DOI:
10.1039/D1RA04405B
(Paper)
RSC Adv., 2021,
11, 28632-28642
High-efficiency solid-phase microextraction performance of polypyrrole enhanced titania nanoparticles for sensitive determination of polar chlorophenols and triclosan in environmental water samples†
Received
7th June 2021
, Accepted 1st August 2021
First published on 25th August 2021
Abstract
In this work, a polypyrrole (PPy)/TiO2 nanocomposite coating was fabricated by the direct electropolymerization of pyrrole on annealed TiO2 nanoparticles and evaluated as a novel direct immersion solid phase microextraction (DI-SPME) fiber coating for extraction of trace amounts of pollutants in environmental water samples. The functionalized fiber is mechanically and chemically stable, and can be easily prepared in a highly reproducible manner. The effects of the pyrrole monomer concentration, polymerization voltage and polymerization time on the fiber were discussed. Surface morphological and compositional analyses revealed that the composite coating of nano polypyrrole and titanium dioxide nanoparticles (TiO2NPs) uniformly doped the Ti substrate. The as-fabricated fiber exhibited good extraction capability for phenolic compounds in combination with high performance liquid chromatography-UV detection (HPLC-UV). At the optimum SPME conditions, the calibration curves were linear (R2 ≥ 0.9965). LODs and LOQs of less than 0.026 μg L−1 and 0.09 μg L−1 , respectively, were achieved, and RSDs were in the range 3.5–7.2%. The results obtained in this work suggest that PPy/TiO2 is a promising coating material for future applications of SPME and related sample preparation techniques.
1. Introduction
Phenolic compounds are widely used in chemical industry production processes, such as in disinfectants, wood preservatives, plastics, dyes, pesticides, paper and petrochemical products.1–5 Consequently, phenolic compounds can be detected in environmental water, soil and sediment.6–8 Most phenolic compounds are highly toxic, are not very biodegradable, can cause cancer, abnormalities and mutations, and can accumulate in organisms, with potentially serious impacts on the human endocrine system.9,10 Since the concentration levels of phenolic compounds are generally low in complex matrices, the development of simple, efficient and selective preconcentration and separation techniques for phenolic pollutants in environmental water is highly desirable to achieve reliable results.11
High-performance liquid chromatography (HPLC) is frequently used to determine phenolic compounds, unlike gas chromatography (GC), it does not lead to the derivatization of compounds.12,13 However, with regards to sample preparation and preconcentration steps in phenolic pollutants analysis, solid phase extraction has gradually replaced liquid–liquid extraction, as it avoids the use of a large number of toxic organic solvents. Until now, the literature survey has shown that a variety of SPME fiber coatings such as graphene,14,15 polysulfone,16 carbon nanotubes17–21 and organic polymer have been used in the extraction of phenolic compounds.22–24 This convenient and solvent-free extraction method is also sensitive, inexpensive and easy to operate. In SPME, the selection and fabrication of the sorbent is a key consideration because it can effect the analytical parameters such as selectivity, affinity and capacity. In order to enhance the adsorption capacity, to improve the removal efficiency, and to increase selectivity to the solid phase, the study of the stability and surface modification techniques of the fiber is necessary.25–31
Electrochemical polymerization is a unique method for the formation of coatings, it has the advantages of a simple preparation process, low cost and good reproducibility.32–34 Among the conducting polymers, polypyrrole (PPy) is an intrinsic conductive polymer with a long-range π–π conjugation structure. PPy is a widely researched polymer because of its good electrical conductivity, facile synthesis, good environmental stability in ambient conditions, and fewer toxicological problems over many other conductive polymers. Besides all these advantages, PPy coatings are mechanically resistant with excellent adhesion to metal substrates especially when applying electrochemical methods. The utilization of PPy as a coating material in SPME has been reported previously for the extraction of organic compounds.35–38 TiO2 nanoparticles (TiO2NPs) are well-defined nanomaterials with large specific surface area, high chemical stability, durability, corrosion resistance, and low-toxicity, and they are easily functionalized by incorporation of organic groups. TiO2NPs have been employed as fiber coatings for the preconcentration and determination of a wide range of organic compounds.39 In order to develop nanostructured PPy on robust substrates for practical applications, it would be of great technological importance to be able to deposit PPy directly on the desired nanostructured substrate for a particular application in a simple and cost-effective way. The aim of the present work is to fabricate a novel PPy/TiO2 nanocomposite using a potentiostatic technique used for the first time as fiber coating for SPME in combination with HPLC-UV, and evaluate its application for the extraction and preconcentration of organic substance in water samples. Electrochemical anodic oxidation of titanium wire is a reliable strategy for the direct growth of the nanostructured TiO2 coating because of its simplicity, controllable morphology and strong reproducibility in fiber fabrication. More titanol groups in TiO2NPs increase the number of potential reaction centers. In addition, the porous surface structure makes it easier for pyrrole to dope the TiO2NPs to form a composite nanomaterial.
The selectivity, interaction mechanism and extraction kinetics of the PPy@TiO2NPs coating were investigated. The effects of various parameters influencing the performance of the extraction are fully discussed in order to achieve the best experimental results. The validation and the application of the developed method are also addressed for the sensitive determination of trace levels of phenolic pollutants in various water samples.
2. Experimental
2.1. Chemicals and reagents
Polypyrrole (PPy) was supplied by Alfa Aesar (Ward Hill, MA, USA) and was distilled under reduced pressure and stored in a dark bottle in a refrigerator before use. Sodium dodecyl sulfate, sodium chloride (NaCl), ammonium fluoride (NH4F), and ethylene glycol were purchased from Sinopharm Chemical Reagent Co., Ltd. (Shanghai, China). The HPLC-grade methanol was purchased from Yuwang Chemical Company (Shandong, China). Certified individual standards of 2-chlorophenol (2-CP), 2,4-dichlorophenol (2,4-DCP), 2,6-dichlorophenol (2,6-DCP), 2-(2,4-dichlorophenoxy)-5-chlorophenol (Triclosan, TCS), 2,4,4′-trichlorobiphenyl (PCB-28), 2,4′,5-trichlorobiphenyl (PCB-31), 2,3′,4,4′,5-pentachlorobiphenyl (PCB-118), 2,2′,4,4′,5,5′-hexachlorobiphenyl (PCB-153), were obtained from AccuStandard (New Haven, CT, USA). 2-Hydroxy-4-methoxy-benzophenone (BP-3), 2-ethylhexyl 4-(N,N-dimethylamino)benzoate (OD-PABA), 2-ethylhexyl-4-methoxycinnamate (EHMC), 2-ethylhexyl salicylate (EHS) were supplied by AccuStandard (New Haven, CT, USA). Naphthalene (Nap), phenanthrene (Phe), fluoranthene (Flt), pyrene (Pyr) and benzo[a] pyrene (B[a]p) were purchased from Aldrich (St. Louis, MO, USA). Stock standard solutions (100 mg L−1) of each compound were prepared in methanol. Working standard solutions were freshly prepared by diluting the stock standard solution with ultrapure water to the required concentration to study extraction performance under different conditions. These solutions were stored in amber bottles in the refrigerator at 4 °C, shielding from light. All other reagents were of analytical grade.
Ti wire (Φ 250 μm, 99.9% in purity) was supplied by the Alfa Aesar (Ward Hill, MA, USA). Commercially available polyacrylate (PA, 85 μm thickness) and polydimethylsiloxane (PDMS, 100 μm thickness) were obtained from Supelco (Bellefonte, PA, USA). A 0.45 μm micropore membrane of polyvinylidene fluoride was purchased from Xingya Purifying Material Factory (Shanghai, China).
2.2. Instrumentation
Anodization of Ti wires was performed with a precise WY-3D power supply (Nanjing, China). TiO2 nanoparticles were annealed in an air atmosphere at high temperatures in a OTF-1200X tubular furnace with a Prog/Controller (Kejing, Hefei, China). A CHI832D electrochemical analyzer (Chenhua, China) was used for electropolymerization of PPy. The fabricated fibers were characterized by an Ultra Plus microscope (Zeiss, Oberkochen, Germany) with an Aztec-X-80 energy dispersive X-ray spectrometer. SPME was carried out in a DF-101S thermostat with controlled temperature and magnetic stirring (Changcheng, Zhengzhou, China). The analyses were carried out on a Waters 600E multi-solvent delivery system (Milford, MA, USA) equipped with a Waters 2487 dual λ absorbance detector and a Zorbax Eclipse Plus C 18 column (150 mm × 4.6 mm, 5 μm, Agilent, USA). Chromatographic data was processed with a N2000 workstation (Zhejiang University, China). Desorption was performed in a commercially available SPME-HPLC interface (Supelco, PA, USA). Ultrapure water was obtained from a Sudreli SDLA-B-X water purification system (Chongqing, China).
2.3. Preparation of the PPy@TiO2NPs/Ti fiber
Fabrication of the PPy@TiO2NPs nanocomposite SPME coating on the Ti wire involved the following processes: (1) Ti wire of length 6.0 cm was used to fabricate the SPME fibers. One end (1.5 cm long) of the Ti wire was rinsed with methanol and ultrapure water in an ultrasonic bath for 5 min, respectively. (2) The cleaned Ti wire was immersed into the electrolyte of ethylene glycol and water (v/v, 1
:
1) containing NH4F of 0.5% (w/v) for anodization at 25 V for 60 min at room temperature. TiO2NPs were formed on the Ti wire in situ using Ti wire as the working electrode and a Pt rod as the counter electrode. (3) The TiO2NPs coating was washed with ultrapure water and then annealed in a quartz tubular furnace at a heating rate of 5 °C min−1 up to the preset temperature and held for 2 h. After annealing, the TiO2NPs coating was then allowed to cool under a nitrogen stream. (4) The composite coating of PPy@TiO2NPs was synthesized electrochemically via in situ polymerization on the surface of the annealed TiO2NPs (as the working electrode) from an aqueous solution containing pyrrole and SDS (0.01 M) by applying a constant potential. Thereafter the prepared fiber was washed with acetone and ultrapure water to remove pyrrole monomers in the PPy@TiO2NPs coating, and dried in vacuum for 3 h at 90 °C. It had been reported in the literature that the introduction of a large counter anion such as anionic surfactants during the preparation of the PPy, leads to improved properties of the polymers.40,41
The added SDS will link with TiO2 via sulfonate-like ester bonds, improving the lipophilicity of the surface of TiO2NPs, and making pyrrole easy to polymerize on its surface. The generated PPy strengthens the bond to inorganic materials, making the polymer difficult to remove. However, high levels of SDS would increase the solution viscosity, resulting in slow ion migration rates under a static state and corresponding reaction rate reduction.
2.4 SPME-HPLC procedure
To examine the extraction capabilities of the fiber, aqueous solutions spiked with the organic compounds were extracted with PPy@TiO2NPs/Ti fibers using the DI-SPME mode. Prior to use, the fiber was conditioned in methanol for 15 min with an agitation speed of 600 rpm. A 15 mL working standard solution or sample solution was placed into a 20 mL glass vial with a 1 cm magnetic stirrer bar inside, and a polytetrafluoroethylene-coated septa. The SPME fiber was immersed into the heated and stirred sample solution for extraction. Subsequently, the fiber was removed from the sample vial and transferred immediately into the SPME-HPLC interface for static desorption of analytes in the mobile phase. The mobile phase of methanol and water was 70/30, 90/10, 75/25 and 85/15 (v/v) at a flowrate of 1 mL min−1 for HPLC analysis of phenolic compounds, PAHs, aromatic amines and UV filters, respectively. The corresponding wavelengths of UV detection were set at 282 nm, 254 nm, 282 nm and 310 nm. To avoid a carryover effect, the fabricated fiber was washed with methanol and ultrapure water for 10 min before the next extraction, respectively.
2.5. Water and waste water samples
A river water sample was freshly collected from the Yellow River (Lanzhou, China). Wastewater (untreated) was sampled from a local wastewater treatment plant. Tap water samples were collected from the university. All water samples were filtered through 0.45 μm micropore membranes to eliminate particulate matter, and then adjusted to pH 7.0 with phosphate buffer, and stored in the refrigerator at 4 °C before analysis.
3. Results and discussion
3.1 Optimization and characterization of electropolymerization
Scanning electron microscopy (SEM) was used to investigate the surface morphology and structure of the PPy@TiO2NPs/Ti fiber. As compared with the untreated Ti wire (Fig. 1a), the anodized fiber shows a considerably rough microscopic surface (Fig. 1b) and exhibits the randomly oriented nanoparticles coating after anodization. After the anodized fiber was annealed at 550 °C for 2 h under a nitrogen atmosphere, the resulting coating possesses a larger surface area, more open access sites, thus providing the desired contact surface area for subsequent strong adhesion of the PPy coating to the TiO2NPs (Fig. 1c). As can be seen in Fig. 1d, homogeneous PPy was immobilized onto the TiO2NPs/Ti fiber after electropolymerization of pyrrole and the PPy@TiO2NPs composite coating was formed on the Ti wire.
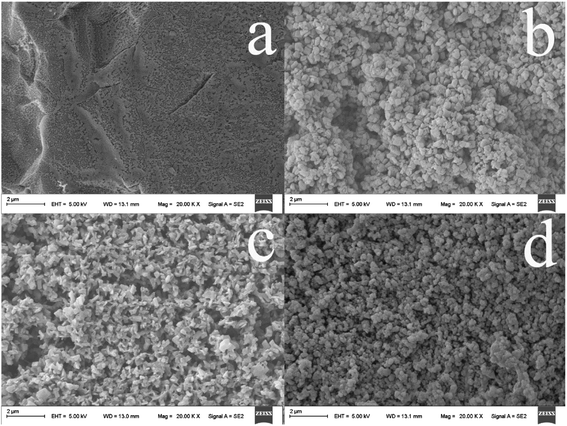 |
| Fig. 1 SEM images of the commercial Ti wire (a), the TiO2NPs/Ti fiber (b), the annealed TiO2NPs/Ti fiber (c), and the PPy@TiO2NPs/Ti coated fiber (d). | |
3.1.1 Pyrrole. The effects of different concentrations of pyrrole on the structure of the fabricated fiber coatings was studied. The amount of PPy electrodeposited on TiO2NPs increases with increasing concentration of pyrrole (Fig. 2a–d). As depicted in Fig. 2b, electrodeposition of PPy is homogeneous at lower concentrations of pyrrole. The results show that along with the increase of the pyrrole concentration, PPy size increases, and aggregation occurs, but it is difficult to form the cross-doping polymer coating. When the concentration of pyrrole reached 0.1 mol L−1, the TiO2NPs were completely covered by PPy, forming a dense deposited film, resulting in a lower specific surface. The surface elements of the fiber were further determined by EDS, and the spectral signal of Ti was hardly observed, which indicates that the TiO2NPs were completely coated by polypyrrole (Fig. 2d). In the case of 0.05 mol L−1 pyrrole, the uniform and compact PPy coating firmly adheres to the TiO2NPs surface.
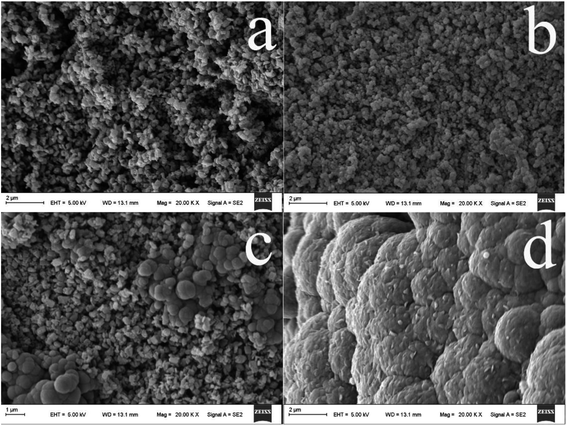 |
| Fig. 2 SEM images of the PPy@TiO2NPs coating at a pyrrole concentration of 0.02 mol L−1 (a), 0.05 mol L−1 (b), 0.08 mol L−1 (c) and 0.1 mol L−1 (d). | |
3.1.2 Applied voltages. Nucleation, oligomerization and growth of PPy are strongly influenced by applied voltages on different metallic substrates. As shown in Fig. 3, different anodic voltages led to PPy coatings with different morphologies. In this study, a relatively lower voltage was needed for the growth of PPy nanoparticles on the annealed TiO2NPs fiber. The SEM image of the nanocomposite showed the growth of the homogenous pattern of PPy, which is present in the pores of the TiO2NPs porous matrix (Fig. 3b). This unique nanostructure results in a much larger surface area, more open access sites and better durability of the as-fabricated fiber. With the increase of voltage (Fig. 3c and d), the pyrrole polymerization reaction was accelerated. High anodic voltages destroy the doped inorganic/organic nanostructures and produce a cauliflower-like PPy coating, resulting in a sharp reduction in the specific surface (Fig. 3d). Therefore 4 V was applied for the electropolymerization of pyrrole on the TiO2NPs coating.
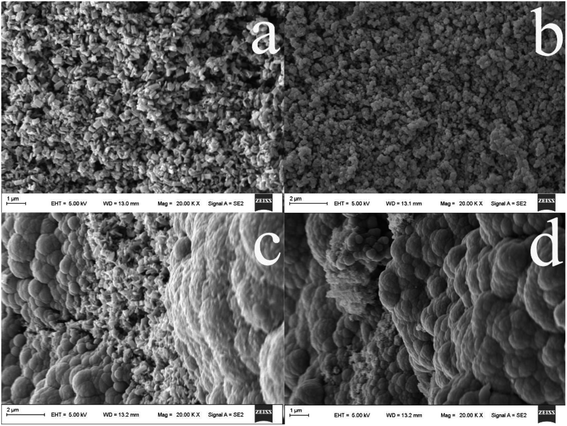 |
| Fig. 3 SEM images of PPy@TiO2NPs at electropolymerization voltages of 2 V (a), 4 V (b), 6 V (c) and 8 V (d). | |
3.1.3 Polymerization time. Furthermore, the effect of polymerization time on different surface morphology of the fiber was studied. It can be seen in Fig. 4, that only a small amount of pyrrole was polymerized to the surface of TiO2NPs coating within 20 minutes. With the extension of polymerization time, PPy particles continue to aggregate and grow. However, when the polymerization time exceeded 40 minutes, the interdoped nanostructures of PPy/TiO2 were gradually replaced by PPy agglomerates (Fig. 4c and d). Therefore, the experiment used 40 minutes as the reaction time to prepare the fiber.
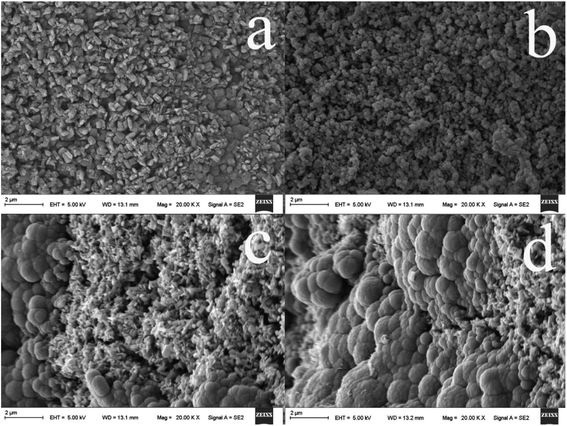 |
| Fig. 4 SEM micrographs of the PPy@TiO2NPs/Ti fiber after polymerization for 20 min (a), 40 min (b), 60 min (c), 80 min (d). | |
3.2 Surface composition
To find out the reasons for the high affinity of the fabricated fiber to the corresponding target analytes, surface elemental analysis of the Ti wire before and after treatment was performed by energy dispersive X-ray spectroscopy (EDS). As shown in Fig. 5a, the presence of a weak O peak in the EDS spectrum of the commercial Ti wire illustrates the formation of a very thin passivation layer at the surface of the commercial Ti wire. Fig. 5b shows peaks corresponding to the presence of Ti and O. The EDS analysis demonstrates the drastic increase in oxygen content due to the formation of the TiO2NPs coating. As can be seen in Fig. 5c, the mass ratio of O and Ti remarkably decreases after annealing treatment at 550 °C. These phenomena clearly indicate that the Ti–OH was destroyed by dehydration under high temperature annealing, resulting in the reduction of oxygen content (Fig. 5c). After electropolymerization of pyrrole, the signals of C and N elements are observed, as well as those of O and Ti elements (Fig. 5d). Moreover, the atomic percentages of O and Ti elements dramatically decreased with the advent of C and N elements. The molar atomic ratios of Ti to O and C to N are almost equal to the stoichiometric ratios of TiO2 and pyrrole, respectively. This result clearly indicates that a uniform and compact PPy@TiO2NPs coating was formed, and complete coverage was achieved on the Ti substrate.
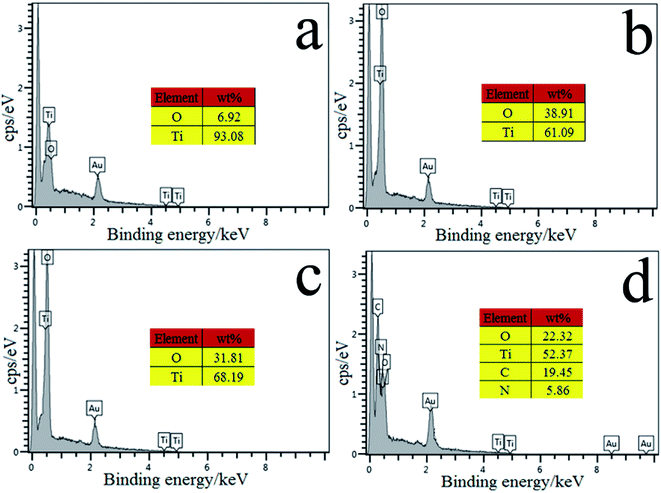 |
| Fig. 5 EDS spectra of the commercial Ti wire (a), the TiO2NPs/Ti fiber (b), the annealed TiO2NPs/Ti fiber (c) and the PPy@TiO2NPs/Ti coated fiber (d). | |
3.3. Extractability of the PPy@TiO2NPs/Ti fiber
The as-prepared SPME fiber coupled with HPLC was then used to extract different polar and structural aromatic compounds including PAHs (Nap, Phe, Flt, Pyr and B[a]p), UV filters (BP-3, OD-PABA, EHMC and EHS), PCBs (PCB-28, PCB-31, PCB-118 and PCB-153) and phenolic compounds (2-CP, 2,4-DCP, 2,6-DCP and TCS). It is exciting that the PPy@TiO2NPs/Ti fiber exhibits excellent extraction capability towards all of the selected organic compounds, especially for phenolic compounds, PAHs and UV filters as shown in Fig. 6.
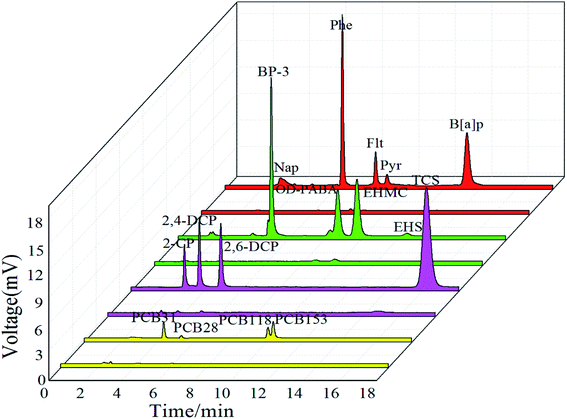 |
| Fig. 6 Typical chromatograms from direct HPLC for PCBs (a), phenolic compounds (c), UV filters (e) and PAHs (g) in water spiked with 25 μg L−1; as well as the corresponding SPME-HPLC with the PPy@TiO2NPs/Ti fiber for PCBs (b), phenolic compounds (d), UV filters (f) and PAHs (h). SPME conditions: extraction time, 55 min; temperature, 40 °C; desorption time, 7 min; stirring, 300 rpm. | |
This special extraction capability may be attributed to the inherent chemical nature of the PPy@TiO2NPs coating. The surface doped fiber coating retained a large amount of the TiO2NPs structure. TiO2 component in the PPy@TiO2NPs composite coating may have Lewis acid sites on the coating surface, which provides the potential retention of non-polar PAHs, less polar UV filters and PCBs through Lewis acid–base interactions. However, due to the hydrophobicity of the TiO2, the TiO2NPs coating allows negligible extraction of hydrophilic phenolic compounds.42 Pyrrole were immobilized on to the surface of the TiO2NPs coating via electrical aggregation and led to its hydrophilicity, allowing the extraction of polar compounds by hydrophilic interaction. Furthermore, additional π–π stacking interactions between PPy and aromatic systems may also contribute to the good extraction capability of PPy@TiO2NPs toward compounds with a larger π–π conjugated structure. The extraction efficiency of phenolic compounds was dramatically improved after surface functionalization. Therefore this novel fiber provides a hopeful approach to effectively extract and analyze trace amounts of chlorophenols and triclosan from complex environmental water samples.
3.4. Optimization of DI-SPME-HPLC-UV conditions
The extraction efficiency of SPME fibers is significantly influenced by extraction conditions. To evaluate the capability of the new fiber, a mixture of four phenolic compounds – 2-CP, 2,4-DCP, 2,6-DCP and TCS – were tested by coupling SPME to HPLC. In order to achieve the optimal extraction efficiency, the effect of various parameters on the efficiency of the SPME process, such as extraction time, desorption time, extraction temperature, extraction temperature and ionic strength of sample solutions, were studied. All measurements were carried out in triplicate at the level of 25 μg L−1 of each phenolic analyte.
3.4.1. Effect of extraction and desorption time. The extraction time is an important parameter in achieving equilibrium in the distribution of analytes between the fiber and the sample. Extraction time also determines the sensitivity and reproducibility of the proposed method. The effect of time on extraction efficiency was examined over 10–60 min with other extraction conditions kept constant. 30 min was enough to nearly reach equilibrium between the fiber and aqueous phase (Fig. S1a†). In DI-SPME-HPLC analysis, desorption time should be optimized in order to ensure the quantitative transfer of the analytes into the HPLC injection port. After extraction, solvent desorption of the extracted phenolic compounds was performed in the mobile phase. For the target phenolic analytes, the peak area reached a constant maximum within 7 min. Consequently, extraction of 30 min and desorption of 7 min were employed in the following experiments.
3.4.2. Effect of extraction temperature. Temperature is very important for SPME because of its potential influence on mass transfer and the partitioning of target analytes between the fiber coating and sample solution.43 Higher temperature can increase the diffusion coefficient of analytes in water and shorten the extraction time. On the other hand, temperature can decrease the partition coefficient between the coating and sample matrix because the absorption is generally an exothermic process. In addition, as the temperature rises, air bubbles tend to form in the aqueous matrix, which has adverse action on the adsorption of the analytes. Fig. S1b† presents the effect of temperature on the extraction of phenolic analytes from 20 to 70 °C. The highest extraction efficiencies were achieved at 40 °C. Subsequently, the extraction capability quickly decreased when the temperature continuously increased. Thus 40 °C was employed for further extraction.
3.4.3. Effect of stirring. Stirring is an important kinetic parameter because extraction is a dynamic diffusion-controlled process. Agitating the solution accelerates the mass transfer of the analytes from bulky solution to fiber coating, and hence facilitates the equilibrium. The effect of sample stirring rates on the extraction efficiency in the range of 300–800 rpm was studied. The results indicated that the extraction efficiency was increased by raising the stirring rate up to 500 rpm (Fig. S1c†). The lower efficiency at higher stirring rates may be due to air bubbles formed on the fiber surface. Therefore, the optimal stirring rate was fixed at 500 rpm.
3.4.4. Effect of ionic strength. It is well known that ionic strength of the solution produces two opposite results for SPME. As shown in Fig. S1d,† a significant positive effect on the extraction efficiency of the phenolic compounds was observed in the aqueous phase with NaCl of 10% (w/v). In this case, the salt out effect played a dominant role. When the content of NaCl is greater than 10%, the extraction effect deteriorates due to the competitive adsorption of Na+ and Cl−. Therefore, according to the results obtained in this work, 10% NaCl was selected for all subsequent experiments.
3.5. Analytical performance of the PPy@TiO2NPs/Ti fiber in the extraction of phenolic compounds
To better understand the extraction efficiency of the phenolic compounds, the PPy@TiO2NPs/Ti fiber along with the fabricated fibers at different stages, were further compared with the most frequently used PA and PDMS fibers for the extraction of phenolic compounds under the same conditions. According to the results in Fig. 6, the untreated Ti wire, the TiO2NPs fiber and the 100 μm PDMS fiber show negligible extraction capability for phenolic analytes except TCS (Fig. S2a–c†). The PPy@TiO2NPs/Ti fiber (Fig. S2e†) presented superior extraction efficiency for all studied phenolic analytes compared to the PA fiber. This result can be attributed to the increased molecular polarity of the PPy@TiO2NPs/Ti fiber surface modified by nano-PPy, which increased the extraction performance for the phenolic analytes due to the increased compatibility. At the same time, the fiber coating has higher porosity, larger specific surface area and the characteristics of TiO2NPs, so the extraction effect of the fiber coating is better than that of commercial polar fiber PA. Such a PPy@TiO2NPs composite coating provides a potential alternative for selective extraction of phenolic analytes from water samples.
3.5.1 Analytical performance. The analytical performance of the developed method with the PPy@TiO2NPs/Ti fiber was investigated for phenolic compounds under the optimized conditions, by extracting a series of standard working solutions. Some useful analytical data including the corresponding correlation coefficients (R2), recoveries, limits of detection (LODs), limits of quantitation (LOQs) and relative standard deviations (RSD) for the studied compounds using this fiber are listed in Table 1.
Table 1 Analytical parameters of the proposed method with the PPy@TiO2NPs/Ti fiber
Analytes |
Linearity (μg L−1) |
R2 |
Recoverya (%) |
RSDa (%) |
LODs (μg L−1) |
LOQs (μg L−1) |
Single fiber (n = 5) |
Fiber-to-fiber (n = 3) |
Calculated at the concentration level of 50 μg L−1. |
2-CP |
0.1–200 |
0.9965 |
94.5 |
5.8 |
7.2 |
0.024 |
0.09 |
2,4-DCP |
0.1–200 |
0.9982 |
109 |
4.3 |
5.1 |
0.021 |
0.07 |
2,6-DCP |
0.1–200 |
0.9978 |
103 |
4.6 |
5.8 |
0.026 |
0.08 |
TCS |
0.05–200 |
0.9993 |
107 |
3.5 |
4.6 |
0.008 |
0.03 |
The linear calibration curves of 2-CP, 2,4-DCP and 2,6-DCP were obtained in the concentration range 0.1–200 μg L−1, with the correlation coefficients (R2) higher than 0.9965 in all instances. The linear range of TCS was 0.05–200 μg L−1, and R2 was 0.9993. The single fiber repeatability for five replicate extractions of phenolic compounds at the 50 μg L−1 spiked level varied from 3.5% to 5.8%. The fiber-to-fiber reproducibility for three parallel PPy@TiO2NPs/Ti fibers fabricated in different batches ranged from 4.6% to 7.2%. The LODs and LOQs ranged from 0.008 to 0.026 μg L−1 and from 0.03 to 0.09 μg L−1 for phenolic compounds based on the signal to noise ratio (S/N) of 3 and 10, respectively.
3.5.2. Analysis of real water samples. To evaluate the reliability of the proposed method, the presented DI-SPME-HPLC-UV method using the PPy@TiO2NPs/Ti nanocomposite coated fiber was applied to the preconcentration and determination of target chlorophenols and triclosan in river water, wastewater and tap water. The analytical results are summarized in Table 2. No phenolic compounds were found in tap water, but they were detected in the Yellow River water (except 2-CP) and wastewater, especially TCS. The reason is that TCs is an effective external antibacterial disinfectant, which is widely used in high efficiency soaps, sanitary lotion, underarm deodorant, air freshener, refrigerator deodorant, etc., which increase the content of it in environmental water accordingly. To evaluate the matrix effect, water samples were spiked with standards of target phenolic compounds at concentrations of 5.0 and 10 μg L−1, respectively. As can be seen in Table 2, relative recoveries were between 89.7% and 112%, and RSDs varied from 3.49% to 7.24%. Fig. S3† shows typical chromatograms of direct HPLC and SPME-HPLC for phenolic compounds in wastewater. The PPy@TiO2NPs/Ti fiber exhibits a much enhanced extraction efficiency for target analytes. These data demonstrate the applicability of the developed method for the analysis of trace phenolic compounds in environmental water samples.
Table 2 Analytical results of phenolic compounds in different environmental water samples
Water samples |
Analyte |
Original (μg L−1) |
Spiked with 5 μg L−1 |
Spiked with 10 μg L−1 |
Detected (μg L−1) |
Recovery (%) |
RSD (%) |
Detected (μg L−1) |
Recovery (%) |
RSD (%) |
ND, not detected or lower than LOD. |
Yellow River |
2-CP |
NDa |
4.73 |
94.6 |
5.15 |
9.88 |
98.8 |
6.73 |
2,4-DCP |
0.142 |
5.08 |
98.8 |
4.53 |
9.72 |
95.8 |
5.84 |
2,6-DCP |
0.113 |
5.32 |
104 |
4.37 |
11.1 |
110 |
5.36 |
TCS |
3.52 |
9.17 |
108 |
3.49 |
14.2 |
105 |
4.91 |
Wastewater |
2-CP |
0.771 |
6.12 |
106 |
5.34 |
11.2 |
104 |
6.62 |
2,4-DCP |
2.26 |
6.51 |
89.7 |
4.68 |
11.3 |
92.3 |
5.39 |
2,6-DCP |
0.762 |
6.45 |
112 |
4.72 |
11.3 |
105 |
5.75 |
TCS |
1.43 |
6.58 |
103 |
4.13 |
12.5 |
109 |
5.03 |
Tap water |
2-CP |
ND |
4.72 |
90 |
6.03 |
9.67 |
96.7 |
7.24 |
2,4-DCP |
ND |
5.44 |
108 |
4.71 |
10.7 |
107 |
5.35 |
2,6-DCP |
ND |
5.23 |
104 |
4.82 |
10.3 |
103 |
5.68 |
TCS |
ND |
5.30 |
106 |
4.07 |
10.7 |
107 |
5.22 |
3.6. Stability of the PPy@TiO2NPs/Ti fiber
The stability of the metal-based fibers greatly depends on their coating procedures, coating structures and surface properties.44 In our study, the PPy@TiO2NPs coating was modified on the surface of TiO2NPs grown in situ on a Ti fiber substrate with characteristics of good mechanical properties, easy acquirability, flexibility and robustness. The fabricated fiber was immersed in 0.01 mol L−1 NaOH and 0.01 mol L−1 H2SO4 for 12 h, respectively. As a matter of fact, negligible morphological changes were observed from its SEM image. In order to examine its reusability, the PPy@TiO2NPs/Ti fiber was soaked in buffer solution and methanol for 15 minutes in sequence to imitate the extraction process in the SPME procedure. The obtained results revealed that the fabricated fiber could be used at least 250 times for extraction and desorption of phenolic compounds. In this case, the acceptable average recovery (85.2–93.5%) and RSDs (4.23–7.34%) were still achieved for five replicate analyses of the target phenolic compounds at the level of 50 μg L−1. However, what matters is that highly concentrated anionic species might reduce the lifetime of the coating due to the changes in the structure or morphology of the polymer during an exchange of counter ions.45
4. Conclusion
In this study, a new PPy@TiO2NPs/Ti fiber was prepared by the electropolymerization of PPy on the surface of annealed TiO2NPs. The proposed fiber coating has many advantages, such as a porous structure, it is inexpensive and easy to prepare, has a long lifespan, strong interactions, reproducible preparation, and strong adhesion to the Ti matrix. Application of the novel nanocomposite coating as an adsorbent material in direct immersion solid phase microextraction of chlorophenols and triclosan was investigated. The results demonstrated that the fiber exhibits excellent extraction efficiency and good selectivity for SPME of phenolic compounds due to its unique surface properties and larger available surface area. The extraction performance of the PPy@TiO2NPs coating for the selected phenolic compounds is superior to that of commercial PA fiber because it had a higher affinity for the studied phenolic compounds.
Conflicts of interest
There are no conflicts to declare.
Acknowledgements
This research work was financially supported by the Science and Technology Projects of Gansu Province (20JR10RA290), the Key Talent Project of Gansu Province (2021) and Innovation Fund for Higher Education of Gansu Province (2021B-282).
References
- S. Chu and R. J. Letcher, Halogenated phenolic compound determination in plasma and serum by solid phase extraction, dansylation derivatization and liquid chromatography-positive electrospray ionization-tandem quadrupole mass spectrometry, J. Chromatogr. A, 2013, 1320, 111–117 CrossRef CAS PubMed.
- B. Nagendran, K. Sundram and S. Samman, Phenolic compounds in plants and agri-industrial by-products: Antioxidant activity, occurrence, and potential uses, Food Chem., 2006, 99, 191–203 CrossRef.
- U. Mustafa, Adsorption of chlorophenolic compounds on activated clinoptilolite, Adsorpt. Sci. Technol., 2019, 37, 664–679 CrossRef.
- A. O. Olaniran and E. O. Igbinosa, Chlorophenols and other related derivatives of environmental concern: Properties, distribution and microbial degradation processes, Chemosphere, 2011, 83, 1297–1306 CrossRef CAS PubMed.
- P. de Morais, T. Stoichev, M. C. P. Basto, M. Teresa and S. D. Vasconcelos, Extraction and preconcentration techniques for chromatographic determination of chlorophenols in environmental and food samples, Talanta, 2012, 89, 1–11 CrossRef CAS PubMed.
- M. Llompart, B. Blanco and R. Cela, Determination of phenols in soils by in situ acetylation headspace solid-phase microextraction, J. Microcolumn Sep., 2015, 12, 25–32 CrossRef.
- D. Bello and C. Trasar-Cepeda, Extraction and quantification of chlorophenolate molecules in soils spiked with 2,4-dichlorophenol and 2,4,5-trichlorophenol, Sci. Total Environ., 2018, 616–617, 179–186 CrossRef CAS PubMed.
- I. A. Ololade, A. O. Adeola, N. A. Oladoja, O. O. Ololade, S. U. Nwaolisa, A. B. Alabi and I. V. Ogungbe, In situ modification of soil organic matter towards adsorption and desorption of phenol and its chlorinated derivatives, J. Environ. Chem. Eng., 2018, 6, 3485–3494 CrossRef CAS.
- S. Xue, C. Wang and Y. Wei, Preparation of magnetic mesoporous carbon from polystyrene-grafted magnetic nanoparticles for rapid extraction of chlorophenols from water samples, RSC Adv., 2017, 7, 11921–11928 RSC.
- W. Ma and K. H. Row, Solid-phase extraction of chlorophenols in seawater using a magnetic ionic liquid molecularly imprinted polymer with incorporated silicon dioxide as a sorbent, J. Chromatogr. A, 2018, 1559, 78–85 CrossRef CAS PubMed.
- P. Kueseng and J. Pawliszyn, Carboxylated multiwalled carbon nanotubes/polydimethylsiloxane, a new coating for 96-blade solid-phase microextraction for determination of phenolic compounds in water, J. Chromatogr. A, 2013, 1317, 199–202 CrossRef CAS PubMed.
- K. M. Kalili and A. D. Villiers, Recent developments in the HPLC separation of phenolic compounds, J. Sep. Sci., 2015, 34, 854–876 CrossRef PubMed.
- S. Insa, E. Besalú, V. Salvadó and E. Anticó, Assessment of the matrix effect on the headspace solid-phase microextraction (HS-SPME) analysis of chlorophenols in wines, J. Sep. Sci., 2015, 30, 722–730 CrossRef PubMed.
- Q. Liu, J. B. Shi, L. X. Zeng, T. Wang, Y. Q. Cai and G. B. Jiang, Evaluation of graphene as an advantageous adsorbent for solid-phase extraction with chlorophenols as model analytes, J. Chromatogr. A, 2011, 1218, 197–204 CrossRef CAS PubMed.
- X. L. Liu, X. Zhou, C. Wang, Q. H. Wu and Z. Wang, magnetic three-dimensional graphene solid-phase extraction of chlorophenols from honey samples, Food Addit. Contam., Part A, 2015, 32, 40–47 CrossRef CAS.
- X. Y. Liu, J. J. Yin, L. Zhu, G. H. Zhao and H. X. Zhang, Evaluation of a magnetic polysulfone microcapsule containing organic modified montmorillonite as a novel solid-phase extraction sorbent with chlorophenols as model compounds, Talanta, 2011, 85, 2451–2457 CrossRef CAS.
- J. Ma, L. Hou, G. Wu, L. Wang and L. Chen, Multi-Walled Carbon Nanotubes for Magnetic Solid-Phase Extraction of Six Heterocyclic Pesticides in Environmental Water Samples Followed by HPLC-DAD Determination, Materials, 2020, 13, 5729–5733 CrossRef CAS PubMed.
- Z. Afsharsaveh, H. Sereshti and H. R. Nodeh, Monitoring of priority pollutants chlorophenols in water and milk by headspace solid phase microextraction based on electrospun polycaprolactam nanofibers decorated with cadmium oxide-carbon nanotubes, J. Sep. Sci., 2020, 43, 4216–4224 CrossRef CAS PubMed.
- X. R. Hu, C. H. Wang, R. Luo, C. Liu, J. W. Qi, X. Y. Sun, J. Y. Shen, W. Q. Han, L. J. Wang and J. S. Li, Double-shelled hollow ZnO/carbon nanocubes as an efficient solid-phase microextraction coating for the extraction of broad-spectrum pollutants, Nanoscale, 2019, 11, 2805–2811 RSC.
- M. Gołębiowski, P. Stepnowski and D. Leszczyńska, Application of carbon nanotubes as solid-phase extraction sorbent for analysis of chlorophenols in water samples, Chem. Pap., 2017, 71, 831–839 CrossRef.
- Y. Sun, W. Y. Zhang, J. X. Cheng and M. Wang, Solid-phase microfibers based on modified single-walled carbon nanotubes for extraction of chlorophenols and organochlorine pesticides, Microchim. Acta, 2011, 173, 223–229 CrossRef CAS.
- X. Y. Liu, J. J. Yin, L. Zhu, G. H. Zhao and H. X. Zhang, Evaluation of a magnetic polysulfone microcapsule containing organic modified montmorillonite as a novel solid-phase extraction sorbent with chlorophenols as model compounds, Talanta, 2011, 85, 2451–2457 CrossRef CAS.
- H. Bagheri, A. Mohammadi and A. Salemi, On-line trace enrichment of phenolic compounds from water using a pyrrole-based polymer as the solid-phase extraction sorbent coupled with high-performance liquid chromatography, Anal. Chim. Acta, 2004, 513, 445–449 CrossRef CAS.
- M. S. Chai, Y. H. Chen, R. R. Xuan, J. F. Ma and Z. F. Jin, Application of polyethyleneimine-modified attapulgite for the solid-phase extraction of chlorophenols at trace levels in environmental water samples, Anal. Bioanal. Chem., 2018, 410, 6643–6651 CrossRef CAS PubMed.
- H. Bagheri, H. Piri-Moghadam and M. Naderi, Towards greater mechanical, thermal and chemical stability in solid-phase microextraction, TrAC, Trends Anal. Chem., 2012, 34, 126–139 CrossRef CAS.
- S. Muhammad, N. M. Khaled, M. Rutkowska, N. Szczepańska, J. Namieśnik and J. Płotka-Wasylka, Solid Phase Microextraction: Apparatus, Sorbent Materials, and Application, Crit. Rev. Anal. Chem., 2019, 49, 271–288 CrossRef.
- R. Esfandiarnejad and H. Sereshti, Designing an absolutely solvent-free binary extraction system as a green strategy for ultra-trace analysis of chlorophenols, Microchem. J., 2019, 146, 701–707 CrossRef CAS.
- X. Yang, T. Muham, J. Yang, A. Yasen and L. Chen, In situ kinetic and thermodynamic study of 2,4-dichlorophenoxyacetic acid adsorption on molecularly imprinted polymer based solid-phase microextraction coatings, Sens. Actuators, A, 2020, 313, 112190 CrossRef CAS.
- G. Li and K. H. Row, Deep eutectic solvents skeleton typed molecularly imprinted chitosan microsphere coated magnetic graphene oxide for solid-phase microextraction of chlorophenols from environmental water, J. Sep. Sci., 2020, 43, 1063–1070 CrossRef CAS PubMed.
- J. P. Ma, R. H. Xiao, J. H. Li, J. Li, B. Z. Shi, Y. J. Liang, W. H. Lu and L. X. Chen, Headspace solid-phase microextraction with on-fiber derivatization for the determination of aldehydes in algae by gas chromatography-mass spectrometry, J. Sep. Sci., 2011, 34, 1477–1483 CrossRef CAS PubMed.
- M. M. Ma, H. J. Wang, Q. Zhen, M. Zhang and X. Z. Du, Development of nitrogen-enriched carbonaceous material coated titania nanotubes array as a fiber coating for solid-phase microextraction of ultraviolet filters in environmental water, Talanta, 2017, 167, 118–125 CrossRef CAS PubMed.
- A. Spietelun, M. Pilarczyk, A. Kloskowski and J. Namieśnik, Current trends in solid-phase microextraction (SPME) fibre coatings, Chem. Soc. Rev., 2010, 39, 4524–4537 RSC.
- B. Buszewski, P. Olszowy, S. Pikus and M. Kozak, Electropolymerized nanoporous polymeric SPME coatings: preparation and characterization by small angle X-ray scattering and scanning electron microscopy, Monatsh. Chem., 2014, 145, 527–531 CrossRef CAS PubMed.
- M. O. Aziz-Zanjani and A. Mehdinia, Electrochemically prepared solid-phase microextraction coatings-A review, Anal. Chim. Acta, 2013, 781, 1–13 CrossRef CAS PubMed.
- A. R. Ghiasvand, S. Abdolhosseini, N. Heidari and B. Paull, Evaluation of polypyrrole/silver/polyethylene glycol nanocompositesorbent
for electroenhanced direct-immersion solid-phase microextraction of carvacrol and thymol from medicinal plants, J. Iran. Chem. Soc., 2018, 15, 2585–2592 CrossRef CAS.
- R. Zakerian and S. Bahar, Electrochemical preparation of zinc oxide/polypyrrole nanocomposite coating for the highly effective solid-phase microextraction of phthalate esters, J. Sep. Sci., 2017, 40, 4439–4445 CrossRef CAS PubMed.
- J. H. Li and H. Xu, A novel polyaniline/polypyrrole/graphene oxide fiber for the determination of volatile organic compounds in headspace gas of lung cell lines, Talanta, 2017, 167, 623–629 CrossRef CAS PubMed.
- F. Ghasemi, S. Pirsa, M. Alizadeh and F. Mohtarami, Extraction and determination of volatile organic acid concentration in pomegranate, sour cherry, and red grape juices by PPy-Ag nanocomposite fiber for authentication, Sep. Sci. Technol., 2018, 53, 117–125 CrossRef CAS.
- Y. Li, M. Zhang, Y. X. Yang, X. M. Wang and X. Z. Du, Electrochemical in situ fabrication of titanium dioxide-nanosheets on a titanium wire as a novel coating for selective solid-phase microextraction, J. Chromatogr. A, 2014, 1358, 60–67 CrossRef CAS PubMed.
- R. Wang, J. D. Zhang and Y. G. Hu, Liquid phase deposition of hemoglobin/SDS/TiO2 hybrid film preserving photoelectrochemical activity, Bioelectrochemistry, 2011, 81, 34–38 CrossRef CAS.
- E. Núñez-Rojas and H. Domínguez, Computational studies on the behavior of Sodium Dodecyl Sulfate (SDS) at TiO2(rutile)/water interfaces, J. Colloid Interface Sci., 2011, 364, 417–427 CrossRef PubMed.
- H. M. Liu, D. A. Wang, L. Ji, J. B. Li, S. J. Liu, X. Liu and S. X. Jiang, A novel TiO2 nanotube array/Ti wire incorporated solid-phase microextraction fiber with high strength, efficiency and selectivity, J. Chromatogr. A, 2010, 1217, 1898–1903 CrossRef CAS PubMed.
- F. Li, L. X. Hou, Y. H. Zhang, Y. K. Wang and G. H. Yan, Synthesis of Highly Hydrophobic Rutile Titania-silica Nanocomposites by an Improved Hydrolysis Co-precipitation Method, Ceram. Int., 2017, 43, 5592–5598 CrossRef.
- J. J. Feng, H. D. Qiu, X. Liu and S. X. Jiang, The development of SPME fibers with metal wires as supporting substrates, TrAC, Trends Anal. Chem., 2013, 46, 44–58 CrossRef CAS.
- J. Wu and J. Pawliszyn, Preparation and applications of polypyrrole films in SPME, J. Chromatogr. A, 2001, 909, 37–52 CrossRef CAS PubMed.
Footnote |
† Electronic supplementary information (ESI) available. See DOI: 10.1039/d1ra04405b |
|
This journal is © The Royal Society of Chemistry 2021 |
Click here to see how this site uses Cookies. View our privacy policy here.