DOI:
10.1039/D1RA03608D
(Paper)
RSC Adv., 2021,
11, 26644-26654
Calix[4]amido crown functionalized visible sensors for cyanide and iodide anions†
Received
8th May 2021
, Accepted 29th July 2021
First published on 4th August 2021
Abstract
This study comprises the design and development of calix[4] arene-amido-based ionophores by varying structural stringency and steric hindrance at the lower rim to probe the anion sensing properties. The ionophores are prepared, purified, and characterized using various analytical techniques. The molecular structure of the most active ionophore I is established by single-crystal X-ray characterisation. Out of various anions investigated, iodide and cyanide show the highest sensitivity towards the ionophores investigated. Both anions are sensitive enough to give a visibly distinct color change. The binding properties of the ionophores are established with 1H & 127I NMR, fluorescence, and UV-vis spectroscopy, revealing that three ionophores strongly interact with CN− and I−. The binding constants are calculated via Benesi–Hildebrand plots using absorption data. The time-dependent 1H NMR revealed strong hydrogen bonding between the OH and NH groups of the ionophore and cyanide anion. The 127I NMR shows the highest 27.6 ppm shift after 6 h for ionophore I. The crystal structure revealed hydrogen bonding of N–H protons of the amide pendulum and phenolic oxygen of the calix rim. The Job's plot depicted the possibility of a 1
:
1 complex of ionophores with both anions.
Introduction
Supramolecular based anion-selective visual sensors have drawn significant attention in the past two decades1 due to their vital role in the environment and biological systems. The detection of anions is more challenging than cations due to their bigger size, structural specificity, basicity, and nucleophilicity2. Among various water-soluble anions, biologically important cyanide (CN−) and iodide (I−) are of great interest. Cyanide is highly toxic because of its deleterious effects on human health in minute quantities. Cyanide is also involved in chemicals, polymers, metal mining industries, and chemical warfare agents.3 As per the WHO guidelines, the maximum allowed cyanide concentration in drinking water is two ppb per liter.4,5
In contrast, iodine is an essential micronutrient for the human body, responsible for producing thyroid hormones. Iodine deficiency can also cause enlarged thyroid glands, thyroid cancer, and pregnancy complications for babies. Excessive ingestion can lead to hyperthyroidism diseases like Graves' disease.6 Thus, there is a dire need to develop easy, quick, and selective sensors in diverse samples.7 Despite the fact that several instrumental methods have been developed for the detection of cyanide and iodide ions, they are time-consuming, expensive, and cumbersome due to portability, calibration, and sample preparation. Hence there is a great need to develop colorimetric chemosensors for anions like cyanide and iodide, which are low cost and nontoxic and can be used with ease. Recently impressive advances have been made towards the development of visible cyanide sensors introducing molecules and materials such as pillararene,8 naphthofuran carbohydrazide,9 supramolecular gel,10 naphthalene,11 cyclodextrin,12 curcumin13 chromone,14 pyrene-thiacalix[4]arenes,15 calix[4]arene–naphthalimide,16 calix[3]pyrrole,17 Schiff bases,18 nitrobenzoxadiazole,19 and organogelators.20 For iodide detection, gold nanoparticles,21 poly(vinylpyrrolidone)-supported copper nanoclusters,22 bidentate ureido-dihomooxacalix[4]arene,23 hexahomotrioxacalix[3]arene,24 and porous ionic polymer7 based colorimetric sensors are noteworthy. Recently, we have reported dipicryl hydrazine based colorimetric sensors for selective determination of anions like fluoride, acetate, hydroxide, cyanide, and hydrogen sulfate. The anion binding ability of dipicrylhydrazine with various anions like fluoride, acetate, hydroxide, cyanide, and hydrogen sulfate is due to polar non-polar interactions and hydrogen bonding.25 Continuing our efforts, hereby we are reporting furfuryl and benzyl functionalized calix[4]amido crown-based visual molecular sensors for cyanide and iodide ions with ppm level detection limit. Limited studies pertain to the application of calix[4]amido crowns have been explored for the detection of transition metal cations26 and limited anions.27 The amide moiety of calix[4]amido crowns have a unique role in sensory behavior since N–H can interact with anions via selective hydrogen bonding donor.28–31 The furfuryl and benzyl substituent attach with amide moiety provide secondary interactions with chromophoric assistance, essential for the colorimetric sensor. These studies are unexplored in the context of cyanide and iodide. The current study also throws the light on detailed synthesis of furfuryl and benzyl functionalized calix[4]amido crowns, their stereochemistry, rigidity, steric hindrance, and interaction behavior using diverse analytical techniques such as NMR, XRD, and UV-vis.
Experimental section
Experimental materials
All reactions and manipulations were routinely performed under an inert atmosphere. Solvents such as toluene, ethanol, tetrahydrofuran, acetonitrile were purchased from Merck and purified by standard procedures, and freshly distilled before use. Reagents such as p-tert-butyl phenol and diphenyl ether, ethyl bromoacetate, chloroacetylchloride, and diethylenetriamine were purchased from Sigma-Aldrich and used as received. The furfuryl amine, benzylamine, and all tetrabutylammonium salts of anions (F−, Cl−, Br−, I−, CN−, OH−, CH3COO−, NO3−, ClO4− and HSO4−) were purchased from Alfa-Aesar. Analytical thin-layer chromatography was carried out on silica plates (SiO2,Merck 60 F254) Obtained from E. Merck Chemical Co.
Physical measurement
UV-vis spectra were recorded using a Varian model Cary Win 400 UV/Vis spectrophotometer. The Perkin Elmer LS55 fluorescence spectrophotometer was used for recording fluorescence spectra. Nuclear magnetic resonance spectra (1H NMR & 13C NMR) were recorded on a Bruker 500 MHz WB FT-NMR spectrometer having proton noise decoupling mode with a standard 5 mm probe. Chemical shifts for 1H NMR spectra are reported as δ in parts per million (ppm) downfield from SiMe4 (δ 0.0) and relative to the signal of chloroform-d (δ 7.26, singlet). Multiplicities were given as: s (singlet); d (doublet); t (triplet); q (quartet) or m (multiplets). For proving strong binding between ionophore hosts and anionic guests, 127I NMR was recorded separately at 100 MHz under same solvent mixture ratio. The melting point was checked by Buchi melting point apparatus M-565. Mass spectra were recorded by Bruker microTOF-Q II using ESI source, and elemental analysis was done on FLASH EA 1112 series from Thermo finnigan, Italy.
Synthesis and characterization of ionophores
The p-tert-butylcalix[4]aene, calix[4]arene, 1,3 diester calix[4]arene and calix[4]amido crown-5 were prepared as per the reported literature procedure.26a,32–34 N-Furfurylchloroacetamide and N-benzylchloroacetamide reagents were synthesized according to reported methods.35,36
Synthesis of ionophore I
To a solution of calix[4]amidocrown-5 (0.91 g, 1.5 mmol) in dry CH3CN (70 mL) were added N-benzyl chloroacetamide (0.83 g, 4.5 mmol) and K2CO3 (1.04 g, 7.5 mmol). The reaction mixture was refluxed for 48 h. The reaction mixture was quenched by addition of 5% HCl (10 mL) and CH2Cl2 (40 mL). The organic phase was separated and washed with water (3 × 40 mL), dried over anhydrous sodium sulphate, and evaporated in vacuo. The crude product was recrystallized with CHCl3 and methanol to yield a white crystalline compound (0.89 g, 78.95%) as shown in Scheme 1. mp 229 °C, 1H-NMR: (500 MHz, CDCl3 see ESI Fig. S1†) δ 8.82 (t, 2H, CONH), 7.80 (s, 2H, OH), 7.64 (t, 1H, NH), 7.37–7.31 (m, 5H, benzyl), 7.13 (d, 4H, ArH), 6.95 (d, 4H, ArH), 6.85 (t, 2H, ArH), 6.78 (t, 2H, ArH), 4.48 (d, 2H, benzyl), 4.40 (s, 4H, OCH2), 4.08 (d, 4H, ArCH2Ar AB system), 3.53 (br s, 4H, CONHCH2), 3.48 (d, ArCH2Ar, AB system), 3.26 (s, 2H, benzyl), 2.93 (br, s, 4H, CONHCH2CH2). 13CNMR: (see ESI Fig. S2†); δ 71.1, 168.7, 151.9, 150.9, 132.5, 129.7, 129.2, 129.1, 128.8, 128.6, 128.5, 127.9, 127.7, 127.6, 127.2, 126.9, 126.7, 120.9 (ArCH, ArC, CONH), 74.8 (OCH2), 59.9, 54.9 (benzylC), 44.19, 43.2, 39.9, 37.1, 31.9, 31.5 (CONHCH2CH2, ArCH2Ar), 30.3, 30.1, 29.7, 29.4, 27.1, 22.7, 19.8, 14.1 (benzylC). Maldi TOF MS ES+ (m/z) calculated for C45H46O7N4, 754.34, found 755.34 [M + H+]+, 756.34 [M + 2H+]+ (see ESI Fig. S14†) CHN Anal. calcd for C45H46O7N4: C 71.60, H 6.14, N 7.42, O 14.84; found: C 71.59, H 6.12, N 7.41, O 14.82.
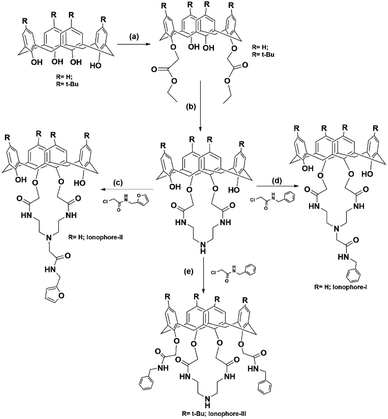 |
| Scheme 1 Showing synthetic procedure for compounds: (a) K2CO3, BrCH2COOC2H5, acetone; (b) toluene/ethanol, diethylenetriamine; (c and d) K2CO3, acetonitrile, 48 h, reflux (e) NaH, THF: DMF: 2.5 : 1, 72 h reflux. | |
Synthesis of ionophore II
To a solution of calix[4]amidocrown-5 (0.91 g, 1.5 mmol) in dry CH3CN (70 mL) were added N-furfurylchloroacetamide (0.78 g, 4.5 mmol) and K2CO3 (1.04 g, 7.5 mmol). The reaction mixture was refluxed for 48 h. The solvent was removed in vacuo and the residue was quenched by addition of 5% HCl (10 mL) and CH2Cl2 (40 mL). The organic phase was separated and washed with water (3 × 40 mL), dried over anhydrous sodium sulphate, and evaporated in vacuo. The crude product recrystallized with CHCl3 and methanol to yield a white crystalline compound (0.80 g, 72.8%) as shown in Scheme 1. mp 258 °C; 1H NMR: (500 MHz, CDCl3 see ESI Fig. S3†); δ 8.76 (t, 2H, CONH), 7.79 (s, 2H, OH), 7.54 (t, 1H, NH), 7.12 (m, 1H, furfuryl) 7.07–7.05 (d, 4H, ArH), 6.90–6.88 (d, 4H, ArH), 6.78 (t, 2H, ArH), 6.71 (t, 2H, ArH), 6.18 (s, 2H, furfuryl), 4.45 (s, 4H, OCH2), 4.39 (d, 2H, CH2 furfuryl), 4.09 (d, 4H, ArCH2Ar, AB system), 3.47 (d, 4H, ArCH2Ar, AB system), 3.42 (d, 4H, CH2), 3.18 (br s, 2H, CONHCH2), 2.82 (brs, 4H, CONHCH2CH2). 13CNMR: (see ESI Fig. S4†): δ 171.1, 168.7, 152.2, 150.9, 141.9, 132.5, 129.7, 129.2, 127.6, 126.7, 120.9 (ArCH, ArC, CONH), 110.5, 106.8 (furfuryl C), 74.9 (OCH2), 59.61, 54.92 (furfuryl C), 39.9, 36.3, 31.9, 31.5 (CONHCH2CH2, ArCH2Ar), 29.7, 22.70, 14.1 (furfuryl C). Maldi TOF MS ES+ (m/z) calculated for C43H44O8N4, 744.32, found 745.32 [M + H+]+, 746.32 [M + 2H+]+, 747.32 [M + 3H+]+ (see ESI Fig. S15†), CHN Anal. calcd for C43H44O8N4: C 69.34, H 5.95, N 7.52, O 17.18; found: C 69.30, H 5.91 N 7.41, O 17.10.
Synthesis of ionophore III
To a solution of p-tert-butyl calix[4]amido crown-5 (1.24 g, 1.5 mmol) in dry THF (50 mL) and dry DMF (20 mL) under N2 was added NaH (0.18 g, 7.5 mmol). The mixture was stirred at RT for 30 min followed by addition of N-benzyl chloroacetamide (0.83 g, 4.5 mmol). The reaction mixture was refluxed for 72 h, the solvent was removed in vacuo and quenched by the addition of 5% HCl (10 mL) and CH2Cl2 (40 mL). The organic phase was separated and washed with water (3 × 40 mL), dried over anhydrous sodium sulfate and evaporated in vacuo. The crude product recrystallized with CHCl3 and methanol to yield a white crystalline compound (1.126 g, 67.42%) as shown in Scheme 1. mp 244 °C; 1H-NMR: (500 MHz, CDCl3 see ESI Fig. S5†); δ 8.82 (t, 2H, CONH), 7.66 (t, 1H, CONH), 7.41 (s, 2H, ArH), 7.28 (m, 6H, benzyl), 7.20 (m, 4H, ArH), 7.11 (s, 4H, ArH), 6.85 (s, 4H, ArH), 4.47 (d, 2H, benzyl), 4.38 (s, 4H, OCH2), 4.04 (d, 4H, ArCH2Ar AB system), 3.51 (br s, 4H, CONHCH2), 3.45 (s, 4H, CONHCH2), 3.40–3.37 (d, ArCH2Ar, AB system), 3.26 (s, 2H, benzyl), 2.91 (br, s, 4H, CONHCH2CH2), 1.63 (1H, NH), 1.30 (s, 18H, t-butyl), 0.99 (s, 18H, t-butyl). 13CNMR: (see ESI Fig. S6†); δ 170.1, 167.9, 148.4, 147.6, 147.5, 142.53, 137.86, 130.9, 127.5, 126.5, 126.4, 126.1, 125.1, 124.6 (ArCH, ArC, CONH), 73.6 (OCH2), 58.7, 53.9 (benzylC), 42.16, 38.5, 37.1, 33.1, 32.9, (CONHCH2CH2, ArCH2Ar), 30.7, 30.5, 29.8, 29.7, 28.6, 21.8, 13.1 (benzyl C). Maldi TOF MS ES+ (m/z) calculated for C70H87O8N5, 1125.66, found 1126.66 [M + H+]+, 1127.66 [M + 2H+]+ (see ESI Fig. S16†), CHN Anal. calcd for C70H87O8N5: C 74.6, H 7.78, N 6.22, O 11.36; found: C 74.9, H 7.12, N 6.41, O 11.82.
Visual detection experiment
Visual detection experiments were carried out for recognizing the host–guest interactions of ionophores I–III with various anions. In a typical experiment, a series of stock solutions of anions (5 × 10−3 M) and ionophores (5 × 10−5 M) were prepared in freshly distilled acetonitrile. Further, 2 mL aliquots of stock solution were taken and mixed with 2 mL of the anion solution. Typical photographs were taken with a digital camera. All ionophores gave instant color change for iodide and cyanide ions. Iodide and cyanide ion gave pink and wine color for ionophore I, respectively, consistent with the color variation displayed in Fig. 1. The ionophore II showed light pink color with iodide while cyanide turned yellow. In contrast to ionophore I and II, ionophore III gives light yellow color with cyanide, but there is no visible change in the case of iodide.
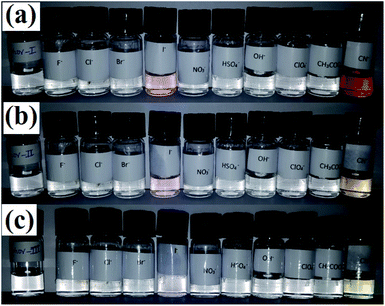 |
| Fig. 1 Colorimetric analysis for (a) ionophore I, (b) ionophore II, and (c) ionophore III upon addition of different anions. | |
Interaction with anions by UV-vis study
In UV-vis titration experiment, 1 mL (5 × 10−3 M) stock solution of tetrabutylammoniumanions (F−, Cl−, Br−, I−, CN−, OH−, CH3COO−, NO3−, ClO4− and HSO4−) was added to a 1 mL (5 × 10−5 M) acetonitrile solution of ionophore I–III in ascending order in ten factions (1
:
100 ratio). The spectral changes were recorded to ascertain the interaction/selectivity. Based on spectral changes, titrations were performed with strongly interacted anions cyanide and iodide to investigate the binding sites and determine the binding constant. We have performed competitive binding with other anions (F−, Cl−, Br−, I−, CN−, OH−, CH3COO−, NO3−, ClO4− and HSO4−) and found that ionophores are showing selective binding and color change towards cyanide.
NMR spectral study
The 1H and 13C NMR spectra of ionophore I–III were recorded before and after the addition of selected anions (CN− and I−) in CDCl3
:
CD3CN (1
:
2.5). In this experiment, 0.003 mmol of ionophore I–III were dissolved separately in 0.5 mL mixture containing CDCl3
:
CD3CN (1
:
2.5), and further, add various anions (20 molar equivalents) in the form of TBA salts. Proton NMR was recorded at different time intervals up to 24 h after the addition of anions.
Structure determination by single-crystal X-ray study
Appropriate crystal was selected for ionophore I, dipped in paratone oil, and mounted on cryo loop. Crystal data were collected at 100 K using graphite monochromatic MoKα (λ = 0.71073 Å) radiation on a Bruker SMART APEX diffractometer equipped CCD area detector. The SAINT software37 was used for data integration and reduction. Empirical absorption correction was applied to the collected reflections with SADABS.38 SHELXTL39 was used to solve the structures by direct methods using and refined on F2 by the full-matrix least-squares technique using the SHELXL-97 (ref. 40) package. Non-hydrogen atoms were refined anisotropically till convergence is reached. The MERCURY 3.8 (ref. 41) is used to generate graphics. The X-ray structure of ionophore I is shown in Fig. S17,† wherein chloroform and water molecules have been omitted for clarity. The phenolic OH of the alternate phenyl rings of the calix moiety are substituted by amide-linked chains. The presence of hydrogen bonding between amide NHs and phenolic oxygen atoms is also observed. Crystallographic parameters for the ionophore I are given in Table S1.†
Result and discussion
UV-vis absorption studies
Quantitative investigations were carried out to understand cyanide and iodide anions' encapsulation behavior, in presence of different ionophores using a spectrophotometric titration method in CH3CN. The absorption spectra of ionophores I, II, and III at variable concentrations of iodide and cyanide are shown in Fig. 2 and 3. Ionophores I and II show a strong absorption maximum at 279 nm, while III shows at 285 nm. Single spectral band for anions and dual spectral bands were observed for all ionophores from 273 to 290 nm. Upon increasing the anions' concentration, the absorbance of ionophores is increased at both the maxima as per Fig. 2a–c (for iodide) and Fig. 3a–c (for cyanide). In the absence of ionophore, iodide and cyanide anions show their respective peaks at 249 and 275 nm, respectively. The absorbance increased with the concentration of anions due to interaction with ionophores. Changes in the absorbance of ionophores (measured against the solvent as reference) upon the addition of anions were observed at 279 nm for ionophore's I and II, and 285 nm for ionophore III. Owing to their hydrophobic cavities, host ionophores offer the iodide and cyanide a suitable environment for interaction to form inclusion complexes. The binding constants for the formation of ionophores: iodide and ionophores: cyanide complexes are determined by analyzing the absorbance changes. The binding constant Ka and stoichiometry of the inclusion complexes of ionophores with iodide and cyanide can be determined by the Benesi–Hildebrand equation (Table 1).42 For ionophores I, II and III, the calculated binding constant from the straight-line slope for iodide is found to be 552 M−1, 509 M−1, and 350 M−1, respectively. At the same time, for cyanide, the values are lower, i.e., 319 M−1, 304 M−1 and 209 M−1, respectively, at 298 K. This behavior explains strong complexation towards both anions in the following order: I > II > III. It is observed that ionophore I gave a stronger Ka value than II and III, indicating its superior interaction compared to heavily alkylated and arylated II and III. Considering the structural features of the host and guest, we presumed that the deprotonation and charge transfer via polarization leads to complexation with iodide. In contrast, hydrogen bonding between cyanide and amines of ionophore leads to deprotonation and strengthens the host–guest association. All the above phenomena are common in all ionophores. Since steric hindrances are in the following order: III > II > I, bind in reverse order.
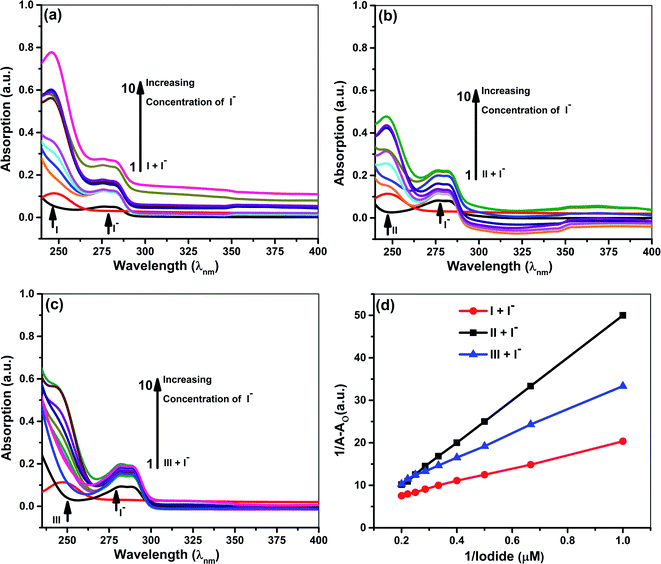 |
| Fig. 2 Absorption spectra of 5 × 10−5 M ionophores in the absence and presence of iodide at concentrations 5 × 10−5 to 5 × 10−6 M (a) ionophore I (b) ionophore II (c) ionophore III (d) Benesi–Hildebrand absorption plot for 1 : 1 complexation of iodide with ionophores I–III. | |
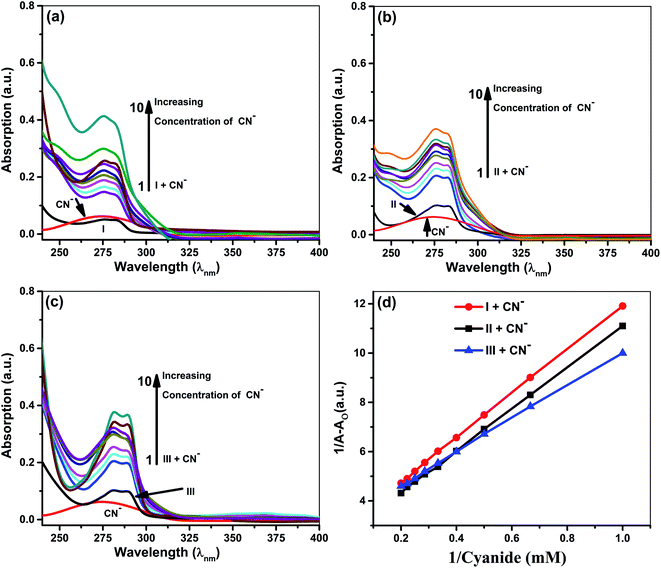 |
| Fig. 3 Absorption spectra of 5 × 10−5 M ionophore's in the absence and presence of cyanide at concentrations 5 × 10−3 to 5 × 10−4 M (a) ionophore I (b) ionophore II (c). Ionophore III (d) Benesi–Hildebrand absorption plot for 1 : 1 complexation of cyanide with ionophores I–III. | |
Table 1 Binding constants of the complexes via absorption spectral maxima for iodide and cyanide anions with Ionophores I, II and III
S. No. |
Ionophores |
λ (nm) |
Monitored λmax (nm) |
Binding constant Ka (M−1) |
R2 |
Complex |
For iodide anion |
1 |
I |
273–284 |
279 |
552.0 |
0.9988 |
1 : 1 |
2 |
II |
273–284 |
279 |
509.0 |
0.9999 |
1 : 1 |
3 |
III |
280–290 |
285 |
350.0 |
0.9982 |
1 : 1 |
![[thin space (1/6-em)]](https://www.rsc.org/images/entities/char_2009.gif) |
For cyanide anion |
4 |
I |
273–284 |
279 |
319.0 |
0.9996 |
1 : 1 |
5 |
II |
273–284 |
279 |
304.0 |
0.9996 |
1 : 1 |
6 |
III |
280–290 |
285 |
209.0 |
0.9993 |
1 : 1 |
The plot's good linearity with better correlation co-efficient R2 more than 0.998 for all complexes shows the strong formation of 1
:
1 complexes between anions and ionophores I, II, and III. Stoichiometric ratios are calculated from Job's Plot, which indicates towards 1
:
1 complex formation (see ESI Fig. S7†).43
Fluorescence studies
Samples prepared for UV-vis analysis were further used for the spectrofluorometric study. Table 2 and Fig. 4 & 5 show the supramolecular interaction of ionophores with iodide and cyanide anions. An enrichment of fluorescence intensity of ionophores was observed upon adding iodide and cyanide aliquots. Such qualitative assessment of the inclusion complexation behavior by spectral titrations shows a single emission maximum at 379 nm for ionophore I and II and 380 for ionophore III, respectively, with very low fluorescence intensity before the addition of anions at excitation wavelength of 279 nm for I and II and 285 nm for ionophore III. In the case of iodide anion, there is no spectral shift observed in ionophore II and III, while ionophore I shows the blue shift to 375 nm (∼5 nm). Fig. 4 and 5 shows the emission spectra of iodide and cyanide anion with all ionophores.
Table 2 Binding constants of the complexes via emission spectral maxima for iodide and cyanide anions with ionophores I, II and III
S. No. |
Ionophores |
λ (nm) |
Monitored λmax (nm) |
Binding constant Ka (M−1) |
R2 |
Complex |
For iodide anion |
1 |
I |
375–385 |
379 |
834 |
0.9997 |
1 : 1 |
2 |
II |
375–385 |
379 |
729 |
0.9995 |
1 : 1 |
3 |
III |
380–384 |
380 |
498 |
0.9990 |
1 : 1 |
![[thin space (1/6-em)]](https://www.rsc.org/images/entities/char_2009.gif) |
For cyanide anion |
4 |
I |
375–385 |
379 |
956 |
0.9994 |
1 : 1 |
5 |
II |
375–385 |
379 |
753 |
0.9998 |
1 : 1 |
6 |
III |
380–384 |
380 |
604 |
0.9991 |
1 : 1 |
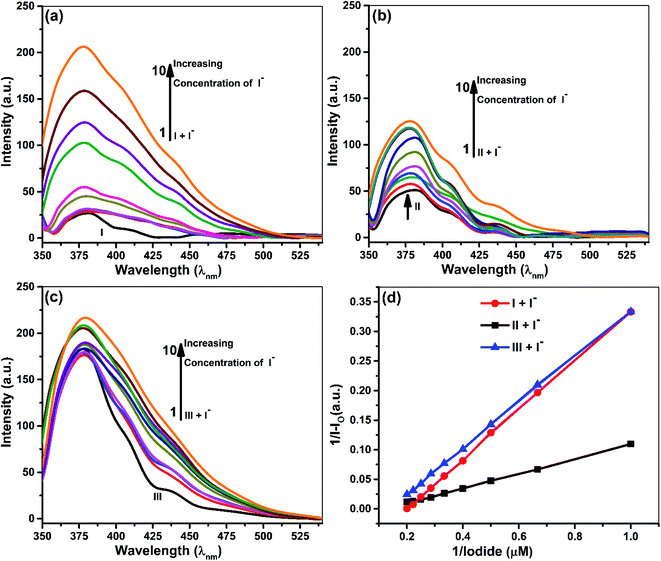 |
| Fig. 4 Fluorescence spectra of 5 × 10−5 M ionophores in the absence and presence of iodide at concentrations 5 × 10−5 M to 5 × 10−6 M. (a) Ionophore I (b) ionophore II (c) ionophore III (d) Benesi–Hildebrand emission plot for 1 : 1 complexation of iodide with ionophores I–III. | |
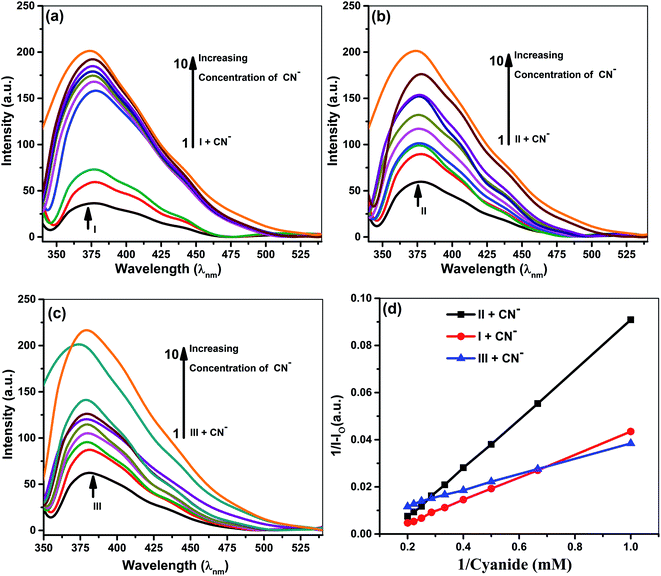 |
| Fig. 5 Fluorescence spectra of 5 × 10−5 M ionophores in the absence and presence of cyanide at concentrations 5 × 10−3 M to 5 × 10−4 M. (a) Ionophore I (b) ionophore II (c) ionophore III (d) Benesi–Hildebrand absorption plot for 1 : 1 complexation of cyanide with ionophores. | |
Enhancement of emission maxima shows gradual redshift for I and II from 379 nm to 375 nm, while no noticeable changes were observed for ionophore III. Alteration in the host molecule's photophysical and photochemical properties establishes transference of the anions from a more protic atmosphere to a less protic atmosphere, i.e., the cavity of ionophores. The ionophores can form intra and inter-molecular hydrogen bonds between the phenolic O–H and the nitrogen of the cyanide. The supramolecular cavity of ionophores offers a protective environment with excitation of singlet species and vibrational restriction to iodide and cyanide molecules during encapsulation. The study clearly explained that the encapsulation or inclusion phenomenon depends on the fitting and selective detection concept between host and guest molecules. Such encapsulations cannot be clearly explained on single parameter, various spectacles leads to several weak intermolecular forces such as ion–dipole, dipole–dipole, van der Waals, electrostatic forces, hydrogen bonding strengths, and steric effects. Not only this, hydrogen-bonding donor binding to an anions involves deprotonation of hydrogen binding donor, in which proton transferred to basic anions and it varies with size and electronegative character of anions CN− and I−.
All the above concepts collaborate and contribute to the formation of a stable inclusion. Hence, inclusion formation constants or binding constants (Ka) of all the complexes were calculated from fluorescence data using the Benesi–Hildebrand equation, and the stoichiometric ratio was determined (Table 2).42,43 A good linearity with a better regression co-efficient, R2 is obtained. The binding constant ‘Ka’ is calculated from the straight line's slope, considering both ground and excited state measurements. It was found to be 834 M−1, 729 M−1 & 498 M−1 in the case of iodide anion, while the derived binding constant from cyanide complexes were 956 M−1, 753 M−1 & 604 M−1 for ionophore I, II & III, respectively. The above behavior indicates that all ionophore forms different inclusion complexes with cyanide and iodide ions. The linearity of the plot shows that the stoichiometry of the complex between ionophores and both the anions was found to be 1
:
1. The binding constant values for ionophore I with all anions are higher than other complexes (Table 2), indicating that ionophore's capability to form inclusion complexes with iodide and cyanide anions. The ionophore I can complex better with the cyanide and iodide than arylated II and III. For iodide, deprotonation and charge transfer via polarization are major interacting forces. However, hydrogen bonding between nitrogen atoms of cyanide and amines of ionophore leads to complete deprotonation and strengthening host–guest association. All above phenomena were common in all ionophores; however, steric hindrances are highest in III, which have the least binding. Stoichiometric ratios were calculated from Job's plot that shows 1
:
1 complex formation (see ESI Fig. S7†).43 Binding constant data's for emission and absorption data's were different, but dual binding constants and better R2 values in same sequence clearly explain that ionophores have a strong binding capability with anions.
1H & 127I NMR study
The inclusion of guest ions inside the ionophore's cavity was ascertained by changes observed in 1H & 127I NMR. The NMR interaction studies were carried out by adding the equimolar concentration of ionophore I and anions at 297 K. For iodide anion there is slight significant change was seen in time-dependent 1HNMR (Fig. 6) due to the large size and poor hydrogen bonding tendency. Peak a get diminished with slight shift in case of peak c and becomes constant after 6 h. Interestingly, the 127I showed 27.6 ppm upfield shift due to inclusion and non-covalent interaction inside the cavity (Fig. 7). No further changes were observed after 6 h. The other two ionophores II and III, also showed similar trends (see ESI Fig. S8–S13†).
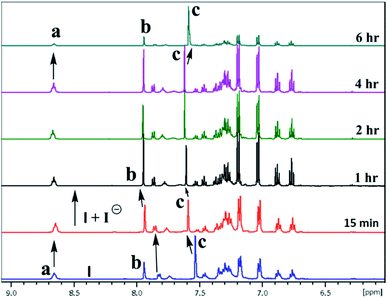 |
| Fig. 6 A part of time-dependent 1H NMR spectra (500 MHz) in CDCl3: CD3CN at 25 °C showing protons of ionophore I in the absence presence of iodide anion. | |
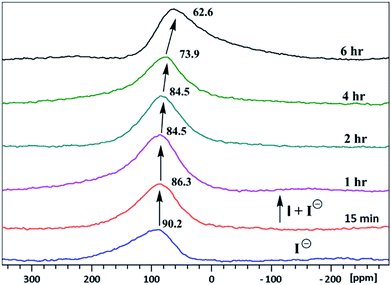 |
| Fig. 7 Time-dependent 127I NMR spectra (500 MHz) in CDCl3: CD3CN at 25 °C of tetrabutylammonium iodide with ionophore I. | |
In the case of cyanide, clear changes were observed in time-dependent proton NMR with deprotonation of peak 8.45 ppm (–NH) and 7.75 ppm (–OH) of ionophores within 15 min, as shown in Fig. 8. For ionophore I, aromatic peaks shifted downfield due to the strong nucleophilicity of cyanide ion inclusion. It has been observed that ionophores do not behave chemidosmetrically as the color started fading after 72 h and the N–H at 8.45 ppm proton started reappearing (Fig. S18†). The sterically crowded ionophore III showed the least binding capability as observed in 127I NMR shift (see ESI Fig. S13†).7b
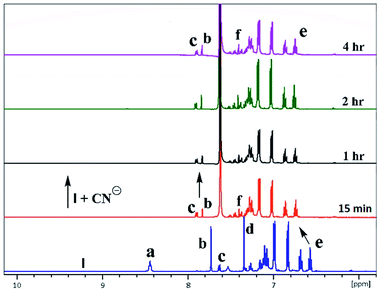 |
| Fig. 8 A part of time-dependent 1H NMR spectra (500 MHz) in CDCl3: CD3CN at 25 °C for tetrabutylammonium cyanide anion with ionophore I. | |
Conclusions
Three new functionalized furfuryl and benzyl derivated of calix[4]amido crown based molecular ionophores were synthesized to investigate their anion sensing properties. These ionophores were designed with variation in lower rim conformation based on rigidity and steric hindrance at the binding site. A number of anions were investigated with three ionophores indicating that ionophore I bind strongly with both iodide and cyanide ions with interaction order I > II > III. The ionophore I shows drastic color change from colorless to pink and wine red for iodide and cyanide anion. For iodide ion, ionophore II and III do not show very sharp color change while CN− gave colorless to light yellow. The strong binding for iodide ion was evident by 127I NMR where ionophore I gave 27.6 ppm upfield shift while for ionophore II and III, 13.9 and 10 ppm, respectively. The cyanide ion shows strong interaction with N–H and O–H groups, with all three ionophores, resulted in a color change. The color change is attributed to the charge transfer process among the anions and lower amide pendulum. The ionophore I interact strongly with iodide and cyanide because of the less hindered binding site, for effective interaction with anions. The binding isotherms were fitted to a 1
:
1 binding model with better binding constants, as suggested by the Benesi–Hildebrand plot for all ionophores.
Conflicts of interest
There are no conflicts to declare.
Acknowledgements
The authors like to thank the Indian Institute of Technology Jodhpur for the infrastructure and CASE facilities. PRS gratefully acknowledges DST Women Scientists Scheme-A (WOS-A) for the research grant SR/WOS-A/CS-117/2018.
Notes and references
- I. A. Rather, S. A. Wagay and R. Ali, Emergence of anion-p interactions: The land of opportunity in supramolecular chemistry and beyond, Coord. Chem. Rev., 2020, 415, 213327 CrossRef CAS.
- R. Ali, R. C. Gupta, S. K. Dwivedi and A. Misra, Excited state proton transfer (ESIPT) based molecular probe to sense F− and CN− anions through a fluorescence “turn-on” response, New J. Chem., 2018, 42, 11746–11754 RSC.
-
(a) C. Young, L. Tidwell and C. Anderson, Cyanide: Social Industrial and Economic Aspects, Minerals, Metals, and Materials Society, Warrendale, 2001 Search PubMed;
(b) Z. Xu, X. Chen, H. N. Kim and J. Yoon, Sensors for the optical detection of cyanide ion, Chem. Soc. Rev., 2010, 39, 127–137 RSC.
- Guidelines for Drinking-Water Quality, World Health Organization, 20 Geneva, 1996 Search PubMed.
- F. P. Simeonova, L. Fishbein, Concise International Chemical Assessment Document 61, Hydrogen cyanide and cyanides: human health aspects, World Health Organization, Geneva, 2004, https://apps.who.int/iris/handle/10665/42942 Search PubMed.
-
(a) H. ling and D. Yue, BMC Pediatr., 2020, 20(169), 1–4 Search PubMed , report on Flashimato and Graves;
(b) P. Saxena, G. C. El Hamri, M. Folcher, H. Zulewski and M. Fussenegger, Synthetic gene network restoring endogenous pituitary–thyroid feedback control in experimental Graves' disease, Proc. Natl. Acad. Sci. U. S. A., 2016, 113, 1244–1249 CrossRef CAS.
-
(a) Z. Chen, R. Sun, S. Feng, D. Wang and H. Liu, Porosity-Induced Selective Sensing of Iodide in Aqueous Solution by a Fluorescent Imidazolium-Based Ionic Porous Framework, ACS Appl. Mater. Interfaces, 2020, 12, 11104–11114 CrossRef;
(b) P. R. Sharma, S. Pandey, V. K. Soni, G. Choudhary and R. K. Sharma, Macroscopic recognition of iodide by polymer appended calix[4]amidocrown resin, Supramol. Chem., 2019, 31, 634–644 CrossRef CAS.
-
(a) Q. Lin, K. P. Zhong, J. H. Zhu, L. Ding, J. X. Su, H. Yao, T. B. Wei and Y. M. Zhang, Iodine controlled pillar [5] arene-based multiresponsive supramolecular polymer for fluorescence detection of cyanide, mercury, and cysteine, Macromolecules, 2017, 50, 7863–7871 CrossRef CAS;
(b) Y. Y. Chen, X. M. Jiang, G. F. Gong, H. Yao, Y. M. Zhang, T. B. Wei and Q. Lin, Pillararene-based AIEgens: research progress and appealing applications, Chem. Commun., 2021, 57, 284–301 RSC.
- W. J. Qu, W. T. Li, H. L. Zhang, T. B. Wei, Q. Lin, H. Yao and Y. M. Zhang, A rational designed fluorescent and colorimetric dual-channel sensor for cyanide anion based on the PET effect in aqueous medium, Sens. Actuators, B, 2017, 241, 430–437 CrossRef CAS.
-
(a) H. H. Yang, P. P. Liu, J. P. Hu, H. Fang, Q. Lin, Y. Hong, Y. M. Zhang, W. J. Qu and T. B. Wei, A fluorescent supramolecular gel and its application in the ultrasensitive detection of CN− by anion–π interactions, Soft Matter, 2020, 16, 9876–9881 RSC;
(b) W. J. Qu, H. H. Yang, J. P. Hu, P. Qin, X. X. Zhao, Q. Lin, H. Yao, Y. M. Zhang and T. B. Wei, A novel bis-acylhydrazone supramolecular gel and its application in ultrasensitive detection of CN−, Dyes Pigm., 2021, 186, 108949 CrossRef CAS.
- S. D. Padghan, C. Y. Wang, W. C. Liu, S. S. Sun, K. M. Liu and K. Y. Chen, A naphthalene-based colorimetric and fluorometric dual-channel chemodosimeter for sensing cyanide in a wide pH range, Dyes Pigm., 2020, 183, 108724 CrossRef CAS.
- R. Rajamanikandan and M. Ilanchelian, β-Cyclodextrin protected gold nanoparticle based cotton swabs as an effective candidate for specific sensing of trace levels of cyanide, Anal. Methods, 2019, 11, 97–104 RSC.
- Y. M. Hijji, A. G. Elsafy, H. S. Al-Easa, B. Attili, M. Abdelrasoul, N. Mohamed and G. K. Nasrallah, Curcumin a Colorimetric and Fluorimetric Cyanide Probe in Aqueous System and Living Cells, Anal. Methods, 2019, 11, 5169–5176 RSC.
- K. Rezaeian, H. Khan mohammadi and A. Talebbaigy, Detection of CN−, Cu2+ and Zn2+ ions using a new chromone-based colorimetric chemosensor: half-adder and integrated circuits, Anal. Methods, 2020, 12, 1759–1766 RSC.
- R. Kumar, V. Bhalla and M. Kumar, Cu2+ and CN− selective fluorogenic sensors based on pyrene-appended thiacalix[4]arenes, Tetrahedron, 2008, 64, 8095–8101 CrossRef CAS.
- M. Shahid, H. M. Chawla and P. Bhatia, A calix[4]arene based turn off/turn on molecular receptor for Cu2+ and CN− ions in aqueous medium, Sens. Actuators, B, 2016, 237, 470–478 CrossRef CAS.
- B. Sathish Kumar, N. N. Pati, K. V. J. Jose and P. K. Panda, Synthetic access to Calix[3]pyrroles via meso-Expansion: Hosts with diverse Guest Chemistry, Chem. Commun., 2020, 56, 5637–5640 RSC.
- P. S. Kumar, S. Ciattini, C. Laura and K. P. Elango, Fluorescent detection of Al(III) and CN− in solid and aqueous phases and their recognition in biological samples, J. Mol. Liq., 2020, 317, 113970 CrossRef CAS.
- M. R. F. Ashworth, A Dual Colorimetric Chemosensor for Hg(II) and Cyanide ions in Aqueous Media based on Nitrobenzoxadiazole (NBD)-Antipyrine Conjugate with INHIBIT logic gate behavior, Chem. Sulphonic Acids, Esters Their Deriv., 2006, 323–350 Search PubMed.
- S. Sharma, M. Kumari and N. Singh, C3-Symmetrical Tripodal Acylhydrazone Organogelator for the Selective Recognition of Cyanide ions in Gel and Solution phase: Practical Applications in Food Samples, Soft Matter, 2020, 16, 6532–6538 RSC.
- L. Chen, W. Lu, X. Wang and L. Chen, A highly selective and sensitive colorimetric sensor for iodide detection based on anti-aggregation of gold nanoparticles, Sens. Actuators, B, 2013, 182, 482–488 CrossRef CAS.
- F. Pena-Pereira, N. Capón, I. de la Calle, I. Lavilla and C. Bendicho, Fluorescent poly(vinylpyrrolidone)-supported copper nanoclusters in miniaturized analytical systems for iodine sensing, Sens. Actuators, B, 2019, 299, 126979 CrossRef CAS.
- M. Singh, N. Singh, J. R. Ascenso and P. M. Marcos, A Dihomooxacalix[4]arene-gold nanohybrid based colorimetric sensor for sensitive and selective detection of iodide, Supramol. Chem., 2019, 31, 313–321 CrossRef.
- H. F. Xie, C. Wu, J. Zou, Y. X. Yang, H. Xu, Q. L. Zhang, C. Redshaw and T. Yamato, A pyrenyl-appended C3v-symmetric hexahomotrioxacalix[3]arene for selective fluorescence sensing of iodide, Dyes Pigm., 2020, 178, 108340 CrossRef CAS.
- P. R. Sharma, V. K. Soni, S. Pandey, G. Choudhary, A. K. Plappally and R. K. Sharma, Dipicrylhydrazine: A versatile visual anions sensor, J. Environ. Chem. Eng., 2017, 5, 2232–2239 CrossRef CAS.
-
(a) H. M. Chawla, S. P. Singh and S. Upreti, Synthesis of calix[4]arene(amido)monocrowns and their photoresponsive derivatives, Tetrahedron, 2006, 62, 9758–9768 CrossRef CAS;
(b) V. Saravanan, A. Kannan and P. Rajakumar, p-tert-Butylcalix[4]arene core based ferrocenyl dendrimers: Novel sensor for toxic Hg2+ ion even in presence of Zn2+, Cu2+ and Ag+ ions, Sens. Actuators, B, 2017, 242, 904–911 CrossRef CAS;
(c) J. Xu, Y. Yang, H. Baigude and H. Zhao, New ferrocene–triazole derivatives for multi signaling detection of Cu2+ in aqueous medium and their antibacterial activity, Spectrochim. Acta, Part A, 2020, 229, 117880 CrossRef CAS;
(d) M. Ramachandran, S. Anandan and M. Ashok kumar, A luminescent on–off probe based calix[4]arene linked through triazole with ruthenium(II) polypyridine complexes to sense copper(II) and sulfide ions, New J. Chem., 2019, 43, 9832–9842 RSC;
(e) B. Mohan and H. K. Sharma, Synthesis of calix[6]arene and transduction of its furfural derivative as sensor for Hg(II) ions, Inorg. Chim. Acta, 2019, 486, 63–68 CrossRef CAS;
(f) Y. Z. Chen, D. Pan, B. Chen, G. X. Wang, C. H. Tung and L. Z. Wu, Synthesis, Characterization, and Selective Sr2+ Sensing Study of Copper(I)-Bridged Calix[4]arene-Based Binuclear Alkynylplatinum(II) Complexes, Eur. J. Inorg. Chem., 2017, 44, 5108–5113 CrossRef.
-
(a) D. Maity, M. Bhatt, M. L. Desai, E. Suresh, M. K. Si, V. P. Boricha, B. Ganguly and P. Paul, Effect of conformation, flexibility and intramolecular interaction on ion selectivity of calix[4]arene-based anion sensors: experimental and computational studies, Supramol. Chem., 2017, 29, 600–615 CrossRef CAS;
(b) A. O. Ortolan, I. Østrøm, G. F. Caramori, R. L. T. Parreira, E. H. Da Silva and F. M. Bickelhaupt, Tuning heterocalixarenes to improve their anion recognition: a computational approach, J. Phys. Chem. A, 2018, 122, 3328–3336 CrossRef CAS;
(c) F. K. W. Hau, H. S. Lo and V. W. W. Yam, Synthesis and Photophysical Studies of Calixarene-Based Alkynylplatinum(II) Terpyridine Complexes with Various Receptor Sites for Colorimetric and Luminescence Sensing of Anions, Chem.–Eur. J., 2016, 22, 3738–3749 CrossRef CAS;
(d) C. Parthiban, S. Ciattini, L. Chelazzi and K. P. Elango, Selective colorimetric sensing of fluoride in an aqueous solution by amino-naphthoquinone and its Co(II), Ni(II), Cu(II) and Zn(II) complexes – effect of complex formation on sensing behavior, RSC Adv., 2016, 6, 91265–91274 RSC.
- H. M. Chawla, S. N. Sahu and R. Shrivastava, Synthesis and binding characteristics of novel calix [4] arene (amidocrown) diquinones, Can. J. Chem., 2009, 87, 523–531 CrossRef CAS.
-
(a) S. B. Nimse and T. Kim, Biological applications of functionalized calixarenes, Chem. Soc. Rev., 2013, 42, 366–386 RSC;
(b) C. Chen, X. Ni, H. W. Tian, Q. Liu, D. S. Guo and D. Ding, Calixarene-Based Supramolecular AIE Dots with Highly Inhibited Nonradiative Decay and Intersystem Crossing for Ultrasensitive Fluorescence Image-Guided Cancer Surgery, Angew. Chem., Int. Ed., 2020, 59, 10008–10012 CrossRef CAS PubMed;
(c) D. Xia, P. Wang, X. Ji, N. M. Khashab, J. L. Sessler and F. Huang, Functional Supramolecular Polymeric Networks: The Marriage of Covalent Polymers and Macrocycle-Based Host−Guest Interactions, Chem. Rev., 2020, 120, 6070–6123 CrossRef CAS.
- G. Yu, K. Jie and F. Huang, Supramolecular Amphiphiles Based on Host−Guest Molecular Recognition Motifs, Chem. Rev., 2015, 115, 7240–7303 CrossRef CAS PubMed.
- S. Patra, D. Maity, R. Gunupuru, P. Agnihotri and P. Paul, Calixarenes: Versatile molecules as molecular sensors for ion recognition study, J. Chem. Sci., 2012, 124, 1287–1299 CrossRef CAS.
- C. D. Gutsche and M. Iqbal, p-tert-butylcalix[4]arene, Org. Synth., 1993, VIII, 75–76 Search PubMed.
- C. D. Gutsche, J. A. Levine and P. K. Sujeeth, Calixarenes. 17. Functionalized Calixarenes: The Claisen Rearrangement Route, J. Org. Chem., 1985, 50, 5802–5806 CrossRef CAS.
- F. Unob, Z. Asfari and J. Vicens, An Anthracene-Based Fluorescent Sensor for Transition Metal Ions Derived From Calix[4]arene, Tetrahedron Lett., 1998, 39, 2951–2954 CrossRef CAS.
- M. Bayrakcl and S. Yigiter, Synthesis of tetra-substituted calix[4]arene ionophores and the irrecognition studies toward toxic arsenate anions, Tetrahedron, 2013, 69, 3218–3224 CrossRef.
- Z. Q. Shi, Y. Q. Feng, N. Song and H. W. Wang, Novel Synthesis and Characterization of N-Substituted-calix[4]azacrown Derivatives, Synth. Commun., 2008, 38, 983–990 CrossRef CAS.
- G. M. Sheldrick, Saint, 5.1 edn, Siemens Industrial Automation Inc., Madison, WI, 1995 Search PubMed.
- SADABS, Empirical
Absorption Correction Program, University of Göttingen, Göttingen, 1997 Search PubMed.
- G. M. Sheldrick, SHELXTL Reference Manual: Version 5.1, Bruker AXS, Madison, WI, 1997 Search PubMed.
- G. M. Sheldrick, SHELXL-97: Program for Crystal Structure Refinement, University of Göttingen, Göttingen, 1997 Search PubMed.
- Mercury 1.3, Supplied with Cambridge Structural Database, CCDC, Cambridge, 2003 Search PubMed.
- I. D. Kuntz, F. P. Gasparro, M. D. Johnston and R. P. Taylor, Molecular Interactions and the Benesi–Hildebrand Equation, J. Am. Chem. Soc., 1968, 90, 4778–4781 CrossRef CAS.
- D. B. Hibbert and P. Thordarson, The death of the Job plot, transparency, open science and online tools, uncertainty estimation methods and other developments in supramolecular chemistry data analysis, Chem. Commun., 2016, 52, 12792–12805 RSC.
Footnote |
† Electronic supplementary information (ESI) available. CCDC 1506051. For ESI and crystallographic data in CIF or other electronic format see DOI: 10.1039/d1ra03608d |
|
This journal is © The Royal Society of Chemistry 2021 |