DOI:
10.1039/D1RA03772B
(Paper)
RSC Adv., 2021,
11, 25431-25440
Effects of trypsin-induced limited hydrolysis on the structural, functional, and bioactive properties of sericin†
Received
14th May 2021
, Accepted 10th July 2021
First published on 21st July 2021
Abstract
The effects of trypsin-induced hydrolysis on the structural, functional, and antioxidant properties of sericin were studied. The structural properties of sericin and its hydrolysates were characterized by using SDS-PAGE, SEC-HPLC, surface hydrophobicity, and circular dichroism. Antioxidative properties were evaluated based on quenching capacity against hydroxyl, DPPH, and ABTS, and metal (Fe2+, Cu2+) chelating activity. The enzymatic hydrolysis raised the flexibility, changed emulsifying and foaming properties, and improved the solubility and antioxidant activity of sericin. Meanwhile, the hydrolysis led to a decline in gelation capacity, oil holding capacity, and water holding capacity. Sericin and its hydrolysates exhibited excellent function with regard to oil holding, emulsifying, and foaming. Sericin and its hydrolysates had clear effects on the growth of both Enterococcus faecalis and Lactobacillus bulgaricus strains.
1. Introduction
Silk sericin, known as silk gum, is a globular protein that accounts for 20–30% of the weight of cocoons. In the textile industry, sericin has to be removed from the raw silk through degumming before dyeing and is discarded as industrial waste.1 Approximately 50
000 tons of sericin are discharged with industrial wastewater all around the world every year, which not only threatens the environment because of the great oxygen demand for degradation of sericin but also wastes a valuable resource.2 Meanwhile, according to a report released by the World Health Organization, the number of people who suffered starvation reached 821 million in 2017, indicating a global shortage of protein-rich foods.3 Thus, there is growing interest in respect to the recovery of protein-rich byproducts, which would be useful in action against hunger and malnutrition worldwide. Sericin has a special amino acid composition, among which glycine (10–14%), aspartic acid (14–16%), and serine (32–34%) are the three most abundant.4 So far, sericin has been found to have diverse biological functions, such as promoting intestinal absorption of dietary minerals,5 ACE-I inhibitory activity,6 hypoglycemic effects,7 antibacterial and antioxidant activity,8 and maintaining good conditions of the digestive tract.9 In addition, in traditional Uyghur medicine, sericin has been used for the treatment of coronary atherosclerosis and brain diseases.10 These previous reports suggest that sericin can be a valuable natural ingredient for the food industry. However, poor solubility and low digestibility of sericin may be a vital drawback for its wide application in the food industry.11,12
Using enzymatic hydrolysis to modify proteins is a widespread technology, which is applied to obtain novel food ingredients with remarkably increased protein solubility, altered functionalities,13 and various biological activities.14 Although hydrolysis can improve the solubility and digestibility of proteins, excessive hydrolysis impairs functional properties and also leads to bitter tastes caused by the formation of smaller peptides.15 Therefore, when modifying proteins through enzymatic hydrolysis, a limited degree of hydrolysis (DH) between 2–8% is recommended not only to reduce the adverse effect on the taste but also to obtain the desired functionality and bioactivity.16
To the best of our knowledge, little is known about the influence of limited proteolysis on the structural, functional, and bioactive properties of sericin. Trypsin selectively cleaves peptide bonds at the carboxyl side of the arginine or lysine; since sericin has a comparatively high lysine and arginine content, trypsin can effectively hydrolyze sericin.17 Therefore, we used trypsin to perform the limited hydrolysis and evaluated the effects of limited hydrolysis on the structural, functional, and bioactive properties of sericin.
2. Materials and methods
2.1 Materials
Cocoons were purchased from Xinjiang Ensar Uyghur Medicine Pieces Co., Ltd (Urumqi, China). Trypsin (250 N.F.U mg−1) was purchased from Beijing Solarbio Science & Technology Co., Ltd (Beijing, China) and stored at 4 °C. Bicinchoninic acid (BCA) Protein Assay Kit (23227) was purchased from Thermo Scientific (Rockford, IL, USA). 8-Anilino-1-naphthalenesulfonic acid (ANS) was purchased from Sigma-Aldrich Chemical Reagent Co., Ltd (St. Louis, MO, USA). Commercial gelatin was purchased from Tianjin Hongyan Reagent Factory (Tianjin, China). All other chemicals used were of analytical grade.
2.2 Preparation of sericin and its hydrolysates
The extraction of sericin was performed according to the previous report.18 The 10 g cocoon shells were immersed in 500 mL of dH2O (1
:
50, w/v). The mixture was put into an autoclave and heated at 121 °C for 60 min. After cooling down, the mixture was centrifuged at 10
000×g for 10 min. To prepare sericin hydrolysates the supernatant was incubated at 37 °C for 5 min before adjusting the pH value to 8.0 with 0.5 mol L−1 NaOH solution. The proteolysis reaction was started by adding trypsin (1
:
50, E/S). The pH value was kept at 8.0 by adding 0.1 mol L−1 NaOH solution and stirred constantly (1000 rpm) at 37 °C during the hydrolysis. After the proteolysis reaction, the reaction was terminated by heating the reaction mixture in an 80 °C water bath for 15 min. The hydrolysate powder was obtained by lyophilizing, using the FDU-2100 freeze dryer (Eyela, Tokyo, Japan), and kept at −20 °C until use.
The sericin powder was obtained under the same conditions without enzymatic hydrolysis. The protein content of sericin powder was determined by using Elementar Vario MICRO cube, and the protein content was 95.15% (N × 6.25).
2.3 Sodium dodecyl sulfate-polyacrylamide gel electrophoresis (SDS-PAGE)
SDS-PAGE under reducing conditions was conducted using 12% separating gel and 5% stacking gel at 80 V. After the electrophoresis, the gel was silver-stained.19
2.4 Molecular weight distribution by size-exclusion high-performance liquid chromatography (SEC-HPLC)
The molecular weight distribution of sericin and its hydrolysates was determined using high-performance liquid chromatography (LC-20A, Shimadzu, Japan) which is equipped with a column (TSKgel G3000PWXL, 7.8 mm I.D. × 30 cm, 7 μm). 20 mM phosphate-buffered saline (pH 6.3) was selected as an eluent, the flow was 0.5 mL min−1. The sample elution was monitored at 214 nm.
2.5 Intrinsic fluorescence
Measuring the intrinsic fluorescence spectra of sericin and its hydrolysates was carried out using a fluorescence spectrofluorometer (F-7000, Hitachi, Tokyo, Japan) according to the method of Zang.20 0.1% (w/v) solutions of samples were diluted 20-fold in phosphate buffer (10 mmol L−1, pH 7.0). The excitation wavelength was set at 280 nm, the emission was recorded between 290 nm and 450 nm, and the slit width was set at 5 nm for both excitation and emission.
2.6 Surface hydrophobicity (H0) measurements
20 mg of sample was dissolved in 20 mL phosphate buffer (10 mmol L−1, pH 7.0). After stirred at 500 rpm for 30 minutes, the sample solutions were centrifuged at 20
000×g for 20 minutes. Then the supernatants were diluted in phosphate buffer (10 mmol L−1, pH 7.0) to prepare a series dilutions (0.0125, 0.025, 0.05, 0.075, 0.1%, w/v, the final concentrations were determined via BCA protein assay kit). A 20 μL aliquot of 8 mmol L−1 ANS solution was added to the 4 mL sample solution. The mixture was mixed thoroughly and then a 200 μL aliquot of mixture was transferred into a microplate. The fluorescence intensities (FIs) of the samples were measured with a SpectraMax M5e Multi-Mode Microplate Reader (Molecular Devices, San Jose, USA). The excitation wavelength was set at 390 nm and the emission was recorded at 484 nm. The relative fluorescence intensity (RFI) of each sample was obtained by subtracting the FI of the blank. RFI versus sample concentration (mg mL−1) was plotted, and the slope was reported as the surface hydrophobicity index (H0).
2.7 Determination of free amino groups and free thiol groups
The determination of free amino groups of sericin and its hydrolysates was carried out using the OPA (o-phthalaldehyde) method. OPA reagent was prepared according to Nielsen.21 100 μL sample solution was mixed with 2 mL OPA reagent and after 2 min of incubation, the absorbance was read at 340 nm. L-Serine was used to establish a standard curve.
The free thiol group was determined according to the method described by Pham.22 Briefly, a sample solution was prepared by dissolving the sample in Tris–glycine buffer with 8 M urea (pH 8, 0.09 M glycine, 0.086 M Tris). 4 mg mL−1 Ellman's reagent was prepared by dissolving 5,5′-dithio-bis-(2-nitrobenzodic acid) (DTNB) in Tris–glycine buffer with 4 mM EDTA (pH 8, 0.09 M glycine, 0.086 M Tris). 5 mL sample solution was mixed with 50 μL Ellman's reagent and after incubated at room temperature for 45 min, the mixture was centrifuged at 7000×g for 15 min. The supernatant was transferred to a cuvette and absorbance was read at 412 nm. The free thiol group content was expressed as nmol per milligram of the sample, which was computed using the equation below:
Free thiol group content (nmol mg−1) = 73.53 × A412/C |
where
C is the concentration of the sample (mg mL
−1),
A412 is the absorbance measured at 412 nm.
2.8 Circular dichroism (CD) analysis
To obtain the far-UV CD spectra, a Chirascan CD spectrometer (Applied Photophysics, Leatherhead, United Kingdom) was applied according to the protocol described previously23 using a 1.0 mm path length quartz cuvette. 0.1% (w/v) solutions of samples were prepared in phosphate buffer (10 mmol L−1, pH 7.0) and filtered through 0.45-micron filters. The CD spectra of the samples were monitored from 190 to 250 nm and the scans were run three times. The spectra of the samples were averaged and smoothed using the Pro-Data Viewer. The interpretation of the CD spectroscopic data was performed by applying the CDSSTR method in DICHROWEB.24
2.9 Protein solubility
0.1% (w/v) dispersions of sericin and its hydrolysates were prepared in dH2O and stirred at 500 rpm for 10 minutes, and then the pH-values were adjusted to pH 2, 3, 4, 5, 6, 7, 8, 9, and 10 respectively using 0.1 mol L−1, 1 mol L−1 HCl solution or NaOH solution. Then the pH-adjusted solutions were stirred at 500 rpm for 30 minutes. An aliquot of 1.5 mL was taken from each sample and centrifuged at 20
000×g for 20 min before measuring the protein content in the supernatant using BCA assay. Bovine serum albumin (BSA) was used to establish the standard curve. Solubility was computed as the formula below:
2.10 Gelation properties
The gelation properties of sericin and its hydrolysates were determined by the method of Jin.25 A range of 20–100 mg mL−1 sample dispersions with 10 mg mL−1 increments were prepared in test tubes. After kept the samples in a hot water bath (95 °C) for 60 minutes, cooled them down rapidly in cold water. Then all samples in test tubes were kept at 4 °C for 12 h. The determination of the least gelation concentration of each sample was done by observing whether the mixture in the inverted test tube would slip or not.
2.11 Oil holding and water holding capacities
Oil holding capacity (OHC) and water holding capacity (WHC) of sericin and its hydrolysates were confirmed according to the method of Yin.26 Rapeseed oil or water and the sample were mixed thoroughly with a vortex mixer in a 50 mL centrifuge tube. Prior to centrifuging at 6000×g for 20 min, the dispersion stood at ambient temperature for 60 min. The volume of sample retaining rapeseed oil or water was computed by subtracting the volume of the supernatant (mL) from the amount of rapeseed oil or water (mL) added to each sample, and the results were presented as mL of oil or water absorbed per gram of protein sample.
2.12 Emulsifying properties
Measuring the emulsifying activity index (EAI) was conducted applying the method described by Moreno.27 30 mg of each sample was mixed with 30 mL of dH2O in a 50 mL flask. Before the solutions were mixed with 10 mL of rapeseed oil, the pH was adjusted to 5, 7, and 9 respectively. The mixture was homogenized at a speed of 10
000 rpm for 1 min. A 100 μL aliquot of the emulsion was shifted immediately from the bottom of the flask after homogenization and added to 4 mL of 0.1% (w/v) SDS solution. Measuring the absorbance of the diluted emulsion was performed at 500 nm using an ultraviolet spectrophotometer (D-8PC, Runqee, Shanghai, China). EAI was computed using the formula mentioned below:
where A is the absorbance of diluted emulsion, DF is the dilution factor, C is the amount of protein in the per unit of dispersion (mg mL−1), Φ is the optical path length of the cuvette (1 cm), θ is the fraction of rapeseed oil used to form the emulsions.
2.13 Foaming capacity and stability
Determining the foaming properties of sericin and its hydrolysates was carried out referring to the method of Gani.28 0.5% (w/v) 30 mL sample solution was blended at a speed of 10
000 rpm for 60 s with a homogenizer (Wiggens, Germany). The prepared sample was instantly shifted into a 250 mL graduated cylinder to measure total volume. After 10 min and 30 min, the total volume was recorded again. The foaming capacity was computed using the formula below:
where V0 is the volume before homogenization, V1 is the volume after homogenization, V2 is the volume after standing.
2.14 Effect of sericin and its hydrolysates on the growth of probiotics
The effect of sericin and its hydrolysate on the growth of three probiotics in vitro was determined using Fructooligosaccharide (FOS) as a positive control and basal medium without sample as blank control. In brief, Enterococcus faecalis, Bifidobacterium adolescentis, and Lactobacillus bulgaricus were respectively inoculated into MARS, TPY, and KEA medium, and sample solutions were added to the medium. After culturing at 37 °C for 16–18 h, a cell counter was used to enumerate the bacteria. Each sample was performed in triplicate.
2.15 Determination of in vitro antioxidant activities
Determination of hydroxyl radical, 2,2-diphenyl-1-picrylhydrazyl (DPPH) radical, and 2,2′-azino-bis(3-ethylbenzothiazoline-6-sulfonic acid) diammonium salt (ABTS) radical scavenging activity of sericin and its hydrolysates was performed according to the method of Zheng.29 Detailed experimental information was given in ESI.†
2.16 Metal (Fe2+, Cu2+) chelating activity
Determining the ferrous chelating activity of sericin and its hydrolysates was applied to the method of Jin.30 Briefly, 1 mL sample and 0.05 mL FeSO4 (2 mM) were premixed before diluted with 2 mL dH2O. Then 0.1 mL ferrozine solution was added and incubated 10 min before the absorbance was measured at 562 nm.
Determination of the copper chelating activity of sericin and its hydrolysates was performed according to literature with slight modifications.31,32 Briefly, 200 μL PV–Cu2+ complex solution (in 50 mM sodium acetate buffer, pH 6.0, containing 0.04 mM pyrocatechol violet and 0.08 mM CuSO4) was mixed with 50 μL sample solution. After 10 min of incubation, the absorbance at 623 nm was measured with a SpectraMax M5e Multi-Mode Microplate Reader (Molecular Devices, San Jose, USA). The metal chelating activity was computed by applying the following formula:
Results were expressed as mg EDTA equivalents per g sample.
2.17 Statistical analysis
The experiments were conducted at least in triplicate, and the results were presented as mean ± SD. The data were analyzed by the one-way ANOVA test using SPSS 22.0 (SPSS Inc., Chicago, IL). For a p-value <0.05 the differences were considered statistically significant.
3. Results and discussion
3.1 Degree of hydrolysis (DH)
The DH change of trypsin-induced hydrolysis of sericin was conducted using the pH-stat method up to 90 min. As shown in ESI Fig. 1,† the DH profile of sericin with time is consistent with the classic proteolysis of proteins.33 Sericin hydrolysis occurred rapidly within the first 10 min and grew at a relatively slower rate over the next 80 min reaction period. Hydrolysates DH 2.58%, 4.45%, and 6.51% were prepared to evaluate the changes of trypsin-induced hydrolysis on the structural and functional properties of sericin.
3.2 Analysis of SDS-PAGE
Fig. 1 illustrates the SDS-PAGE profile of sericin and its hydrolysates. Continuous high-density protein bands were observed above 150 kDa, between 150 and 10 kDa, and under 10 kDa (lane 2), which is consistent with the previous report that sericin is a family of protein whose molecular weight distribution ranges between 10–300 kDa.34 There are large aggregates on the top of the separating gel (lane 2). The aggregates band and peptide band under 10 kDa on lane 2 suggests that smaller peptides formed during the high-temperature extraction (121 °C). After the hydrolysis, the aggregates band emerged on the top of separating gel intensified (lane 3, 4, 5) which indicates more peptides formed during the hydrolysis. Overall, hydrolysis significantly changed the molecular weight distribution of sericin, hydrolysates DH 2.58%, 4.45%, and 6.51% presented a similar pattern that high-intensity bands between 27–150 kDa almost disappeared, aggregation bands at the top of separating gel and small peptide bands under 10 kDa became more intense.
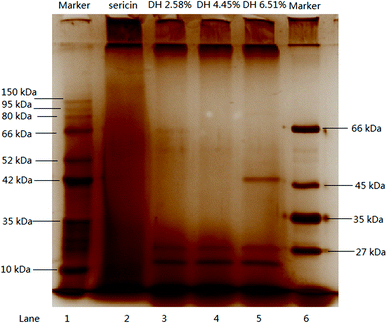 |
| Fig. 1 SDS-PAGE profile of sericin and its hydrolysate. | |
3.3 Molecular weight distribution by SEC-HPLC
SEC-HPLC was used to illustrate the molecular weight distribution of sericin and its hydrolysates (Fig. 2). Three major peaks (I, II, III) were observed on the chromatogram of sericin. After the enzymatic hydrolysis, the main peak I disappeared, whereas, four new peaks (1, 2, 4, and 6) emerged, peak 3 and peak 5 corresponded to the original peak II and III. Aggregates produced by aggregation of low molecular weight peptides are greater than sericin molecules and discernible by SEC-HPLC (peak 1). Trypsin-induced hydrolysis results in the formation of smaller molecular weight hydrolysates and aggregates, thus the overall molecular weight distribution of sericin changed.
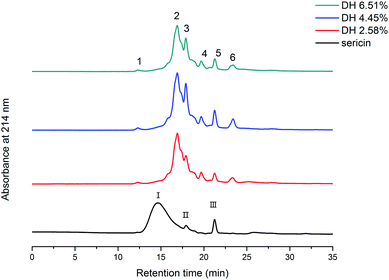 |
| Fig. 2 Molecular weight distribution profile of sericin and its hydrolysates determined by SEC-HPLC. | |
3.4 Intrinsic fluorescence
Fluorescence intensity can indicate the degree of exposure of aromatic amino acids (phenylalanine, tyrosine, and tryptophan) to water, which is linked with the alternation of protein tertiary structure.35 The fluorescence intensities of the sericin hydrolysates were found to be higher than that of sericin (Fig. 3). It can be assumed that trypsin-induced hydrolysis brought about more aromatic groups exposing to water. The fluorescence intensities climbed with increasing DH when the DH was less than 4.45%, however, as the DH further increased to 6.51%, the fluorescence intensity decreased. It suggests that the hydrophobic groups embedded inside of the molecules were exposed to water at the very beginning of the hydrolysis, however, as the DH reached to some extent, more peptide bonds were broken, resulting in more intense hydrophobic interactions among the peptides. These intense interactions impelled the previously exposed hydrophobic groups to bed into the inner part of the molecules again.20,33
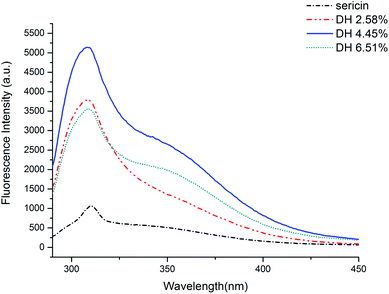 |
| Fig. 3 Intrinsic fluorescence of sericin and its hydrolysates in 10 mm phosphate buffer (pH 7) at 0.05 mg mL−1. | |
3.5 Surface hydrophobicity (H0)
Changes in surface hydrophobicity are often associated with transformation in protein conformations, thus surface hydrophobicity is of importance to studying conformational and functional properties of proteins.36 The surface hydrophobicity of sericin and its hydrolysates with selected DH was quantified (Table 1). In the beginning, the surface hydrophobicity of the hydrolysates showed a trend of rising with increasing hydrolysis when the DH value was lower than 4.45%, but it dropped when the DH reached 6.51%. The rise of surface hydrophobicity indicates that hydrolysis of protein occurred with the unfolding of the protein conformation which caused exposure of hydrophobic parts embedded in the inside of the conformation.35 According to the report of Avramenko,33 the decline in H0 values could be explained as further enzymatic hydrolysis increased hydrophobic interactions that lead to protein aggregation which could rebury hydrophobic groups.
Table 1 Content of free amino, thiol group and surface hydrophobicity of sericin and its hydrolysatesa
Samples |
Free amino groups (nmol per mg per protein) |
Free thiol group (nmol SH per mg per protein) |
Surface hydrophobicity (H0) |
Results are the means of triplicates and different letters in the same column indicate significant differences (p <0.05). |
Sericin |
239.36 ± 1.61a |
1.31 ± 0.16a |
75.76 ± 3.54a |
DH 2.58% |
691.4 ± 9.67b |
1.93 ± 0.10b |
105.34 ± 6.19b |
DH 4.45% |
749.44 ± 11.02c |
4.46 ± 0.26c |
139.68 ± 2.98c |
DH 6.51% |
770.55 ± 14.89c |
2.51 ± 0.09d |
113.50 ± 2.15b |
3.6 The influence of hydrolysis on the content free amino groups and free thiol group
The change in the free amino groups of sericin and its hydrolysates was determined using OPA reagent, which reacts with primary amino groups to form a compound that has absorption at 340 nm.21 The free amino group content (expressed as nmol per mg per protein) of sericin increased during the hydrolysis (Table 1). All hydrolysates have significantly higher free amino group content than sericin (p <0.05). As an indicator of proteolysis efficiency, the changing trend in the content of free amino groups is consistent with the degree of hydrolysis.
The change of free thiol groups' content reflects the alteration in the formation and cleavage of intra- and intermolecular disulfide bond, which is a covalent bond formed between thiol groups.37 Having specificity for thiol groups, DNTB is a useful reagent for the determination of free –SH groups. Besides, the addition of urea breaks hydrogen bonds, so that the change of free thiol group content can be ascribed to covalent bonds. As shown in Table 1, free thiol group content in sericin before enzymatic hydrolysis was 1.31 ± 0.16 nmol SH per mg per protein. When DH reached 2.58% and 4.45%, the free thiol group content increased to 1.93 ± 0.10 and 4.46 ± 0.26 nmol SH per mg per protein, respectively, reflecting the cleavage of disulfide bonds. However, a reduction of the free thiol group was observed at DH 6.51%. According to SDS-PAGE and SEC-HPLC analysis aggregates formed during the hydrolysis of sericin, since aggregation involves disulfide bonds and noncovalent interactions,38 the reduction of the free thiol group might be ascribed to the formation of disulfide bonds during aggregation.
3.7 CD spectra analysis
CD is a convenient means for rapidly quantifying the secondary structure of proteins. The alternation in the secondary structure of sericin that occurred during the hydrolysis was characterized by CD spectroscopy (Fig. 4). Sericin was mainly comprised of random coil (69.0%), β-sheet (17.0%), and β-turn (11.0%), which is in accordance with the previous reports.39,40 The hydrolysis led to changes in the secondary structure of sericin. Compared with those in native sericin, when the DH reached 6.51% the content of random coil rose from 69.0% to 77.0%, and the contents of the β-sheet and β-turn decreased from 17.0% to 12.0%, from 11.0% to 8% respectively. Contrast to more stable β-sheet structures, α-helix, β-turn, and random coils are more flexible. These changes indicate that hydrolysis caused the secondary structure of sericin to become more flexible. Increasing flexibility of protein structure means protein molecules can be more easily absorbed at the interface formed between oil and water boundary, improving the emulsion.41
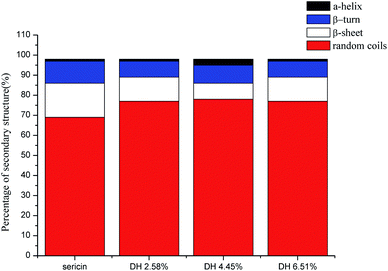 |
| Fig. 4 Estimated secondary structure content of sericin and its hydrolysates determined by CD analysis. | |
3.8 Protein solubility
As a physicochemical property that has to do with other functional properties, protein solubility usually is the basic property measured before developing and testing new protein ingredients.42 The protein solubility profile of sericin and its hydrolysates with different DH is presented in Fig. 5. In contrast to sericin that showed an obvious isoelectric point at pH 4.0, all hydrolysates presented a relatively flat curve over the whole pH range tested. Moreover, trypsin-induced hydrolysis improved the solubility of sericin hydrolysates. The increased solubility of sericin hydrolysates can be ascribed to the generation of smaller peptides and exposure of ionizable amino acids, both of which enhance protein and water molecules' interaction.43
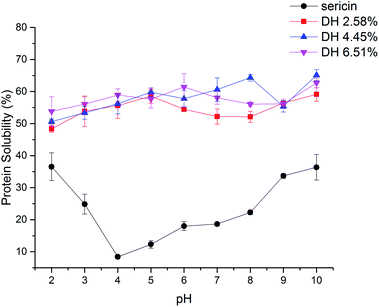 |
| Fig. 5 Protein solubility of sericin and its hydrolysates. Results are the means of triplicates. | |
3.9 Gelation properties
Gelation is an important functionality of proteins and gelation capacity can be compared by the least gelation concentration. As shown in Table 2, the minimum concentration of sericin to form gel is 30 mg mL−1. Though all the sericin hydrolysates showed gelation properties, the minimum gelation concentration rose to 80 mg mL−1 after the hydrolysis. The gelation capacity of protein in an aqueous solution is affected by its molecular mass, amino acid composition, hydrophobicity, and concentration.42 Generation of a three-dimensional (3D) molecular matrix of sericin via hydrogen bonding interactions between sericin–sericin and sericin–water molecules gives rise to the gelation of sericin in an aqueous solution.44 The decrease in the molecular weight of sericin after the hydrolysis indicates a reduction of the average molecular chain length of sericin, which impairs hydrogen bonding interaction among the sericin molecules. In view of the fact that a shorter sericin chain may not come across a proximate sericin chain and form a 3D molecular matrix easily than a longer sericin chain. Hence, the ability of sericin to form a 3D network declines. Besides, it has been found that the gelling process of sericin is accompanied by the β-sheet crystallization of sericin.45 According to the CD spectra analysis (Fig. 4), the hydrolysis led to a decrease in the β-sheet proportion in sericin hydrolysates. Consequently, trypsin-induced hydrolysis impaired the gelation properties of sericin.
Table 2 Gelation properties of sericin and its hydrolysates with different degree of hydrolysisa
Samples |
Concentration (mg mL−1) |
20 |
30 |
40 |
50 |
60 |
70 |
80 |
90 |
100 |
× means the sample slipped when the test tube was inverted. √ means the sample did not drop when the test tube was inverted. |
Sericin |
× |
√ |
√ |
√ |
√ |
√ |
√ |
√ |
√ |
DH 2.58% |
× |
× |
× |
× |
× |
× |
√ |
√ |
√ |
DH 4.45% |
× |
× |
× |
× |
× |
× |
√ |
√ |
√ |
DH 6.51% |
× |
× |
× |
× |
× |
× |
√ |
√ |
√ |
3.10 Water holding capacity (WHC) and oil holding capacity (OHC)
Sericin molecule has highly hydrophilic properties,46 which is of significant importance to protein–water interaction. Sericin and its hydrolysates at different DHs manifested exceptionally good WHC and OHC as presented in Fig. 6. Sericin has significantly higher WHC than its hydrolysates. The OHC was slightly (p >0.05) decreased by hydrolysis. Sericin and its hydrolysates showed superior OHCs compared to commonly used proteins such as milk protein powder (2.8 g of oil per g of protein) and soy protein powder (1.2 g of oil per g of protein).47 An inverse correlation was found between the solubility and WHC and OHC of sericin and its hydrolysates, which is in line with the previous report of Diniz.48
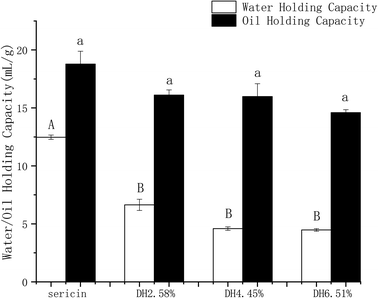 |
| Fig. 6 Water holding capacity and oil holding capacity of sericin and its hydrolysates. Results are the means of triplicates and different letters in the same parameters indicate significant differences (p <0.05). | |
3.11 Emulsifying and foaming properties
In foods such as ice cream, beverages, and dressings, emulsifying and foaming properties play a crucial role. A protein emulsifier takes part in the formation of emulsion and improves the stability of a newly created emulsion.49 EAI is a measure of the amount of oil emulsified by per unit of protein. As presented in Table 3, all samples' EAI profiles with pH change were similar. Sericin and its hydrolysates presented the maximal values at pH 9.0 and minimal EAI values at pH 5.0. Among them, at pH 9.0, the hydrolysates with DH 4.45% showed the highest EAI (44.22 ± 1.21 m2 g−1) as compared to other samples. Besides, sericin had significantly higher EAI than its hydrolysates at pH 5.0 and pH 7.0 (p <0.05).
Table 3 Emulsifying activity index (EAI) of sericin and its hydrolysatesa
Samples |
EAI (m2 g−1) |
pH 5.0 |
pH 7.0 |
pH 9.0 |
Results are the means of triplicates and different letters in the same column indicate significant differences (p <0.05). |
Sericin |
23.16 ± 0.24a |
25.33 ± 1.15a |
32.28 ± 0.39b |
DH 2.58% |
9.75 ± 0.68bc |
17.22 ± 0.33b |
33.06 ± 0.68b |
DH 4.45% |
7.52 ± 0.34c |
15.08 ± 0.30b |
44.22 ± 1.21a |
DH 6.51% |
8.45 ± 0.63c |
14.79 ± 0.47b |
34.54 ± 0.15b |
Gelatin |
11.55 ± 1.06b |
14.22 ± 2.11b |
16.09 ± 1.96c |
The emulsifying capacity of a protein is related to proteins' solubility, flexibility, amphiphilic and interfacial properties.50 For our samples, the changing trend in EAI at pH 9.0 is likely correlated to the observed changing trend in the surface hydrophobicity, in which a rise in protein surface hydrophobicity corresponds to an increased EAI. On the other hand, aggregates increase the oil droplets' coalescence by inhibiting the process of forming viscoelastic films on the oil-in-water surface.26 Thus, the relatively lower EAI of hydrolysates at pH 5.0 and pH 7.0 might be attributed to oil droplets' coalescence caused by the formation of the aggregates in the hydrolysates.
3.12 Foaming properties
Fig. 7 shows the foaming capacity and stability of sericin and its hydrolysates, the foaming properties of gelatin are shown as a comparison. The foaming capacity profile of sericin hydrolysates, which initially showed a trend of increase with increasing hydrolysis when DH was less than 4.45%, but then dropped at DH 6.51%, agrees with the intrinsic hydrophobicity profile of sericin hydrolysates (Fig. 3). According to the foam formation mechanism, the rise in foaming capacity may be attributed to the increase of total hydrophobicity which facilitates the association of the polypeptides to form cohesive films, promoting more air to be incorporated. The decrease in foaming capacity of DH 6.51% is a result of both a drop of total hydrophobicity and forming shorter polypeptide chains that might not favorable for the association of the polypeptides.48
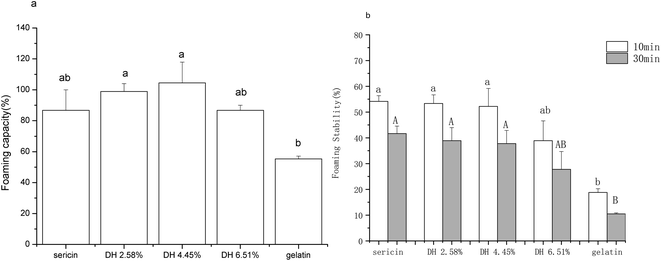 |
| Fig. 7 Foaming properties of sericin and its hydrolysates. (a) Foaming capacity. (b) Foaming stability. Results are the means of triplicates and different letters in the same parameters indicate significant differences (p <0.05). | |
Though the foam stability of sericin and hydrolysates with DH 2.58% and DH 4.45% did not show a significant difference (p >0.05), a drop was observed as DH escalated from 4.45% to 6.51%. It is in line with the previous report that long protein chains can produce thick absorbed films more easily, which is apt for higher foam stability.51
3.13 Effects on growth of probiotics
It has been reported that some proteins and their hydrolysates can selectively promote the growth of probiotics.52 Thus the effect of sericin and its hydrolysates on the growth of Enterococcus faecalis, Bifidobacterium adolescentis, and Lactobacillus bulgaricus was investigated (Table 4). Sericin and its hydrolysates had clear effects on the growth of both Enterococcus faecalis and Lactobacillus bulgaricus strains. The final bacteria counts of Enterococcus faecalis treated with sericin and its hydrolysates were significantly higher (p <0.05) compared to the control and FOS. For the growth of Bifidobacterium adolescentis, the effect of sericin and its hydrolysates is not significant (p >0.05). For Lactobacillus bulgaricus, significantly higher cell growth was observed when the bacteria grew in DH 4.45%. The mechanisms of protein action on probiotics growth are complex, recent studies show that the mechanisms of protein enhancing probiotics including providing nitrogen sources for cell growth, enhancing acid tolerance of bacterial strains, and promoting polypeptide transport systems.53
Table 4 Effect of sericin and its hydrolysates on the growth of E. faecalis, B. adolescentis, and L. bulgaricusa
sample |
Bacterial count (log CFU mL−1) |
E. faecalis |
B. adolescentis |
L. bulgaricus |
Results are the means of triplicates and different letters in the same column indicate significant differences (p <0.05). |
Control |
5.37 ± 0.16a |
3.97 ± 0.17a |
6.77 ± 0.05a |
FOS |
5.95 ± 0.15ab |
4.19 ± 0.10a |
6.65 ± 0.11a |
Sericin |
6.27 ± 0.23b |
3.87 ± 0.17a |
6.94 ± 0.07ab |
DH 2.58% |
6.19 ± 0.12b |
4.03 ± 0.24a |
6.82 ± 0.09ab |
DH 4.45% |
6.12 ± 0.10b |
4.09 ± 0.28a |
7.06 ± 0.09b |
DH 6.51% |
6.21 ± 0.03b |
3.97 ± 0.17a |
7.04 ± 0.13ab |
3.14 Antioxidant activity
Antioxidant activity of sericin and its hydrolysates were evaluated by basing on their scavenging activity against hydroxyl, DPPH, and ABTS, and metal (Fe2+, Cu2+) chelating activity (Fig. 8).
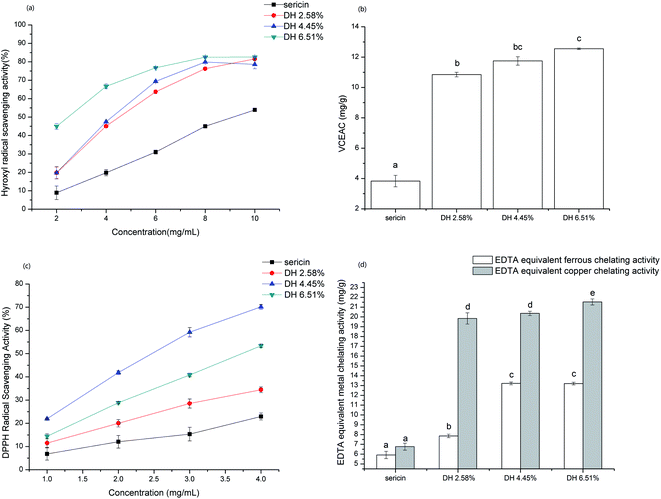 |
| Fig. 8 Antioxidant activity of sericin and its hydrolysates. (a) Hydroxyl radical scavenging activity. (b) ABTS radical scavenging activity. (c) DPPH radical scavenging activity. (d) Metal (Fe2+, Cu2+) chelating activity. Results are the means of triplicates and different letters on the bars indicate a statistical difference (p <0.05). | |
Hydroxyl radical is an extremely reactive oxygen species generated in the organism that can react with DNA and proteins, which gives birth to some physiological disorders in the human body.54 As shown in Fig. 8a hydrolysis remarkably enhanced the hydroxyl radical scavenging activity of sericin, which is in line with the common view that short-chain peptides have higher antioxidant capacity. Besides, the hydroxyl radical scavenging activity of samples is concentration-related in the tested range and at DH 6.51%, the highest scavenging activity reached 82.56%.
For ABTS assay that can determine the antioxidant capacity of both hydrophilic and lipophilic compounds, sericin hydrolysates exhibited significantly higher (p <0.05) vitamin C equivalent antioxidant capacity than sericin (Fig. 8b). The ABTS radical quenching activity enhanced with increasing DH and DH 6.51% showed the highest VCEAC (12.55 ± 0.05 mg g−1) among the samples tested.
The DPPH assay is mainly based on single electron transfer (SET) reaction in which DPPH radicals are neutralized by electron donation of antioxidants. Hydrolysate DH 4.45% exhibited remarkably higher DPPH radical scavenging activity than sericin and other hydrolysates (Fig. 8c). The nitrogen atom at the center of the DPPH molecule is the radical portion.55 Thus, short-chain sericin hydrolysates have the advantage to access the radical portion than sericin due to steric hindrances. Tryptophan act via the SET mechanism, therefore the higher DPPH radical scavenging activity of DH 4.45% than DH 6.51% might be attributed to having more exposure of aromatic group (including tryptophan) caused by hydrolysis.
3.15 Metal chelating activity
Iron and copper are transition metals, which are essential dietary minerals and play vital roles in oxygen transport and enzyme activity. However, transition metal ions also react with peroxide, producing radicals that might be initial of some damages in cells. Moreover, the chelation of antioxidants with transition metal ions can put off the reaction of the transition metal ion with peroxide, reducing oxidative damage. In the metal (ferrous and copper) chelating activity assay, results showed that hydrolysates display significantly higher (p <0.05) EDTA equivalent chelating capacity compared to native sericin (Fig. 8d). The EDTA equivalent metal chelating activity rose sharply by increasing DH from 0 to 2.58%. However, the increasing rate of EDTA equivalent metal chelating capacity of hydrolysates from DH 2.58% to 6.51% slowed down. Sericin is rich in aspartic acid, since carboxyl (COOH) binds with metal ions,56 the remarkably higher metal chelating activity of sericin hydrolysates can be attributed to increased exposure of carboxylic groups in the side chain of aspartic acid caused by hydrolysis.
4. Conclusion
The trypsin-induced enzymatic hydrolysis with low levels of DH (2.58%, 4.45%, and 6.51%) remarkably altered the structural and functional properties of sericin. The poor solubility of sericin was remarkably improved over the range from pH 2 to pH 10. The enzymatic hydrolysis decreased the gelation properties, water holding capacity, and oil holding capacity and changed emulsifying and foaming properties. Sericin hydrolysates showed significantly higher antioxidant capacity and metal chelating than untreated sericin. Sericin and its hydrolysates exhibited excellent function on oil holding capacity, emulsifying, and foaming. Sericin and its hydrolysates had clear effects on the growth of both Enterococcus faecalis and Lactobacillus bulgaricus strains. In summary, limited hydrolysis gave rise to positive effects on antioxidative and some functional properties of sericin. However, the decline in emulsifying and foaming properties of hydrolysate DH 6.51% suggests undue proteolysis is not favorable because it might negatively affect the functional properties. From a technological point of view, our study suggests that sericin hydrolysate DH 4.45% shows promise to be used in the food industry.
Conflicts of interest
There are no conflicts of interest to declare.
Acknowledgements
National Science and Technology Major Project of China [No. 2017ZX09301045], Xinjiang Branch, Chinese Academy of Sciences [No. 2019D01A94], and Young Western Scholars Program, the Chinese Academy of Sciences supported this work.
References
- N. M. Mahmoodi, M. Arami, F. Mazaheri and S. Rahimi, J. Cleaner Prod., 2010, 18, 146–151 CrossRef CAS.
- Z. Wang, Y. Zhang, J. Zhang, L. Huang, J. Liu, Y. Li, G. Zhang, S. C. Kundu and L. Wang, Sci. Rep., 2014, 4, 7064 CrossRef PubMed.
- T. Aewsiri, S. Benjakul, W. Visessanguan, J.-B. Eun, P. A. Wierenga and H. Gruppen, Food Chem., 2009, 117, 160–168 CrossRef CAS.
- U. C. Javali, N. V. Padaki, B. Das and K. B. Malali, in Advances in Silk Science and Technology, ed. A. Basu, Woodhead Publishing, 2015, pp. 261–270, DOI:10.1016/B978-1-78242-311-9.00013-6.
- M. Sasaki, H. Yamada and N. Kato, Nutr. Res., 2000, 20, 1505–1511 CrossRef CAS.
- H. Sun, Q. Chang, L. Liu, K. Chai, G. Lin, Q. Huo, Z. Zhao and Z. Zhao, J. Agric. Food Chem., 2017, 65, 10020–10028 CrossRef CAS PubMed.
- X. Dong, S.-X. Zhao, X.-L. Yin, H.-Y. Wang, Z.-G. Wei and Y.-Q. Zhang, Int. J. Biol. Macromol., 2020, 150, 1061–1071 CrossRef CAS PubMed.
- Z.-L. Zhao and Y.-Q. Zhang, J. Cleaner Prod., 2020, 261, 121080 CrossRef CAS.
- M. Sasaki, H. Yamada and N. Kato, Food Sci. Technol. Int., Tokyo, 2000, 6, 280–283 CAS.
- B. Zhu, P. Yang, N. Mammat, H. Ding, J. He, Y. Qian, J. Fei and K. Abdukerim, BMC Complementary Altern. Med., 2015, 15, 285 CrossRef PubMed.
- Y.-Q. Zhang, M.-L. Tao, W.-D. Shen, Y.-Z. Zhou, Y. Ding, Y. Ma and W.-L. Zhou, Biomaterials, 2004, 25, 3751–3759 CrossRef CAS PubMed.
- H. Kim, J. Lee and Y. Cho, Nutr. Res., 2012, 32, 956–964 CrossRef CAS PubMed.
- N. Akbari, J. Mohammadzadeh Milani and P. Biparva, J. Sci. Food Agric., 2020, 100, 1320–1327 CrossRef CAS PubMed.
- L. Beaulieu, S. Bondu, K. Doiron, L. Rioux and S. L. Turgeon, J. Funct. Foods, 2015, 17, 685–697 CrossRef CAS.
- B. C. Saha and K. Hayashi, Biotechnol. Adv., 2001, 19, 355–370 CrossRef CAS.
- J. Lee, Soy protein hydrolysate; solubility, thermal stability, bioactivity, and sensory acceptability in a tea beverage, master's degree thesis, 2011.
- P. Anand, J. P. Pandey and D. M. Pandey, J. Genet. Eng. Biotechnol., 2021, 19, 32 CrossRef PubMed.
- T.-T. Cao and Y.-Q. Zhang, Mater. Sci. Eng. Carbon, 2016, 61, 940–952 CrossRef CAS PubMed.
- M. Chevallet, S. Luche and T. Rabilloud, Nat. Protoc., 2006, 1, 1852–1858 CrossRef CAS PubMed.
- X. Zang, C. Yue, Y. Wang, M. Shao and G. Yu, J. Cereal Sci., 2019, 85, 168–174 CrossRef CAS.
- P. M. Nielsen, D. Petersen and C. Dambmann, J. Food Sci., 2001, 66, 642–646 CrossRef CAS.
- L. B. Pham, B. Wang, B. Zisu and B. Adhikari, Food Chem., 2019, 293, 463–471 CrossRef CAS PubMed.
- N. J. Greenfield, Nat. Protoc., 2006, 1, 2876–2890 CrossRef CAS PubMed.
- N. Sreerama and R. W. Woody, Anal. Biochem., 2000, 287, 252–260 CrossRef CAS PubMed.
- W.-G. Jin, H.-T. Wu, B.-W. Zhu and X.-Q. Ran, Eur. Food Res. Technol., 2012, 234, 863–872 CrossRef CAS.
- S.-W. Yin, C.-H. Tang, J.-S. Cao, E.-K. Hu, Q.-B. Wen and X.-Q. Yang, Food Chem., 2008, 106, 1004–1013 CrossRef CAS.
- P. J. García-Moreno, R. Pérez-Gálvez, F. J. Espejo-Carpio, C. Ruiz-Quesada, A. I. Pérez-Morilla, O. Martínez-Agustín, A. Guadix and E. M. Guadix, J. Sci. Food Agric., 2017, 97, 299–308 CrossRef PubMed.
- A. Gani, A. A. Broadway, F. A. Masoodi, A. A. Wani, S. Maqsood, B. A. Ashwar, A. Shah, S. A. Rather and A. Gani, J. Food Sci. Technol., 2015, 52, 7697–7709 CrossRef CAS PubMed.
- X.-q. Zheng, J.-t. Wang, X.-l. Liu, Y. Sun, Y.-j. Zheng, X.-j. Wang and Y. Liu, Food Chem., 2015, 172, 407–415 CrossRef CAS PubMed.
- D.-x. Jin, X.-l. Liu, X.-q. Zheng, X.-j. Wang and J.-f. He, Food Chem., 2016, 204, 427–436 CrossRef CAS PubMed.
- S. Kubglomsong, C. Theerakulkait, R. L. Reed, L. Yang, C. S. Maier and J. F. Stevens, J. Agric. Food Chem., 2018, 66, 8346–8354 CrossRef CAS PubMed.
- J. Su, Y.-Q. Sun, F.-J. Huo, Y.-T. Yang and C.-X. Yin, Analyst, 2010, 135, 2918–2923 RSC.
- N. A. Avramenko, N. H. Low and M. T. Nickerson, Food Res. Int., 2013, 51, 162–169 CrossRef CAS.
- S. Dutta, T. Chowdhury and A. Kumar Ghosh, Colloids Surf., B, 2020, 188, 110822 CrossRef CAS PubMed.
- X. Xu, W. Liu, C. Liu, L. Luo, J. Chen, S. Luo, D. J. McClements and L. Wu, Food Hydrocolloids, 2016, 61, 251–260 CrossRef CAS.
- T. Li, C. Wang, T. Li, L. Ma, D. Sun, J. Hou and Z. Jiang, Molecules, 2018, 23(9), 2383 CrossRef PubMed.
- J. Jia, B. Ji, L. Tian, M. Li, M. Lu, L. Ding, X. Liu and X. Duan, Food Hydrocolloids, 2021, 111, 106218 CrossRef CAS.
- P. Mudgal, C. R. Daubert, D. A. Clare and E. A. Foegeding, J. Agric. Food Chem., 2011, 59, 1491–1497 CrossRef CAS PubMed.
- M. L. Gulrajani, R. Purwar, R. K. Prasad and M. Joshi, J. Appl. Polym. Sci., 2009, 113, 2796–2804 CrossRef CAS.
- J. P. Kumar and B. B. Mandal, Free Radicals Biol. Med., 2017, 108, 803–818 CrossRef CAS PubMed.
- R. Mozafarpour, A. Koocheki, E. Milani and M. Varidi, Food Hydrocolloids, 2019, 93, 361–373 CrossRef CAS.
- J. Zayas, Functionality of Proteins in Food, Springer-Verlag, Berlin Heidelberg, 1997 Search PubMed.
- B. Purschke, P. Meinlschmidt, C. Horn, O. Rieder and H. Jäger, Eur. Food Res. Technol., 2018, 244, 999–1013 CrossRef CAS.
- M. J. Jang and I. C. Um, Eur. Polym. J., 2017, 93, 761–774 CrossRef CAS.
- C. J. Park, J. Ryoo, C. S. Ki, J. W. Kim, I. S. Kim, D. G. Bae and I. C. Um, Int. J. Biol. Macromol., 2018, 119, 821–832 CrossRef CAS PubMed.
- R. I. Kunz, R. M. C. Brancalhão, L. d. F. C. Ribeiro and M. R. M. Natali, BioMed Res. Int., 2016, 2016, 8175701 Search PubMed.
- J. Miedzianka, A. Pęksa, M. Pokora, E. Rytel, A. Tajner-Czopek and A. Kita, Food Chem., 2014, 159, 512–518 CrossRef CAS PubMed.
- F. M. Diniz and A. M. Martin, LWT–Food Sci. Technol., 1997, 30, 266–272 CrossRef CAS.
- R. S. H. Lam and M. T. Nickerson, Food Chem., 2013, 141, 975–984 CrossRef CAS PubMed.
- J. A. Lopes-da-Silva and S. R. Monteiro, Food Chem., 2019, 294, 216–223 CrossRef CAS PubMed.
- V. Klompong, S. Benjakul, D. Kantachote and F. Shahidi, Food Chem., 2007, 102, 1317–1327 CrossRef CAS.
- X. N. Wang, M. Qin, Y. Y. Feng, J. K. Chen and Y. S. Song, J. Sci. Food Agric., 2017, 97, 4235–4241 CrossRef CAS PubMed.
- C. Zhang, Y. Zhang, H. Li and X. Liu, Food Funct., 2020, 11, 1946–1957 RSC.
- L. You, M. Zhao, C. Cui, H. Zhao and B. Yang, Innovative Food Sci. Emerging Technol., 2009, 10, 235–240 CrossRef CAS.
- R. L. Prior, X. Wu and K. Schaich, J. Agric. Food Chem., 2005, 53, 4290–4302 CrossRef CAS PubMed.
- V. Conway, S. F. Gauthier and Y. Pouliot, J. Agric. Food Chem., 2013, 61, 364–372 CrossRef CAS PubMed.
Footnote |
† Electronic supplementary information (ESI) available. See DOI: 10.1039/d1ra03772b |
|
This journal is © The Royal Society of Chemistry 2021 |