(dien)]2+ cations transmitted by intermolecular pathways. The cyclic voltammetry showed the one-electron process associated with the Cu3+/Cu2+ oxidation followed by the multi-electron catalytic wave due to water oxidation with a faradaic yield of 86%. The title compound was thus employed in homogeneous water oxidation catalysis using tris(bipyridine)ruthenium photosensitizer. At pH 8.0, efficiency of the catalytic system attained 0.19 turnovers per second supported by the relatively mild water oxidation overpotential of 0.54 V.
1 Introduction
Polyoxometalates (POMs) are a well-known class of inorganic compounds, which has attracted attention of chemists for years due to their different archetypes, structural flexibilities and functionalities. There have been recently several successful attempts aimed at their decoration with complex cations to obtain hybrid organic–inorganic materials.1 This strategy may result in formation of products with new promising properties superior to those inherent for the starting building blocks.2 Another interesting modification of such compounds is a combination of different metal centers in one POM, as for example, in mixed-metal Mo/V anions. It can be used as one of the promising ways to obtain new prospective materials. There is more versatility of Mo-containing polyoxoanion forms compared to polyoxovanadates, and the former usually have acidic properties. At the same time V-based anions are found to be more active in redox processes.3,4 Mo/V anions usually have the structural types characteristic for polyoxomolybdates and the introduction of easily-reducible V-centers into a POM may change the nature of products from purely acidic to redox. Moreover, the redox properties can be tuned by the number of V-centers in the clusters.5 The mixed-metal Mo/V POMs are mainly represented in the literature by the following types of anions: disubstituted α-octamolybdate form [α-V2Mo6O26]6−,6–8 pseudo-Keggin [Mo8V7O42]7− and Keggin-based structures [VMo12O40]3−, [VMo8V6O42]7−,8–10 [VMo6O22]3− (ref. 11 and 12) and Linqwist [VMo5O19]3− anion.13 One the most common Mo/V POM among the mentioned above anions is α-octamolybdate [α-V2Mo6O26]6−. Also, a hybrid compound based on the disubstituted β-octamolybdate [β-V2Mo6O26]6− and Gd complex has been recently reported.14
There were only two publications on a new monosubstituted variant of the β-octamolybdate cluster, [β-VMo7O26]5−. The first compound with this anion was reported in 2018. It consisted of a protonated isoniazid dimer, potassium, sodium cations, [β-VMo7O26]5− cluster and water molecules.15 The hybrid had semiconducting properties. Another compound featuring this rare anion was reported in 2019; it was purely inorganic, with the charge of the cluster compensated by potassium cations.16
Catalytic properties of POMs have been in a focus of both early studies and the most recent developments of hybrid organic–inorganic materials.17 Compared to molecular catalysts, POMs have distinctive properties as, for example, tunable acidity and rich redox chemistry, inherent resistance to oxidative decomposition, high thermal stability, and possibility for photosensitization.18 Large-scale industrial applications of POMs include alkenes hydration19 and oxidation,20 ketones amination,21 and esterification processes.22 The applications of POM-based materials to solar fuels production, is becoming a new strategy in the field of artificial photosynthesis. Various POMs have been examined in different functional roles for solar fuel generation, including photosensitizers, catalysts and charge transfer relays.23–27
This paper is a continuation of our research devoted for the synthesis of hybrid compounds based on POMs under mild conditions.3,4,28–30 Our work is focused on the study of influence of synthetic conditions such as the stoichiometry of reagent, metal sources in the reaction mixtures and the time of interaction. Previously we have successfully synthesized organic–inorganic hybrid compounds with Mo/V anions based on mixed-metal α-octamolybdate [α-V2Mo6O26]6− and Keggin [PMo8V4O40]7− anions.28,30 Herein, we report new compound (NH4){[Cu(dien)(H2O)2]2[β-VMo7O26]}·1.5H2O (1) based on monosubstituted β-octamolybdate anion. The obtained 1 is a first example of compound based on extremely rare [β-VMo7O26]7− anion with Cu-containing complex cations.
2 Experimental
2.1 Synthesis of (NH4){[Cu(dien)(H2O)2]2[β-VMo7O26]}·1.5H2O (1)
All reagents were of analytical grade and used as purchased without further purification. The mixture of Cu powder (0.025 g, 0.40 mmol), VOSO4 (0.239 g, 1.20 mmol) and (NH4)6Mo7O24·4H2O (0.847 g, 0.685 mmol) was placed in 50 mL conical flask and 15 mL of water was poured into the solid reagents. Diethylenetriamine (dien, 0.043 mL, 0.40 mmol) was then added under vigorous stirring. The reaction mixture was mechanically stirred at 60–65 °C in open system with air access for 2.5 h and total dissolution of metal powder was observed. The resulting hot solution was filtered and cooled down to the room temperature. Slow evaporation of the filtrate (pH = 4.98) yielded blue crystals of 1 in a day. Yield 0.15 g (47% by copper). Anal. Calc./found for Cu2VMo7O31,5C8N7H41: C – 6.05/6.22; H – 2.60/2.24; N – 6.17/6.00%.
2.2 Crystallography
Single crystal diffraction data was obtained on an Xcalibur-3 diffractometer using Mo-Kα radiation (λ = 0.71073 Å) at 298 K. Empirical absorption correction was provided with a multi-scan method using spherical harmonics, implemented in the SCALE3 ABSPACK scaling algorithm of the CrysAlisPro program package.31 Structure was solved by direct methods and refined against F2 within anisotropic approximation for all non-hydrogen atoms using the OLEX2 (ref. 32) program package with SHELXT and SHELXL modules.33,34 All H atoms were placed in idealized positions (C–H = 0.97 Å, N–H = 0.85–0.89 Å, O–H = 0.84–0.91 Å) and constrained to ride on their parent atoms, with Uiso = 1.2 Ueq. (except Uiso = 1.5 Ueq. for hydroxyl groups). Final refinement was converged at R1 = 0.0573, wR2 = 0.1721, GOF = 1.054. Atom coordinates and crystallographic parameters have been deposited to the Cambridge Crystallographic Data Centre (CCDC 1958821).
2.3 Magnetic susceptibility measurements
The DC magnetic susceptibility was recorded using the SQUID magnetometer (MPMS, Quantum Design) calibrated with a palladium rod (Materials Research Corporation, purity 99.9985%). The crystalline sample (ca. 11 mg) was powdered in order to prevent its displacement in the magnetic field. The susceptibility data was acquired at the field of 0.5 T in the temperature range from 1.8 to 300 K. The susceptibility was corrected in respect to the background signals and underlying diamagnetism and transformed to the effective magnetic moment. The field-dependent magnetization measurements were carried out at T = 2.0 K in the magnetic field range up to 5 T. No remnant magnetization was detected.
2.4 Water oxidation studies
Water oxidation experiments were carried out in a 1.5 mL cuvette containing 1.0 mL of the aqueous catalytic mixture. The content of the mixture was as follows: 4 μM of the title complex, 0.1 mM of {[Ru(bpy)3](ClO4)2}·6H2O, 2 mM of Na2S2O8 in borate buffer (0.04 M, pH = 8.0). In the reference experiment, 8 μM solution of CuCl2·2H2O or [Cu(dien)](ClO4)2 were used instead of the title complex with other components being in identical quantities. Oxygen evolution was detected polarographically by using a Clark-type electrode separated from the catalytic mixture by a Teflon membrane. Air-saturated distilled water ([O2]20 °C = 276 μm) was used for calibration of the electrode. The cell was purged with argon gas before each experiment, and the solution in the cell was continuously stirred. Blue light LEDs (λ = 450 nm, 3 W) were used as illumination sources. The TOF and TON were calculated based on the total number of copper centres in compounds. TOF = number of O2 molecules evolved per second per one Cu ion at the maximum rate of oxygen evolution (mol(O2) mol(Cu)−1 s−1); TON = total number of O2 molecules per one Cu atom evolved by the moment of time, when reaction rate decreased to zero (mol(O2) mol(Cu)−1). All experiments were repeated three times to obtain reproducible TON and TOF values.
2.5 Electrochemistry
Cyclic voltammograms were recorded using an electrochemical three-electrode cell with a 3 mm glass carbon working electrode, a glassy carbon rod counter electrode, and an Ag/AgCl in saturated KCl aqueous solution reference electrode (E = +0.195 vs. NHE). The counter and reference electrodes were in compartments separated from the bulk solution by fritted discs. The working electrode was polished with 0.05 μm alumina paste and sonicated before use. The solution under study (5 mL) contained the title complex (0.25 mM), borate buffer (0.04 M, pH 8.0) and KClO4 (0.1 M) as a supporting electrolyte. Before all measurements, oxygen was removed from the cell by bubbling argon through the stirred solutions. Samples were kept under argon during the measurements. Controlled potential electrolysis experiments were carried out in a three-electrode cell (ITO glass working electrode, Pt counter electrode and a miniature Ag/AgCl reference electrode) equipped with a Clark-type electrode for dissolved oxygen measurements.
2.6 Other methods
CHN analysis was performed using a Vario El Cube elemental analyzer. Infrared (IR) spectrum was recorded in the range of 400–4000 cm−1 in KBr pellets using a PerkinElmer Spectrum BX spectrometer. The EPR spectra were recorded on Bruker ELEXSYS E500 CW-EPR spectrometer operating at X-band equipped with frequency counter and NMR Teslameter.
3 Results and discussion
Hybrid compound based on mixed-metal Mo/V polyoxoanion was obtained from reaction system Cu0 – dien – (NH4)6Mo7O24 – VOSO4 – H2O in the course of the one-pot synthesis accompanied by air oxidation. In contrast to previously studied system with V2O5 as a source of vanadium,28 this procedure yielded another type of POM, namely, the rare mixed-metal [β-VMo7O26]5− anion. The advantage of the use of metallic Cu as the source of Cu cations is the anion deficiency conditions during the synthesis resulting in the formation and crystallization of hybrid compound with negatively charged POM.
Compound 1 has cationic–anionic structure containing two complex cations [Cu(dien)(H2O)2]2+, ammonium, and V-substituted [β-VMo7O26]5− polyoxoanion (Fig. 1); the crystal also contains crystallization water molecules. The POM is based on a common β-octamolybdate structure type, however, one of the Mo centers is substituted by V. All metal centers (V and Mo) have distorted octahedral oxygen surrounding. The most distorted are coordination polyhedra at the Mo(1)/V(1) centers (M–O bond lengths vary in the range of 1.651–2.396, while all other polyhedra possess the range of 1.699–2.326 Å). Generally the geometrical parameters for the anion are close to those published previously.15,16 The coordination polyhedron of CuII center is formed by three nitrogen atoms from the dien molecule and two oxygen atoms from the coordinated H2O. The value of the angular structural index parameter τ is 0.21 [τ = (β − α)/60°, α and β are the two largest valence angles centred on the same Cu atom, α < β].35 Thus, the [CuN3O2] coordination polyhedron has distorted square-based pyramidal geometry.
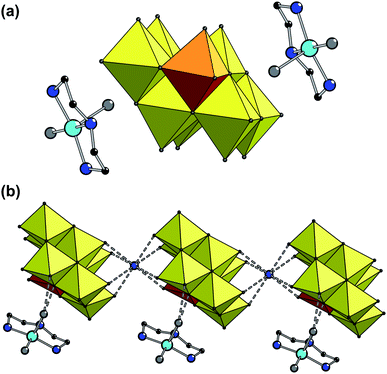 |
| Fig. 1 Mixed polyhedral and ball-and-stick representation of the structure of 1 (a) and chains parallel to (100) direction in 1 (b). Color code: Cu, cyan, Mo yellow, V orange, O grey, N blue, C black. All of the hydrogen atoms and crystallization water molecules are omitted for clarity. Hydrogen bonds are shown as dashed lines. | |
In the crystal, complex anions alternate with ammonium forming chains along the (100) crystallographic direction by the means of charge-assisted N–H⋯O′ hydrogen bonds. Each complex cation is linked to the one of the POM anions by N–H⋯O′ and O–H⋯O′ hydrogen bonds involving the hydrogen atoms from dien and water ligands. Interactions of the complex cations with POM anions from other chains are rather weak. Chains are linked via the bridging coordinated water molecules that connect the amino group of the dien ligand of the cation from one chain with the water ligand of the cation from a neighboring chain, forming the network.
The IR spectrum of 1 (Fig. S1, ESI†) is different from a typical one for non-substituted β-octamolybdate anion,36,37 but has a similar pattern with other compounds based on [β-VMo7O26]5−.15,16 The most intensive bands at 930, 900 and 870 cm−1 are assigned to M = O stretching (M = Mo, V), while the bands at the region 850–470 cm−1 correspond to asymmetric and symmetric deformation vibrations of the M–O–Mo bonds. The characteristic bands attributed to water molecules (O–H) and coordinated dien ligands (N–H) are in the 3500–2800 cm−1 region.28
In order to understand the paramagnetic species transformation in the synthesis of 1, we compare EPR spectra of the reaction mixture obtained after dissolution of metallic copper with the aqueous solution of as-prepared 1. Two types of paramagnetic species in the frozen filtrate of reaction mixture (Sol1 in Fig. 2) were determined by EPR spectroscopy in the current system.
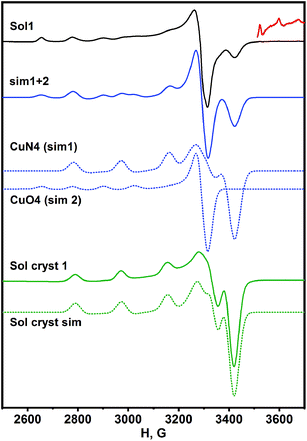 |
| Fig. 2 EPR spectra of frozen aqueous solutions (77 K) of the reaction mixture (Sol1), the title compound (Sol cryst 1), and the corresponding simulated spectra for the copper(II) species with the EPR parameters given in the text. The fragment of the magnified spectrum of Sol1 featuring V(IV) is shown in red. | |
The spin-Hamiltonian parameters determined by the simulation of the experimental spectrum are as follows: g‖ = 2.230, A‖ = 194 × 10−4 cm−1 and g‖ = 2.410, A‖ = 138 × 10−4 cm−1. The values are in agreement with those reported previously for Cu(II) complexes with N4 and O4 atoms in an equatorial plane, respectively.38 The additional weak lines observed in the spectrum at the high magnetic field were assigned to the hyperfine splitting of parallel orientation of oxovanadium(IV) species indicating that insignificant quantities of V(IV) remained after the interaction. In contrast, the EPR spectrum of the aqueous solution of crystals of 1 revealed only one type of copper centers with g‖ = 2.232, A‖ = 183 × 10−4 cm−1 that is in accordance with N3O equatorial surrounding of metal ions (Sol cryst 1 in Fig. 2). The difference between Sol1 and Sol cryst 1 indicated the rearrangement of complex units during crystallization. Thus, similar copper cationic complexes exist in the solid state and in the aqueous solution of 1. There was no residual V(IV) found in the solution of 1.
The magnetic properties of 1 recorded in the temperature range 1.8–300 K are shown in Fig. 3. The product of the molar magnetic susceptibility with temperature, χMT (and the effective magnetic moment) slowly decreases on cooling from room temperature down to 40 K; χMT (300 K) = 1.00 cm3 mol−1 K (μeff = 2.83 μB) is adequate to expected for two Cu(II) ions (S = ½ each) without any exchange interactions with gav = 2.00.
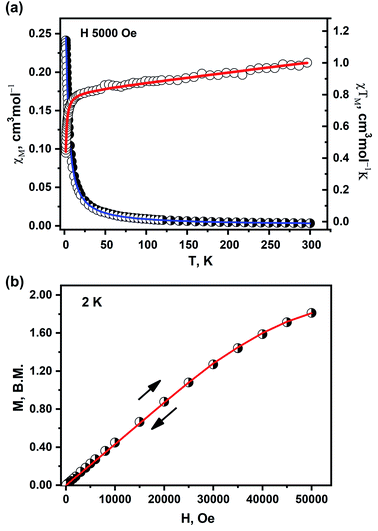 |
| Fig. 3 DC magnetic data for 1. (a) Temperature dependence of χM (half-open circles) and χMT (open circles). (b) Dependence of the magnetic moment on the external magnetic field. The solid lines on both graphs are calculated using the HDVV spin Hamiltonian and PHI program.39 | |
A rapid decrease of the χMT below 40 K is observed down to 0.43 cm3 mol−1 K at 1.8 K. The susceptibility behavior indicates antiferromagnetic exchange interactions between the copper ions in 1. As a local maximum of the χMT(T) function is not seen in the temperature range 1.8–300 K, the magnitude of such interaction is rather small.40
The exchange interaction between two Cu(II) ions (SA = SB = ½) in the title compound was described by the model of binuclear units realized through Cu⋯Cu intermolecular contacts. The calculations were based on the Heisenberg–Dirac–Van Vleck Hamiltonian in zero field given by eqn (1). The temperature independent paramagnetism (TIP) and the fraction of paramagnetic impurities (x) were also included into the fitting procedure.
The best agreement with the experimental magnetic data for 1 was obtained with the magnetic exchange parameter J = −0.93 cm−1, g = 2.09, and x = 0.005, TIP = 334 × 10−6 cm3 mol−1, R = Σ[(χT)exp − (χT)calc.]2/Σ[(χT)exp]2 = 7.1 × 10−5 (red and blue lines in Fig. 3a). The value of the J parameter confirms that the exchange interaction between the nearest Cu(II) centers in the examined complex is antiferromagnetic in nature but weak and can be attributed to the presence of the superexchange pathway through the long Cu⋯Cu contacts.
Detailed examination of the crystal packing of the complex 1 suggests that such weak magnetic coupling may be transmitted through double nonbonding Cu–O⋯O–Cu contact with distance d = 4.265 Å or 5.860 Å and angle of 97.8° or 94.7° (far from the optimal angle 180° typical for strong interaction)41,42 with the alternating Cu–Cu separations of 6.059 Å and 6.944 Å. (Fig. S2, ESI†). Additionally, the magnetic interactions through a longer Cu⋯Cu distance (7.682 Å) can be supported by N–H⋯O′ and O–H⋯O′ hydrogen bonds involving the hydrogen atoms from dien and water ligands.
The magnetization vs. field dependence recorded and 2.0 K did not reach a saturation plateau (Fig. 3b). In such case, the ground state has S = 1 and the magnetization per a formal Cu2 unit should saturate to the value of 2.0 μB at field above 5 T.
The catalytic activity of the title compound in water oxidation was evaluated using tris(bipyridine)ruthenium(II) light harvester and sodium persulfate as a sacrificial electron acceptor (Fig. S3, ESI†).43,44 The water-based catalytic mixture buffered by H3BO3–NaOH (pH 8.0) was driven by the visible light (3 W LEDs, λ = 450 nm) to excite the photosensitizer:
|
[Ru(bpy)3]2+ + hν → [Ru(bpy)3]2+*
| (2) |
The excited triplet state of the photosensitizer is rapidly quenched by persulfate giving [Ru(bpy)3]3+ and sulfate radical (eqn (3)), which then reacts with the second equivalent of the light harvester:
|
[Ru(bpy)3]2+* + S2O82− → [Ru(bpy)3]3+ + SO42− + SO4˙−
| (3) |
|
[Ru(bpy)3]2+ + SO4˙− → [Ru(bpy)3]3+ + SO42−
| (4) |
The photochemical generation of [Ru(bpy)3]3+ can be observed by steady-state UV-vis spectroscopy (Fig. S4, ESI†). When the copper complex is present in the reaction mixture, no accumulation of [Ru(bpy)3]3+ is observed because of the fast hole transfer to the catalyst with the subsequent oxidation of water. Using the Clark-type electrode, we have observed oxygen evolution with turnover number (TON, number of O2 molecules evolved per one copper atom) of 10 and turnover frequency (TOF) of 0.19 s−1 (Fig. 4). Thus, the catalytic activity of the title complex is similar to that reported for Cu-containing catalysts with [α-V2Mo6O26] (POM).28 Importantly, efficiency of the title complex is considerably higher compared to the plane Cu2+ salt under virtually identical photocatalytic conditions. For CuCl2 used as a catalyst, we were able to observe ∼2.5 O2 molecules produced per one copper.
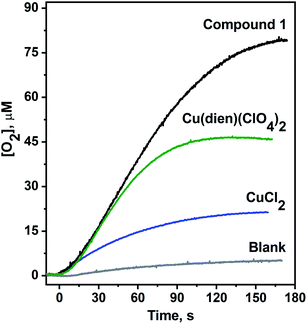 |
| Fig. 4 Oxygen evolution traces obtained for the title complex (4 μM, black curve), [Cu(dien)](ClO4)2 (8 μM, green curve) and CuCl2 (8 μM, blue curve) at pH 8.0. Oxygen evolution in the absence of any copper is shown in gray. Zero on the timescale corresponds to the start of illumination with LEDs (450 nm). | |
However, when a [Cu(dien)]2+ complex was used as a catalyst, the catalytic system reached the TON of 6 (green trace in Fig. 4), suggesting a pivotal role of the dien ligand in water oxidation catalysis via e.g. stabilization of a presumable CuIII intermediate.45,46
The electrochemistry of the title compound was studied in the buffered aqueous solution at pH 8.0 — conditions resembling our photochemical water oxidation experiment. At various scan rates from 10 to 1000 mV s−1, two oxidative waves can be identified (Fig. 5). The first one centered around 1.2 V vs. NHE is the one-electron process that can be assigned to the CuII/CuIII redox couple.47 The second wave with onset around 1.3 V is a multi-electron process corresponding to the electrocatalytic water oxidation. Thus, water oxidation using the title compound starts at a relatively mild overpotential 0.54 V compared to other WOCs.48,49 To understand the role of the ligand environment on the redox chemistry of copper in 1, we recorded cyclic voltammograms for the related complex [Cu(dien)](ClO4)2 (Fig. S5, ESI†). Both compounds exhibit similar oxidation processes up to 1.7 V vs. NHE indicating that the POM fragment in 1 is virtually redox innocent in this potential range. The peak current for the catalytic wave depends linearly on the square root of the scan rate (Fig. 5, inset) indicating a diffusion controlled homogeneous catalysis. The observation rules out a possible contribution of copper oxide precipitate to the catalytic current. Assignment of this oxidation process to the water oxidation was confirmed by controlled potential electrolysis experiments, coupled to oxygen evolution measurements using a Clark-type electrode. When a potential of 1.55 V vs NHE was applied, a stationary current of 0.063 mA was detected with a simultaneous dissolved oxygen increase of 8.5 μM min−1, corresponding to the overall faradaic yield of 86% (Fig. 6). This value indicates that oxidation of the ligand may occur slowly as a side reaction, but not as a dominant redox process at this potential.45,46
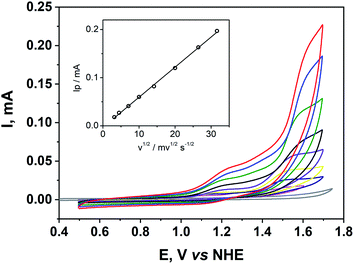 |
| Fig. 5 Cyclic voltammograms for the title complex recorded at different scan rates between 10 mV s−1 (magenta) and 1000 mV s−1 (red) at pH 8.0. The background current recorded at 100 mV s−1 is shown in gray. Inset shows dependence of the peak current at 1.6 V vs. NHE on the square root of the scan rate. | |
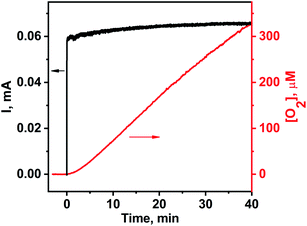 |
| Fig. 6 Electric current (black) and oxygen evolution trace (red) for the bulk electrolysis experiment using the title complex (0.1 mM) at pH 8.0. | |
After 45 min of sustained bulk electrolysis, the working electrode was recovered and immersed in the catalyst-free buffered solution. At the same potential of 1.55 V vs. NHE, the initial current of ca. 5 mA was observed accompanied by a negligible amount of oxygen evolved (Fig. S6, ESI†), which corroborates the absence of catalytically active precipitates on the working electrode surface.
4 Conclusions
In summary, we report synthesis of a novel hybrid compound based on the extremely rare mixed-metal polyoxoanion [β-VMo7O26]. The compound was obtained under mild conditions starting from zero-valent copper. The equilibrium of CuN4 and CuO4 species in the reaction mixture was established by EPR spectroscopy, while the solution of the final product 1 contained CuN3O species only. The oxidative process V(IV) → V(V) was almost completed till the end of the interaction while the crystalline 1 contained V solely in its highest oxidation state according to the magnetization studies. The prominent oxidative wave in cyclic voltammograms with onset at 1.3 V vs. NHE corresponded to electrocatalytic water oxidation which occurred under diffusion control. The efficiency of the catalytic system with the title compound in homogeneous water oxidation catalysis attained 0.19 turnovers per second supported by the relatively low water oxidation overpotential of 0.54 V.
Conflicts of interest
There are no conflicts to declare.
Acknowledgements
SIS gratefully thanks Dr Gustav Berggren for kind hosting and the Swedish Institute for support (grant no. 23913/2017).
Notes and references
- D. Li, P. Ma, J. Niu and J. Wang, Coord. Chem. Rev., 2019, 392, 49–80 CrossRef CAS.
- N. Lotfian, M. M. Heravi, M. Mirzaei and B. Heidari, Appl. Organomet. Chem., 2019, 33, e4808 CrossRef.
- M. V. Pavliuk, E. Mijangos, V. G. Makhankova, V. N. Kokozay, S. Pullen, J. Liu, J. Zhu, S. Styring and A. Thapper, ChemSusChem, 2016, 9, 2957–2966 CrossRef CAS PubMed.
- M. V. Pavliuk, V. G. Makhankova, O. V. Khavryuchenko, V. N. Kokozay, I. V. Omelchenko, O. V. Shishkin and J. Jezierska, Polyhedron, 2014, 81, 597–606 CrossRef CAS.
- V. F. Odyakov, E. G. Zhizhina, Y. A. Rodikova and L. L. Gogin, Eur. J. Inorg. Chem., 2015, 2015, 3618–3631 CrossRef CAS.
- J. Wang, J. Wang, P. Ma and J. Niu, J. Coord. Chem., 2009, 62, 2641–2647 CrossRef CAS.
- A.-M. Nenner, Acta Crystallogr., Sect. C: Cryst. Struct. Commun., 1985, 41, 1703–1707 CrossRef.
- J. Wang, Y. Feng, J. Zhao, P. Ma, X. Zhang and J. Niu, J. Coord. Chem., 2009, 62, 3754–3762 CrossRef CAS.
- L. N. Xiao, Y. Wang, Y. Chen, Y. Peng, G. D. Li, X. B. Cui, S. Y. Shi, T. G. Wang, Z. M. Gao and J. Q. Xu, Inorg. Chem. Commun., 2010, 13, 1217–1220 CrossRef CAS.
- L.-M. Duan, C.-L. Pan, J.-Q. Xu, X.-B. Cui, F.-T. Xie and T.-G. Wang, Eur. J. Inorg. Chem., 2003, 2003, 2578–2581 CrossRef.
- J. Li, L. Xu, N. Jiang, L. Zhao and F. Li, Struct. Chem., 2011, 22, 1339–1345 CrossRef CAS.
- N. Strukan, M. Devcic, B. Kamenar and M. Cindric, Inorg. Chem. Commun., 1999, 2, 558–560 CrossRef.
- J. Tucher, Y. Wu, L. C. Nye, I. Ivanovic-Burmazovic, M. M. Khusniyarov and C. Streb, Dalton Trans., 2012, 41, 9938–9943 RSC.
- Q. Gao, B. Qi, T. Wu and L. Xu, Inorg. Chem. Commun., 2019, 107, 107481 CrossRef CAS.
- Y. Zhao, Q. Gao, R. Tao, F. Li, Z. Sun and L. Xu, Inorg. Chem. Commun., 2018, 89, 94–98 CrossRef CAS.
- L. Krivosudský, A. Roller and A. Rompel, Acta Crystallogr., Sect. C: Struct. Chem., 2019, 75, 872–876 CrossRef PubMed.
- S. S. Wang and G. Y. Yang, Chem. Rev., 2015, 115, 4893–4962 CrossRef CAS PubMed.
- N. I. Gumerova and A. Rompel, Nat. Rev. Chem., 2018, 2, 0112 CrossRef CAS.
- Y. Izumi, Catal. Today, 1997, 33, 371–409 CrossRef CAS.
- K. Sano, H. Uchida and S. Wakabayashi, Catal. Surv. Asia, 1999, 3, 55–60 CrossRef CAS.
- J. N. Armor, Appl. Catal., A, 2001, 222, 407–426 CrossRef CAS.
- M. J. Howard, G. J. Sunley, A. D. Poole, R. J. Watt and B. K. Sharma, in Science and Technology in Catalysis 1998, ed. H. Hattori and K. Otsuka, Elsevier, 1999, vol. 121, pp. 61–68 Search PubMed.
- J. J. Stracke and R. G. Finke, ACS Catal., 2014, 4, 79–89 CrossRef CAS.
- J. Wei, Y. Feng, P. Zhou, Y. Liu, J. Xu, R. Xiang, Y. Ding, C. Zhao, L. Fan and C. Hu, ChemSusChem, 2015, 8, 2630–2634 CrossRef CAS PubMed.
- H. Lv, J. Song, Y. V Geletii, J. W. Vickers, J. M. Sumliner, G. Musaev, P. Kögerler, P. F. Zhuk, J. Bacsa, G. Zhu, C. L. Hill and G. Djamaladdin, J. Am. Chem. Soc., 2014, 136, 9268–9271 CrossRef CAS PubMed.
- X. B. Han, Y. G. Li, Z. M. Zhang, H. Q. Tan, Y. Lu and E. B. Wang, J. Am. Chem. Soc., 2015, 137, 5486–5493 CrossRef CAS PubMed.
- Q. Han and Y. Ding, Dalton Trans., 2018, 47, 8180–8188 RSC.
- H. Buvailo, V. Makhankova, V. Kokozay, I. V. Omelchenko, S. V. Shishkina, J. Jezierska, M. V. Pavliuk and S. I. Shylin, Inorg. Chem. Front., 2019, 6, 1813–1823 RSC.
- H. I. Buvailo, V. G. Makhankova, V. N. Kokozay, I. V. Omelchenko, S. V. Shishkina, P. Zabierowski, D. Matoga and J. Jezierska, Eur. J. Inorg. Chem., 2017, 2017, 3525–3532 CrossRef CAS.
- H. I. Buvailo, V. G. Makhankova, V. N. Kokozay, I. V. Zatovsky, I. V. Omelchenko, S. V. Shishkina, P. Zabierowski, D. Matoga and J. Jezierska, Eur. J. Inorg. Chem., 2016, 2016, 5456–5466 CrossRef CAS.
- CrysAlis PRO, Agilent Technologies, 2011 Search PubMed.
- O. V. Dolomanov, L. J. Bourhis, R. J. Gildea, J. A. K. Howard and H. Puschmann, J. Appl. Crystallogr., 2009, 42, 339–341 CrossRef CAS.
- G. M. Sheldrick, Acta Crystallogr., Sect. A: Found. Crystallogr., 2008, 64, 112–122 CrossRef CAS PubMed.
- G. M. Sheldrick, Acta Crystallogr., Sect. C: Struct. Chem., 2015, 71, 3–8 Search PubMed.
- A. W. Addison, T. N. Rao, J. Reedijk, J. van Rijn and G. C. Verschoor, J. Chem. Soc., Dalton Trans., 1984, 9, 1349–1356 RSC.
- X.-L. Wang, D.-N. Liu, H.-Y. Lin, G.-C. Liu, N. Han, J. Luan and Z.-H. Chang, RSC Adv., 2015, 5, 56687–56696 RSC.
- A. B. Lysenko, G. A. Senchyk, L. V. Lukashuk, K. V. Domasevitch, M. Handke, J. Lincke, H. Krautscheid, E. B. Rusanov, K. W. Krämer, S. Decurtins and S. X. Liu, Inorg. Chem., 2016, 55, 239–250 CrossRef CAS PubMed.
- S. Kumar, R. P. Sharma, P. Venugopalan, M. Witwicki and V. Ferretti, J. Mol. Struct., 2016, 1123, 124–132 CrossRef CAS.
- N. F. Chilton, R. P. Anderson, L. D. Turner, A. Soncini and K. S. Murray, J. Comput. Chem., 2013, 34, 1164–1175 CrossRef CAS PubMed.
- G. A. Bain and J. F. Berry, J. Chem. Educ., 2008, 85, 532 CrossRef CAS.
- J. L. Wikaira, R. J. Butcher, Ü. Kersen and M. M. Turnbull, J. Coord. Chem., 2016, 69, 57–71 CrossRef CAS.
- M. M. Turnbull, C. P. Landee and B. M. Wells, Coord. Chem. Rev., 2005, 249, 2567–2576 CrossRef CAS.
- S. I. Shylin, M. V. Pavliuk, L. D'Amario, F. Mamedov, J. Sá, G. Berggren and I. O. Fritsky, Chem. Commun., 2019, 55, 3335–3338 RSC.
- S. I. Shylin, M. V. Pavliuk, L. D'Amario, I. O. Fritsky and G. Berggren, Faraday Discuss., 2019, 215, 162–174 RSC.
- C. Lu, J. Du, X.-J. Su, M.-T. Zhang, X. Xu, T. J. Meyer and Z. Chen, ACS Catal., 2016, 6, 77–83 CrossRef CAS.
- A. Prevedello, I. Bazzan, N. Dalle Carbonare, A. Giuliani, S. Bhardwaj, C. Africh, C. Cepek, R. Argazzi, M. Bonchio, S. Caramori, M. Robert and A. Sartorel, Chem.–Asian J., 2016, 11, 1281–1287 CrossRef CAS PubMed.
- M. K. Coggins, M.-T. Zhang, Z. Chen, N. Song and T. J. Meyer, Angew. Chem., Int. Ed., 2014, 53, 12226–12230 CrossRef CAS PubMed.
- K. J. Fisher, K. L. Materna, B. Q. Mercado, R. H. Crabtree and G. W. Brudvig, ACS Catal., 2017, 7, 3384–3387 CrossRef CAS.
- B. Rudshteyn, K. J. Fisher, H. M. C. Lant, K. R. Yang, B. Q. Mercado, G. W. Brudvig, R. H. Crabtree and V. S. Batista, ACS Catal., 2018, 8, 7952–7960 CrossRef CAS.
Footnote |
† Electronic supplementary information (ESI) available. CCDC 1958821. For ESI and crystallographic data in CIF or other electronic format see DOI: 10.1039/d1ra05030c |
|
This journal is © The Royal Society of Chemistry 2021 |
Click here to see how this site uses Cookies. View our privacy policy here.