DOI:
10.1039/D1RA03621A
(Paper)
RSC Adv., 2021,
11, 21851-21856
A GGA + U investigation into the effects of cations on the electromagnetic properties of transition metal spinels†
Received
9th May 2021
, Accepted 7th June 2021
First published on 21st June 2021
Abstract
Spinel oxides are promising low-cost catalysts with manifold and controllable physicochemical properties. Trial and error strategies cannot achieve the effective screening of high-performance spinel catalysts. Therefore, unraveling the structure–performance relationship is the foundation for their rational design. Herein, the effects of cations in tetrahedral and octahedral sites on the electronic structures of spinels were systematically investigated using GGA + U calculations based on ACr2O4 (A = Mn, Fe, Co, Ni, and Zn) and Zn/LiB2O4 (B = Cr, Mn, Fe, Co and Ni). The results indicate that the octahedrally coordinated B cations have notable influence on the electronic structures of spinels. The Jahn–Teller active ions Fe2+, Ni2+, Mn3+, Ni3+, Cr4+ and Fe4+ can remarkably reduce the band gaps of spinels and even change their electroconductibilities. These results will provide theoretical insights into the electronic properties of 3d transition metal spinels.
Introduction
Spinels were initially regarded as precious stones such as the famous Black Prince's Ruby and Timur Ruby. In 1915, the first report of the crystal structure of a spinel lifted the veil of gems.1 The spinel structure is formulated AB2X4, where A and B are tetrahedrally and octahedrally coordinated metal cations, respectively, and X is an anion (typically O or F). Fig. 1A presents the structural model of normal AB2O4, where O2− has a close-packed array and A2+ and B3+ ions are in tetrahedral and octahedral cages formed by the oxygens, respectively. Each unit cell contains eight formula units and has a composition of A8B16O32.
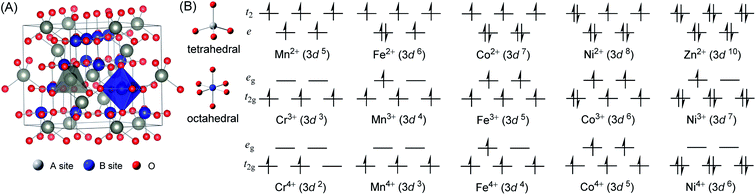 |
| Fig. 1 (A) The geometric structure of AB2O4 spinel. The A2+ ions (grey spheres) and the B3+ ions (light blue spheres) are in tetrahedral and octahedral cages formed by the oxygen atoms (red spheres). (B) Electron configurations of A2+ and B3+ ions with increasing 3d electron. | |
Spinels contain a large number of species and embody almost all main group metals and transition metals.2,3 The benefits of spinels, such as tunable composition, electron configurations, valence and magnetic states as well as low cost, make them increasingly attractive in optical, electromagnetic, information technology, environmental science, energy storage and conversion fields.4–6 Recently, their intrinsic enzyme-mimicking activities, such as peroxidase-, oxidase-, superoxide dismutase- and catalase-like activities, cast light on broad biomedical applications.7–19
However, trial and error strategies cannot achieve the effective screening of high-performance spinels, which hinder the rational design spinel catalysts. Recently, Xu et al. developed cations in octahedral sites as an effective descriptor to predict spinel catalysts with high oxygen-reduction reaction (ORR) and oxygen-evolution reaction (OER) performance.20 Although progresses have been achieved,21–25 efforts are still needed to reveal the unambiguous structure–function relationship. The rational design roots in the physicochemical properties. According to previous reports, the electronic configuration of metal cations,26 the occupation of tetrahedral and octahedral sites20 and the spin orders22,23,27 are key factors affecting the geometric and electronic properties of spinels.
Herein, to investigate the effects of cations in tetrahedral and octahedral sites on the electronic structures of spinels, three series of spinels (Fig. 1B), where the metals on A and B sites have continuously varying electronic conformations, namely ACr2O4 (A = Mn, Fe, Co, Ni, and Zn) and Zn/LiB2O4 (B = Cr, Mn, Fe, Co and Ni), were investigated as the model system. The B cations in the LiB2O4 series have two valence states, particularly half B3+ and half B4+. The results indicate that the magnetism of spinels depends on the indirect exchange of cations (JMM). The configuration of octahedrally coordinated B cations has notable influence on the electronic structures of spinels. The results presented here provide insights into the physicochemical properties of spinels.
Methods
Calculations were performed under periodic boundary conditions employing the Vienna Ab initio Simulation Package (VASP).28 Projector-augmented wave (PAW)29 pseudopotentials and Perdew–Burke–Ernzerhof (PBE)30 exchange-correlation functional of generalized gradient approximation (GGA) were used to describe the interactions between core electrons. Both geometric optimizations and density of states (DOS) analysis were performed using a standard Monkhorst–Pack grid31 sampling at 7 × 7 × 7. An energy cut-off was set to 450 eV and the convergence thresholds for the electronic structure and forces were set to 10−5 eV and 0.02 eV Å−1, respectively. The Hubbard U correction32,33 was used to make the strong coulomb interactions of d electrons more accurate. Following a literature survey,25,34–36 the Ueff values used for 3d metals are shown in Table S1.†
Results and discussion
To verify the ground states for spinels, initial magnetic states illustrated in Fig. 2 with different spin orders were set for geometric optimizations. All initial structures with eight formula units in one unit cell are crystallized in the Fd
m space group (No. 227). The calculated energies for all magnetic states are listed in Table S1,† and the geometric structures of the ground states are illustrated in Figure S1.† The optimized physicochemical characteristics including the ground state (GS), lattice parameters, band gap (Eg) and magnetic moments on tetrahedral and octahedral metal atoms (MO and MT) are listed in Table 1.
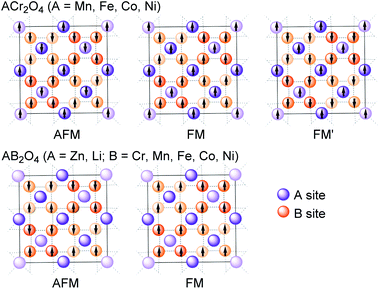 |
| Fig. 2 Magnetic states initially set for optimizations. There are three initial states, namely AFM, FM and FM′, for ACr2O4 (A = Mn, Fe, Co, and Ni). The net magnetic moments for A, B and the whole bulk are zero in the AFM state. In the FM state, the magnetic moments of A and B are in the same direction, while in the opposite directions in the FM′ state. There are two magnetic states for Zn/LiB2O4 (B = Cr, Mn, Fe, Co, and Ni) since Zn2+ and Li+ have no unpaired 3d electron. | |
Table 1 Summary of the calculated ground states (GS), lattice parameters, band gaps (Eg, in eV) and the magnetic moments (μ) on the metal atoms in tetrahedral (MT) and octahedral (MO) environmentsa
Spinel |
GS |
Lattice parameters |
Eg |
Magnetic moment (μ) |
Cal. |
Exp. |
δ (%) |
Theor. |
Exp. |
μMT |
n3d_MT |
μMO |
n3d_MO |
The number of unpaired 3d electrons (n3d) of the metals are derived from μ . The lattice parameters measured by experiments are also listed for comparison. |
MnCr2O4 |
AFM |
8.46 |
8.4445 |
0.237 |
2.91 |
2.5046 |
4.56 |
3.67 |
2.95 |
2.11 |
FeCr2O4 |
FM′47 |
a = c = 8.41, b = 8.39 |
8.3848 |
0.239 |
2.15 |
1.9549 |
3.69 |
2.82 |
2.95 |
2.11 |
2.9049 |
CoCr2O4 |
FM′50 |
8.36 |
8.3350 |
0.360 |
2.87 |
2.7751 |
2.70 |
1.88 |
2.95 |
2.11 |
NiCr2O4 |
FM′35 |
a = 8.30, b = c = 8.36 |
a = 8.18, b = 8.17, c = 8.5727 |
— |
2.39 |
1.7052 |
1.75 |
1.42 |
2.96 |
2.12 |
ZnCr2O4 |
AFM53 |
8.34 |
8.3153 |
0.361 |
3.30 |
1.6654 |
0.0 |
0.00 |
2.95 |
2.11 |
3.6155 |
ZnMn2O4 |
AFM53 |
a = b = 8.63, c = 8.0 |
a = b = 8.10, c = 9.2453 |
— |
0.22 |
1.2856 |
0.00 |
0.00 |
3.79 |
2.92 |
ZnFe2O4 |
AFM53 |
8.39 |
8.4657 |
0.827 |
1.60 |
1.7858 |
0.00 |
0.00 |
4.25 |
3.37 |
ZnCo2O4 |
AFM |
a = c = 8.38, b = 8.08 |
8.0553 |
— |
1.11 |
1.7159 |
0.00 |
0.00 |
3.25 |
2.40 |
2.7759 |
ZnNi2O4 |
FM |
8.17 |
— |
— |
— |
|
0.00 |
0.00 |
1.39 |
0.71 |
LiCr2O4 |
AFM |
a = c = 8.16, b = 8.24 |
8.1160 |
— |
1.03 |
|
0.00 |
0.00 |
2.90/2.03 |
2.07/1.26 |
LiMn2O4 |
AFM61 |
a = c = 8.32, b = 8.00 |
8.2562,63 |
— |
0.69 |
0.5864 |
0.00 |
0.00 |
3.77/2.96 |
2.90/2.12 |
LiFe2O4 |
AFM |
a = b = 8.32, c = 8.05 |
— |
— |
— |
|
0.00 |
0.00 |
4.20/3.61 |
3.32/2.75 |
LiCo2O4 |
AFM26 |
a = 8.06, b = c = 8.19 |
7.99,26 8.15365 |
— |
0.24 |
|
0.00 |
0.00 |
3.45/2.78 |
2.59/1.95 |
LiNi2O4 |
FM |
a = 8.06, b = c = 7.80 |
8.0466 |
— |
0.44 |
|
0.00 |
0.00 |
1.05/0.01 |
0.45/0.00 |
Ferrite spinels are of technological interest because of their magnetic ordering. How the unpaired spins of metal ions are coupled determines the magnetism of spinels. The 2nd column of Table 1 lists the magnetic orders for the ground states of the spinels. The Goodenough–Kanamori rules37–44 predict the local magnetism, which results from the superexchange coupling of the electron spins of transition metal ions. The O2− ions shared by two metal ions can mediate the coupling of spins by superexchange, as shown in Fig. 3.
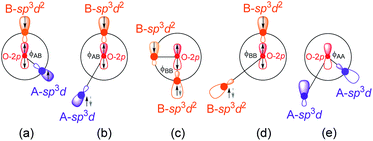 |
| Fig. 3 Five cases of indirect exchange interactions between A–B (a and b), B–B (c and d) and A–A (e). | |
In spinel, each magnetic ion at site A has 12 magnetic ions at site B to undergo A–B exchanges and each B site ion has 6 A site ions undergo A–B exchanges. Moreover, the angles between A and B (ϕAB) are close to 180° and the distances between A and B are the shortest. Therefore, the A–B exchanges (Fig. 3a and b) are the strongest, followed by B–B exchanges (Fig. 3c and d) and A–A (Fig. 3e) exchanges are the weakest. The above rules can profoundly explain the magnetic ground states of spinels listed in Table 1. For ACr2O4 (A = Mn, Fe, Co, and Ni), the exchange between A and its nearest B are the strongest leading to opposite spin orders on A and B. If the A–B and B–B exchanges (Fig. 3b–d) are strong enough to oppositely arrange the spin orders of A–B and B–B, the final magnetism of the spinel will be AFM like MnCr2O4, where the Mn2+ has the largest net spins, as shown in Fig. 1b. Spinels ACr2O4 (A = Fe, Co, and Ni) have strong A–B but weak B–B exchanges, which finally leads to the FM′ magnetic states. For Zn/LiB2O4 (A = Zn and Li; B = Cr, Mn, Fe, Co, and Ni), the B–B exchanges are the dominating interactions because Zn2+ and Li+ have fully and empty occupied 3d orbitals.
The B–B exchanges in Zn/LiB2O4 (B = Cr, Mn, Fe, and Co) are stronger than those in Zn/LiNi2O4, which leads to their AFM and FM states.
The 3–4 columns of Table 1 list the lattice parameters for the spinels calculated in present work and as reported by previous experiments. The relative errors (δ) between them are less than 1.00%. In ACr2O4 (A = Fe, Co, and Ni), A = Fe and Ni are Jahn–Teller active ions23 with orbital degeneracy and slightly collective distortion are observed in the relaxed unit cell. For spinels with nonmagnetic A site ions, namely Zn/LiB2O4 (B = Cr, Mn, Fe, and Co), the antiferromagnetic JB–B exchange interactions lead to highly frustrated ground states on the pyrochlore sublattice. This frustration can be released by the spin-Jahn–Teller effect67,68 and lead to lattice distortions. The calculated magnetic moments (μ) on MO and MT are also listed in Table 1. The number of unpaired 3d electrons (n3d) can be derived from
. Although the calculated n3d are smaller than the theoretical values illustrated in Fig. 1B, it does not affect the reliability of the calculations since the rational tendency is predicted.
To investigate the influences induced by cation A and B on the electronic structures of spinels, we calculated the projected density of states (PDOS) of ACr2O4 (A = Mn, Fe, Co, Ni, Zn) and Zn/LiB2O4 (B = Cr, Mn, Fe, Co and Ni). The band gaps were also calculated and are listed in Table 1. Fig. 4A presents the PDOS for ACr2O4 (A = Mn, Fe, Co, Ni, and Zn), which indicate that cation A has limited impact on the electronic structures of spinels. The Eg of ACr2O4 differs in a narrow range from 2.30 to 3.30 eV. Mn/Co/ZnCr2O4 has Jahn–Teller inactive A ions and thus have similar electronic structures. The Jahn–Teller active Fe2+ and Ni2+ can reduce Eg because the e3 configuration of Fe2+ raises the valence-band maximum (VBM) and the t2g4 configuration of Ni2+ lowers the conduction band minimum (CBM). The above-mentioned results can be verified by the fact that the partial charge populations, corresponding to the VBM of FeCr2O4 and the CBM of NiCr2O4 are mainly contributed by the e orbital of Fe2+ and the t2 orbital of Ni2+.
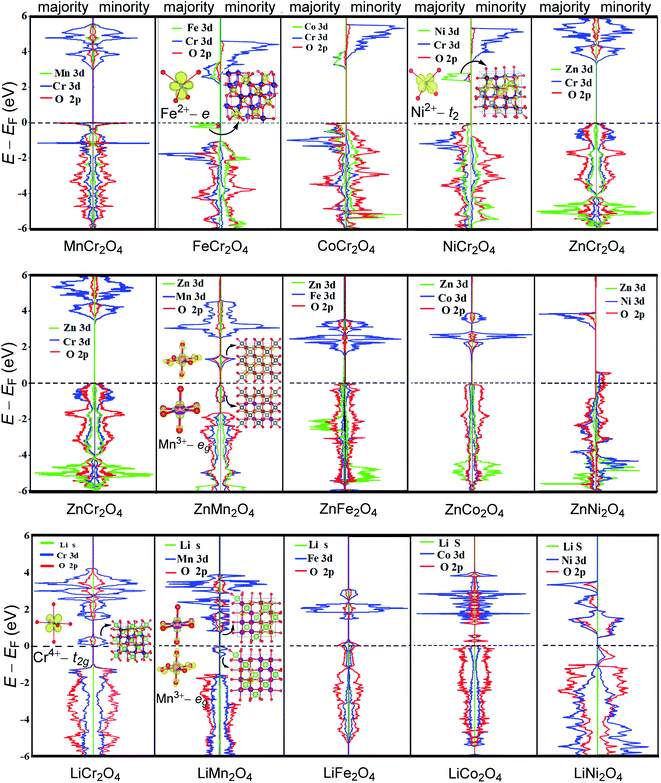 |
| Fig. 4 The spin-resolved PDOS of ACr2O4 (A = Mn, Fe, Co, Ni, and Zn) and Zn/LiB2O4 (B = Cr, Mn, Fe, Co and Ni). The partial charge density populations corresponding to some specific states are also illustrated. | |
Fig. 4B and C indicate that the electronic configuration of cation B has notable influence on the electronic structure of spinel. The Jahn–Teller active Mn3+, Ni3+, Cr4+ and Fe4+ can remarkably reduce the Eg of spinels and even change the semiconductor to metal and half-metal. The notable differences between ZnCr2O4 and LiCr2O4 indicate that the Jahn–Teller active Cr4+in LiCr2O4 lowers the CBM to the Fermi level and notably narrows the band gap of LiCr2O4. The reason is that CBM in ZnCr2O4 is contributed by the eg orbital but by the t2g orbital with a lower energy level in LiCr2O4. In ZnMn2O4, VBM and CBM are contributed by the two eg orbitals, namely dz2 and dx2−y2, of Mn3+. The introduction of Mn4+ in LiMn2O4 does not change the contributions of eg orbitals to VBM and CBM, and thus, LiMn2O4 has similar Eg with ZnMn2O4. Compared with ZnFe2O4, the extra electron on the eg orbital of Fe4+ raises the VBM to the Fermi level and makes LiFe2O4 a metal. Analogously, the single eg electron of Ni3+ makes ZnNi2O4 a half-metal, while LiNi2O4 is a semiconductor because Ni4+ is a Jahn–Teller inactive ion with fully occupied t2g orbitals but empty eg orbitals. To evaluate the quality of the above-mentioned quantum chemical calculations, we compared the present results with experimental data and listed the theoretically calculated and experimentally measured band gaps in Table 1. The scatter of the band gap values is associated with different determining methods. Most of the Exp. Eg values listed in Table 1 are optical band gaps obtained by UV-Vis absorption49,51,54–56,58,59,64 or diffuse reflectance spectroscopy.46,52 The values indicate that the calculated band gaps of FeCr2O4, CoCr2O4, ZnCr2O4, ZnFe2O4, ZnCo2O4 and LiMn2O4 accord well with the experimental measured values with errors within 0.5 eV.
In summary, the configuration of cation B has notable influence on the electronic structure of spinels. The Jahn–Teller active ions Fe2+, Ni2+, Mn3+, Ni3+, Cr4+ and Fe4+ can remarkably regulate the Eg of spinels and even change the electroconductibility.
Conclusions
The spinels are promising low-cost catalysts. Unravelling the structure–performance relationship is the foundation to realize the rational design of spinel-based catalysts. Herein, the effects of cations in tetrahedral and octahedral sites on the electronic structures of spinels were systematically investigated via GGA + U calculations. Three series of spinels with varying A and B, namely ACr2O4 (A = Mn, Fe, Co, Ni, and Zn) and Zn/LiB2O4 (B = Cr, Mn, Fe, Co and Ni), were elected as the model system. The results indicate that the magnetism of spinels depends on the indirect exchanges of cations (JMM). The configurations of B cations in an octahedral O environment, rather than the A in tetrahedral ones have notable influence on the electronic structures of spinels. The Jahn–Teller active ions Fe2+, Ni2+, Mn3+, Ni3+, Cr4+ and Fe4+ can remarkably regulate the band gaps of spinels and even change their electroconductibilities. The results will provide theoretical insights into the electronic properties of 3d transition metal spinels.
Author contributions
Chunyu Li: data curation, investigation, writing – original draft. Peng Li: investigation. Leyun Li: investigation. Dingjia Wang: investigation. Xingfa Gao: supervision. Xuejiao J. Gao: project administration, writing – review & editing.
Conflicts of interest
There are no conflicts to declare.
Acknowledgements
This work was supported by the National Natural Science Foundation of China (NSFC) Project (21907043 and 21773095), the Natural Science Foundation of Jiangxi, China (20202BABL213013).
Notes and references
- W. H. Bragg, Philos. Mag., 1915, 30, 305–315 CAS.
- M. G. Brik, A. Suchocki and A. Kamińska, Inorg. Chem., 2014, 53, 5088–5099 CrossRef CAS PubMed.
- Q. Zhao, Z. Yan, C. Chen and J. Chen, Chem. Rev., 2017, 117, 10121–10211 CrossRef CAS PubMed.
- N. R. D. Fino, G. Saracco and V. Specchia, J. Catal., 2006, 242, 38–47 CrossRef.
- Q. Chen, Z. Tang, H. Lia, Q. Zhao and B. Pana, Chem. Eng. J., 2020, 381, 122656 CrossRef CAS.
- G. S. H. Michael Woodhouse and B. A. Parkinson, Chem. Mater., 2005, 17, 4318–4324 CrossRef.
- D. Bhattacharya, A. Baksi, I. Banerjee, R. Ananthakrishnan, T. K. Maiti and P. Pramanik, Talanta, 2011, 86, 337–348 CrossRef CAS PubMed.
- N. Dhandapani, A. Rebekah, C. Viswanathan and P. Nagamony, Mater. Res. Bull., 2017, 95, 1–8 CrossRef.
- V. Figueroa-Espí, A. Alvarez-Paneque, M. Torrens, A. J. Otero-González and E. Reguera, Colloids Surf., A, 2011, 387, 118–124 CrossRef.
- C. M. Hussain and B. Kharisov, Advanced Environmental Analysis. Applications of Nanomaterials, 2016 Search PubMed.
- S. Mumtaz, L.-S. Wang, M. Abdullah, S. Z. Hussain, Z. Iqbal, V. M. Rotello and I. Hussain, J. Phys. D: Appl. Phys., 2017, 50, 11LT02 CrossRef.
- X. Niu, Y. Xu, Y. Dong, L. Qi, S. Qi, H. Chen and X. Chen, J. Alloy. Compd., 2014, 587, 74–81 CrossRef CAS.
- Y. Peng, Z. Wang, W. Liu, H. Zhang, W. Zuo, H. Tang, F. Chen and B. Wang, Dalton Trans., 2015, 44, 12871–12877 RSC.
- R. Sahoo, S. Santra, C. Ray, A. Pal, Y. Negishi, S. K. Ray and T. Pal, New J. Chem., 2016, 40, 1861–1871 RSC.
- W. Shi, X. Zhang, S. He and Y. Huang, Chem. Commun., 2011, 47, 10785–10787 RSC.
- L. Su, W. Qin, H. Zhang, Z. U. Rahman, C. Ren, S. Ma and X. Chen, Biosens. Bioelectron., 2015, 63, 384–391 CrossRef CAS PubMed.
- A. A. Vernekar, T. Das, S. Ghosh and G. Mugesh, Chem.–Asian J., 2016, 11, 72–76 CrossRef CAS PubMed.
- Z. Yang, F. Ma, Y. Zhu, S. Chen, C. Wang and X. Lu, Dalton Trans., 2017, 46, 11171–11179 RSC.
- K. Zhang, W. Zuo, Z. Wang, J. Liu, T. Li, B. Wang and Z. Yang, RSC Adv., 2015, 5, 10632–10640 RSC.
- C. Wei, Z. Feng, G. G. Scherer, J. Barber, Y. Shao-Horn and Z. J. Xu, Adv. Mater., 2017, 29, 1606800 CrossRef PubMed.
- S. H. Lee, C. Broholm, T. H. Kim, W. Ratcliff and S. W. Cheong, Phys. Rev. Lett., 2000, 84, 3718–3721 CrossRef CAS PubMed.
- A. B. Sushkov, O. Tchernyshyov, W. R. Ii, S. W. Cheong and H. D. Drew, Phys. Rev. Lett., 2005, 94, 137202 CrossRef CAS PubMed.
- V. Kocsis, S. Bordács, D. Varjas, K. Penc, A. Abouelsayed, C. A. Kuntscher, K. Ohgushi, Y. Tokura and I. Kézsmárki, Phys. Rev. B: Condens. Matter Mater. Phys., 2013, 87, 064416 CrossRef.
- F. Zhou, M. Cococcioni, C. A. Marianetti, D. Morgan and G. Ceder, Phys. Rev. B: Condens. Matter Mater. Phys., 2004, 70, 235121 CrossRef.
- F. Zasada, J. Grybos, P. Indyka, W. Piskorz, J. Kaczmarczyk and Z. Sojka, J. Phys. Chem. C, 2014, 118, 19085–19097 CrossRef CAS.
- V. B. O. Peña, T. Guizouarn, E. Meza and J. L. Gautier, Bol. Soc. Esp., 2004, 43, 736–739 CrossRef.
- M. R. Suchomel, D. P. Shoemaker, L. Ribaud, M. C. Kemei and R. Seshadri, Phys. Rev. B: Condens. Matter Mater. Phys., 2012, 86, 054406 CrossRef.
- G. Kresse, Phys. Rev. B: Condens. Matter Mater. Phys., 1996, 54, 11169–11186 CrossRef CAS PubMed.
- P. E. Blöchl, Phys. Rev. B: Condens. Matter Mater. Phys., 1994, 50, 17953–17979 CrossRef PubMed.
- J. P. Perdew, K. Burke and M. Ernzerhof, Phys. Rev. Lett., 1996, 77, 3865–3968 CrossRef CAS PubMed.
- H. J. Monkhorst and J. D. Pack, Phys. Rev. B: Solid State, 1976, 13, 5188–5192 CrossRef.
- V. V. Anisimov, J. Zaanen and O. K. Andersen, Phys. Rev. B: Condens. Matter Mater. Phys., 1991, 44, 943–954 CrossRef CAS PubMed.
- S. L. Dudarev, G. A. Botton, S. Y. Savrasov, C. J. Humphreys and A. P. Sutton, Phys. Rev. B: Condens. Matter Mater. Phys., 1998, 57, 1505–1509 CrossRef CAS.
- D. Debashish and S. Ghosh, J. Phys. D: Appl. Phys., 2015, 48, 425001 CrossRef.
- D. Andersson and C. Stanek, Phys. Chem. Chem. Phys., 2013, 15, 15550–15564 RSC.
- D. Santos-Carballal, A. Roldan, R. Grau-Crespo and N. H. de Leeuw, Phys. Rev. B: Condens. Matter Mater. Phys., 2015, 91, 195106 CrossRef.
- J. S. Zhou and J. B. Goodenough, Phys. Rev. Lett., 2006, 96, 247202 CrossRef PubMed.
- K. Ueda, H. Tabata and T. Kawai, Science, 1998, 280, 1064 CrossRef CAS PubMed.
- J. Kanamori, J. Appl. Phys., 1960, 31, S14–S23 CrossRef.
- J. Kanamori, J. Phys. Chem. Solids, 1959, 10, 87–98 CrossRef CAS.
- J. Kanamori, Prog. Theor. Phys., 1957, 17, 177–196 CrossRef CAS.
- J. B. Goodenough and J. S. Zhou, in Localized to Itinerant Electronic Transition in Perovskite Oxides, Springer Berlin Heidelberg, Berlin, Heidelberg, 2001 Search PubMed.
- J. B. Goodenough, J. Phys. Chem. Solids, 1958, 6, 287–297 CrossRef CAS.
- J. B. Goodenough, Phys. Rev., 1955, 100, 564–573 CrossRef CAS.
- N. Mufti, A. A. Nugroho, G. R. Blake and T. T. M. Palstra, J. Phys.: Condens. Matter, 2010, 22, 075902 CrossRef CAS PubMed.
- V. Kocsis, S. Bordács, D. Varjas, K. Penc, A. Abouelsayed, C. A. Kuntscher, K. Ohgushi, Y. Tokura and I. Kézsmárki, Phys. Rev. B: Condens. Matter Mater. Phys., 2013, 87, 064416 CrossRef.
- C. Benhalima, S. Amari, L. Beldi and B. Bouhafs, Spin, 2019, 09, 1950014 CrossRef CAS.
- D. E. C. G. Shirane and S. J. Pickart, J. Appl. Phys., 1964, 35, 954 CrossRef.
- T. L. S. L. Wijesighe and D. J. Blackwood, J. Phys.: Conf. Ser., 2006, 28, 74–78 CrossRef.
- M. Akyol, İ. Adanur, A. O. Ayaş and A. Ekicibil, Phys. B, 2017, 525, 144–148 CrossRef CAS.
- I. Efthimiopoulos, Z. T. Y. Liu, S. V. Khare, P. Sarin, T. Lochbiler, V. Tsurkan, A. Loidl, D. Popov and Y. Wang, Phys. Rev. B: Condens. Matter Mater. Phys., 2015, 92, 064108 CrossRef.
- M. Enhessari, A. Salehabadi, S. Khanahmadzadeh, K. Arkat and J. Nouri, High Temp. Mater. Processes, 2017, 36, 121–125 CAS.
- H. J. Kim, I. C. Song, J. H. Sim, H. Kim, D. Kim, Y. E. Ihm and W. K. Choo, Solid State Commun., 2004, 129, 627–630 CrossRef CAS.
- Y. Benrighi, N. Nasrallah, T. Chaabane, V. Sivasankar, A. Darchen and O. Baaloudj, Opt. Mater., 2021, 115, 111035 CrossRef CAS.
- Q. Wang, J. Chen, H. Lu, P. Huang, J. Wang, M. Li, Y. Lu, G. Chang, Z. C. Feng and Y. He, Appl. Surf. Sci., 2019, 475, 820–827 CrossRef CAS.
- R. Gherbi, Y. Bessekhouad and M. Trari, J. Phys. Chem. Solids, 2016, 89, 69–77 CrossRef CAS.
- W. P. W. Schiessl, H. Karzel, M. Steiner and G. M. Kalvius, Phys. Rev. B: Condens. Matter Mater. Phys., 1996, 53, 9143–9152 CrossRef CAS PubMed.
- N. Kislov, S. S. Srinivasan, Y. Emirov and E. K. Stefanakos, Mater. Sci. Eng., B, 2008, 153, 70–77 CrossRef CAS.
- H. Guo, J. Chen, W. Weng, Q. Wang and S. Li, Chem. Eng. J., 2014, 239, 192–199 CrossRef CAS.
- M. Lauer, R. Valenti, H. Kandpal and R. Seshadri, Phys. Rev. B: Condens. Matter Mater. Phys., 2004, 69, 75117 CrossRef.
- C. Y. Ouyanga, S. Q. Shib and M. S. Leid, J. Alloys Compd., 2009, 474, 370–374 CrossRef.
- D. Santos-Carballal, P. Ngoepe and N. Leeuw, Phys. Rev. B, 2018, 97, 085126 CrossRef CAS.
- G. R. J. Rodríguez-Carvajal, Masquelier and M. Hervieu, Phys. Rev. Lett., 1998, 81, 4660–4663 CrossRef.
- V. Paulraj, B. Swami and K. Kamala Bharathi, Appl. Phys. Lett., 2019, 115, 093901 CrossRef.
- Y. Mouhib, M. Belaiche, C. A. Ferdi, M. Lacham and A. Elacham, New J. Chem., 2020, 44, 2538–2546 RSC.
- M. G. S. R. Thomas, W. I. F. David and J. B. Goodenough, Mater. Res. Bull., 1985, 93, 473–476 Search PubMed.
- O. Tchernyshyov, R. Moessner and S. L. Sondhi, Phys. Rev. Lett., 2002, 88, 067203 CrossRef PubMed.
- Y. Yamashita and K. Ueda, Phys. Rev. Lett., 2000, 85, 4960–4963 CrossRef CAS PubMed.
Footnote |
† Electronic supplementary information (ESI) available: The Hubbard Ueff (eV) values employed for metal ions, the calculated energies for spinels with different magnetic states and the optimized structures for the spinels. See DOI: 10.1039/d1ra03621a |
|
This journal is © The Royal Society of Chemistry 2021 |
Click here to see how this site uses Cookies. View our privacy policy here.