DOI:
10.1039/D1RA02910J
(Paper)
RSC Adv., 2021,
11, 19333-19350
PEI functionalized NaCeF4:Tb3+/Eu3+ for photoluminescence sensing of heavy metal ions and explosive aromatic nitro compounds†
Received
14th April 2021
, Accepted 12th May 2021
First published on 27th May 2021
Abstract
This work reports an eco-friendly hydrothermal approach for the synthesis of hexagonal NaCeF4:Tb3+/Eu3+ nanophosphors. The phase, morphology and optical properties were characterized by Powder X-ray diffraction (PXRD), field emission scanning electron microscopy (FE-SEM), transmission electron microscopy (TEM), Fourier transform infrared (FT-IR) spectroscopy and photoluminescence (PL) spectroscopy respectively. Herein, the as-synthesized nanophosphor was functionalized with amine rich polyethylenimine (PEI) resulting in development of a luminescent nanoprobe bearing dual sensing functions for hazardous nitroaromatics and heavy metal ions. The strong photoluminescence emission of Eu3+ ions was selectively quenched upon addition of toxic analytes at concentrations from 10 to 100 ppm due to complex formation between the analytes and PEI functionalized nanostructure. The synthesized nanomaterial shows sharp emission peaks at 493, 594, 624, 657 and 700 nm. Significantly, the peak at 594 nm shows a noticeable quenching effect on addition of toxic analytes to the aqueous solution of the nanocrystals. The nanophosphors are sensitive and efficient for the PA and Fe3+ ion detection with an LOD of 1.32 ppm and 1.39 ppm. The Stern–Volmer (SV) quenching constant (KSV) is found to be 2.25 × 105 M−1 for PA and 3.8 × 104 M−1 for Fe3+ ions. The high KSV value and low LOD suggest high selectivity and sensitivity of the nanosensor towards PA and Fe3+ ions over other analytes. Additionally, a reduced graphene oxide and nanophosphor based nanocomposite was also synthesized to investigate the role of energy transfer involving delocalized energy levels of reduced graphene oxide in regulating the luminescence properties of the nanophosphor. It was observed that PEI plays central role in inhibiting the quenching effect of reduced graphene oxide on the nanophosphor.
Introduction
Lanthanide doped luminescent nanomaterials have gained immense attention due to their potential applications in various fields such as drug delivery,1 catalysis,2 optoelectronics3,4 and chemical sensing.5 These nanocrystals are widely used for the identification of hazardous heavy metal ions (Fe, Cr, Co, Cd) and explosive aromatic nitro compounds (p-nitro aniline, picric acid, p-nitrophenol, 4-nitrotoulene).6–8 The toxic chemical compounds are heavily spread over surface of soil via various anthropogenic activities like machinery and electronic manufacturing, fossil fuel, oil refining and electroplating.9,10 The discharge of toxic chemical compounds into water disturb the whole ecosystem and has become a challenge worldwide. The pollution caused by chemical compounds originates from rapid industrial development and urbanization.11 The release of the hazardous compounds into the environment has increased drastically in underdeveloped countries and may cause massive affect to all living creatures.12,13 These chemicals can exert harmful effect on respiratory system, nervous system, connective – epithelial tissue, irritation to the eyes, inflammation in nose and skin.14 The non-biodegradable nature of many chemical compounds in the environment deteriorates the situation than ever before.15 A multipronged strategy is needed to tackle theses deleterious compounds. Researchers all around the world have employed various analytical techniques for the detection of heavy metal ions and aromatic nitro compounds like inductively coupled plasma mass spectrometry (ICPMS),16 atomic absorption spectroscopy (AAS),17 gas chromatography coupled with electron capture detection,18 ion mobility spectroscopy (IMS),19 Raman spectroscopy20 and high-pressure liquid chromatography (HPLC).21 However, these techniques are expensive and time-consuming since they depend on sophisticated equipment. These factors limit fast and convenient detection.
The metal ion (Fe3+) is one of the most significant metal ions that play an important role in biological systems.22 The Fe3+ metal ion participates in many biological processes such as RNA and DNA synthesis and helps in the oxygen transport capacity of haemoglobin. Deficiency and excess of this metal ion can cause serious disorders and diseases in human beings. The deficiency of Fe3+ ions can lead to anaemia in the body, whereas its excess can cause life-threatening conditions, such as heart problems, liver and pancreas disease and diabetes.23 Picric acid (PA) is also an essential chemical source used as an antiseptic agent for the ministration of burns, small pox, and malaria.24 The PA continues to accumulate in the atmosphere as a highly toxic and poorly degradable compound, which poses pernicious influence to both the human (central nervous system and cardiovascular systems) and the environment.25 Therefore, the detection of these toxic analytes becomes crucial for monitoring human health and the environment.
Lanthanide based photoluminescent nanocrystals have been proposed as potential material as new class of luminescent probes.26 They exhibit exceptional chemical and optical properties particularly sharp emission peaks with large Stokes shift and longer lifetimes due to forbidden nature of intra 4f–4f transitions.27–29 Moreover, the lanthanide-doped nanostructures shows a distinct set of sharp emission peaks independent of particle size compared to that of single and broad emission peaks observed for quantum dots or other nanoparticles.30 Furthermore, the emission peak position is independent of the excitation wavelength.31 The lanthanide-doped host matrix such as fluorides,32 oxides,33 phosphates34 and oxysulfides35 are thermally and photo-chemically stable.36 The fluorides are appropriate among the hosts compared to other host matrices. This is due to their low phonon energies that can quickly reduce non-radiative relaxations, resulting in higher photoluminescence quantum yields.37,38
Malik and co-workers developed PEI functionalized NaYF4:Gd3+/Tb3+ for selective detection of 2,4,6-trinitrotoluene via photoluminescent sensing technique. The PEI helps in detection of the hazardous nitroaromatic compound by the formation of the Meisenheimer complex and quenching the photoluminescence.39 Tashi et al. fabricated down converting serine functionalized NaGdF4:Eu3+/Ce3+@NaGdF4:Tb3+ core–shell nanomaterial for the selective and sensitive detection of picric acid.40 Abbas and co-workers developed C-encapsulated three-dimensional Cu nano-pyramids (C@3DCu-PY) sensors for the control and monitoring of phenolic compounds.41 Saleemi et al. reported a novel OVs rich spinel ZnFe2O4 micro-spheres (ZF-Ms) sensor for the effective detection of As3+ metal ion present in underground water.42 Abbas et al. fabricated a novel Cu nanoplates functionalized carbon nanoparticles (Cu@CNP) for the selective and sensitive detection of dopamine.43
Herein, we have synthesized PEI functionalized NaCeF4:Tb3+/Eu3+ (see Scheme 1) for the selective detection of picric acid and Fe3+ metal ion in the aqueous solution. The presence of PEI residues on the surface of synthesized nanocrystals hinders aggregation and increases water solubility of probe nanostructure. Moreover, acid–base reactivity of this luminescent probe facilitates effective binding of analytes on the surface of nanomaterial due to the formation of Meisenheimer complexes between electron-rich amine groups and electron deficient analytes.44
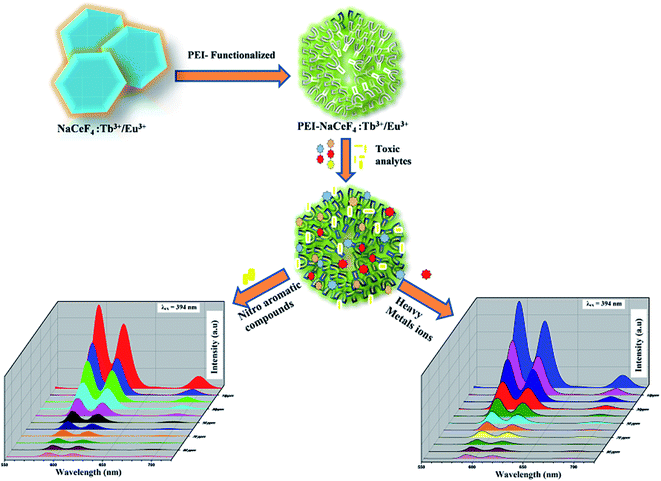 |
| Scheme 1 Schematic procedure for the synthesis of NaCeF4:Tb3+/Eu3+ (Eu-7%) and PEI functionalized NaCeF4:Tb3+/Eu3+ (Eu-7%) nanophosphors. | |
Additionally, to unravel the effect of graphene derivatives on the photoluminescence properties of the nanophosphor, R-GO@PEI-NaCeF4:Tb3+/Eu3+ nanocomposite was synthesized with and without the functionalizing material. The results showed a remarkable improvement in the PL intensity resulting in the red emission of nanocomposite with the addition of the amine rich PEI ligand rather than without the PEI attached nanocomposite.
Experimental section
Chemicals and materials
All the chemicals used in the work including terbium(III) nitrate hexahydrate Tb (NO3)3·6H2O (99.9%), cerium(III) nitrate hexahydrate Ce (NO3)3·6H2O (99.5%), europium(III) nitrate hexahydrate Eu (NO3)3·6H2O (99.7%), ammonium tetrafluoroborate NH4BF4 and ethylenediaminetetraacetic acid (EDTA) were purchased from Alfa Aesar. Nitric acid (HNO3), phosphoric acid (H3PO4), potassium permanganate KMnO4 and ethanol all were of analytical grade. Graphite flakes and polyethylenimine (PEI) were procured from Sigma Aldrich. All the chemicals were used without further purification. Tripled time distilled water was used for experiments.
Synthesis of NaCeF4:Tb3+ nanophosphors
In a usual procedure, a series of Tb doped NaCeF4 phosphors were fabricated via modified hydrothermal method at varying temperature conditions. In a typical synthesis method Tb (NO3)3·6H2O (0.05 mmol, 0.0217 g), Ce (NO3)3·6H2O (0.95 mmol, 0.4125 g) were dissolved in 10 mL of distilled water and further stirred for 10 minutes for proper mixing of both the metal ions. Separately, 0.37 g (1 mmol) of Na2EDTA was added to 5 mL of distilled water and stirred for 15 minutes. After continuous stirring, the two solutions were mixed together and agitated for half an hour at room temperature. To the resultant reaction mixture, 5 mL aqueous solution of NaOH was drop wise added under continuous stirring followed by addition of 10 mL of ethanol. Lastly, 2 mL aqueous solution of NH4BF4 (6 mmol, 0.629 g) was drop wise added into the reaction solution followed by vigorous stirring for 1 hour at room temperature. The resultant precursor solution was finally transferred into a 50 mL Teflon-lined autoclave and heated at 120 °C for 24 h. The reaction vessel was naturally cooled down at room temperature. The obtained product was separated by centrifugation and repeatedly washed with distilled water followed by 10% ethanol. The product obtained was finally dried at 70–80 °C for 12 h. Three more samples with the same chemical composition were synthesized under similar experimental conditions, at varying temperatures (140 °C, 160 °C, and 180 °C) for 24 h.
Synthesis of NaCeF4:Tb3+ co-doped Eu3+
In a similar procedure, NaCeF4:Tb3+ co-doped Eu3+ nanostructures were synthesized with 5% Tb3+ doping and with varying Eu3+ ion concentrations (1%, 3%, 5%, 7%). Na2EDTA was chosen as surfactant or surface directing agent for better morphology of the nanoparticles. Tb (NO3)3·6H2O (0.05 mmol, 0.0217 g), Ce(NO3)3·6H2O (0.94 mmol, 0.408 g) and Eu(NO3)3·6H2O (0.01 mmol, 0.004 g) were dispersed in 7 mL of distilled water and stirred for about 10 minutes until complete dissolution. 0.37 g (1 mmol) of Na2EDTA was added to 7 mL of distilled water and stirred for further 10 minutes. The two solutions were then mixed together and continuously stirred for extra 30 minutes. Additionally, 5 mL aqueous solution of NaOH was added drop wise followed by 10 mL of ethanol and 2 mL solution of NH4BF4 (6 mmol, 0.629 g) under vigorous stirring for 1.5 h at room temperature. The resultant reaction mixture was then transferred into a 50 mL Teflon-lined autoclave and the reaction vessel was heated at 160 °C for 24 h. The reaction vessel was cooled down naturally and the product so obtained was centrifuged and washed three times with distilled water followed by ethanol. The product was finally dried at 60 °C for 24 h.
Under similar experimental conditions, three more samples were synthesized at same temperature conditions like (160 °C at 24 h) but different Eu ion concentrations (3%, 5% and 7%).
PEI-surface functionalization of nanophosphor
In a typical hydrothermal method, 0.01 g of as synthesized NaCeF4:Tb3+/Eu3+ (Eu-7%) sample was dispersed in a 10 mL of water and sonicated for 30 minutes. Furthermore, 0.02 g of PEI was separately mixed in 10 mL of water and sonicated for about 15 minutes until proper mixing. Finally, dropwise PEI solution was added to the above sonicated solution with further addition of 5 mL ethanol followed by vigorous stirring for about 1 h. The surface-functionalized nanomaterial PEI @NaCeF4:Tb3+/Eu3+ (Eu-7%) so obtained was separated by centrifugation, washed twice with distilled water followed by ethanol washing and finally vacuum dried at room temperature for 24 h.
Synthesis of graphene oxide (GO)
Graphene oxide (GO) was prepared by one-pot synthesis gleaned from new improved Hummers method.45 Natural graphite flakes were used as the precursor material and KMnO4 as the oxidising agent for the preparation of GO. In a typical procedure, 1 g of pure graphite flakes were dissolved in a solution containing stichometric ratio 9
:
1 of sulphuric acid and phosphoric acid and vigorously stirred for about 6 h. The suspension mixture was then kept in an ice bath and the temperature was adjusted below 5 °C. Next, 8 g of KMnO4 was added slowly into the mixture while stirring followed by stirring for 1.5 h. The resulting green color suspension was further stirred in a water bath (40 °C) for 2 h. The mixture was then adjusted to a linearly high temperature of 70 °C and stirred for about 45 minutes. Later, distilled water was added to the brown color suspension mixture and then transferred into beaker containing 400
mL of additional water. In order to terminate the oxidation process 15
mL of H2O2 was added and mixed vigorously for 10 minutes imparting yellow color. The product obtained was finally separated by centrifugation and then repeatedly decant off with distilled water followed by 10% HCl solution. Lastly, the solid product was dried under vacuum at room temperature and product was obtained as GO powder. Additionally, the reduced graphene oxide (R-GO) was synthesized by simple hydrothermal method using hydrazine hydrate and ammonia as the reducing agent as described in the literature.46
Synthesis of R-GO@PEI@NaCeF4:Tb3+/Eu3+
A facile hydrothermal route was used for the synthesis of nanocomposite material. 0.02 g of R-GO was dispersed in 15 mL distilled water and sonicated for 40 minutes. Separately, 0.08 g of as-synthesized PEI-functionalized NaCeF4:Tb3+/Eu3+ (Eu-7%) nanomaterial was dispersed in 30 mL of distilled water and sonicated for 20 minutes. The two solutions were mixed together followed by addition of 7 mL ethanol and stirred for 1 h. Finally, the suspension mixture was centrifuged and washed twice with distilled water followed by 10% HCl solution. The solid product was vacuum dried at room temperature for 24 h.
Spectroscopic and microscopic measurement
X-Ray diffraction (XRD) data was used to determine the phase purity and crystallite size of as-synthesized nanophosphors. The XRD data was collected using D8 X-ray diffractometer (Bruker) at scanning rate of 12 min−1 in the 2θ range of 10 to 80° with Cu Kα radiation (λ = 0.15405 nm). Field emission scanning electron micrographs (FE-SEM) were recorded on FEI Nova Nano SEM-450 to examine the external morphology and particles size of as-synthesized nanophosphors. The internal composition, lattice parameters, grain size and morphology was recorded by using transmission electron microscopy (TEM) and high-resolution TEM (HR-TEM). The high-resolution TEM (HR-TEM) was recorded on Tecnai G2 S-Twin transmission electron microscope having field emission gun operating at 200 kV. The presence of surface directing agent as well as the functionalized materials which was assimilated on the surface of nanocrystals was explored by Shimadzu Fourier Transform Infrared Spectrophotometer (FT-IR) using standard KBr pellet technique (4000–400 cm−1). For UV-visible down conversion photoluminescence emission spectra of as-synthesized nanophosphors Hitachi F-4700 Fluorescence Spectrophotometer having xenon lamp as excitation source was employed.
Sensing of hazardous nitroaromatics and heavy metal ions
The Photoluminescence detection experiment for the sensing of heavy metal ions and explosive aromatic nitro compounds were carried out on Hitachi F-4700 Fluorescence Spectrophotometer at room temperature. PEI-functionalized NaCeF4:Tb3+/Eu3+ nanostructure (0.03 g) was dispersed in 30 mL of distilled water and sonicated for around 20 minutes. Six stock solutions of explosives were prepared by dissolving 0.1 g of each of nitroaromatic compounds as well as heavy metal ions in 100 mL of water (1000 ppm solution). From the stock solutions, ten different solutions of each compound were prepared by simple dilution having concentrations ranging from 10–100 ppm. To each dilute solution, 2 mL of as prepared sample suspension was added. The florescence spectrum of each solution was recorded immediately. Herein, for sensing of nitro-compounds as well as heavy metals ions the luminescence spectra was analyzed by exciting the PEI-functionalized NaCeF4:Tb3+/Eu3+ luminescent material selectively at 394 nm for the solution phase at variant ppm concentrations.
Result and discussion
Powder X-ray diffraction
The composition, phase evolution, purity, and size of the as-prepared sodium cerium fluoride core doped with rare earth metals ions were confirmed by PXRD technique. A series of NaCeF4:Tb3+ nanocrystals samples were synthesized keeping the reaction time and concentration of the metals ions as well as the concentration of the surfactant (EDTA) constant while varying the reaction temperature. Fig S1: ESI† shows the diffraction pattern of synthesized NaCeF4:Tb3+ nanophosphors at different heating temperatures. All the diffraction peaks are in good agreement with the JCPDS no. 75-1924.47 Furthermore, to explore the effect of doping ions concentration on the structure of nanophosphors, another series of NaCeF4:Tb3+/Eu3+ nanocrystals with different Eu3+ ion concentration were prepared keeping the reaction time, temperature and molar concentration of surfactant constant. Fig. 1 shows the diffraction pattern of the as-synthesized nanophosphors. It was observed that all the diffraction peaks of nanocrystals are in accordance with the JCPDS no. 75-1924 and are perfectly indexed to pure hexagonal phase. From the XRD pattern (Fig. 1), the Eu3+ concentrations do not cause any difference in the crystal phase and structure of nanophosphors. All the diffraction peaks can be readily indexed as the hexagonal NaCeF4, agreeing well with the data reported in the JCPDS no. 75-1924.48 However, only small variation in particles size can be seen. The powder XRD patterns of as-synthesized nanocrystals were systematically investigated and no extra impurity peak was observed clearly indicating that Tb3+ and Eu3+ ions were perfectly dissolved in the NaCeF4 host lattice without further alteration in the crystal structure.
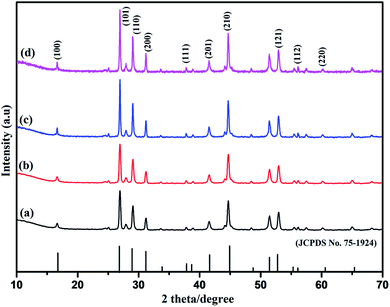 |
| Fig. 1 PXRD patterns of as-synthesized NaCeF4:Tb3+/Eu3+ (x%) nanoparticles with different Eu3+ ion concentrations: (where x = (a) 1% (b) 3% (c) 5% (d) 7%). | |
It was also observed that no additional peak was noticed in the spectra of nanophosphors with amine rich ligand (polyethylenimine (PEI)). However the diffraction pattern gets broadened which signify the nanoscale range of as-synthesized nanocrystals as shown in Fig. 2.
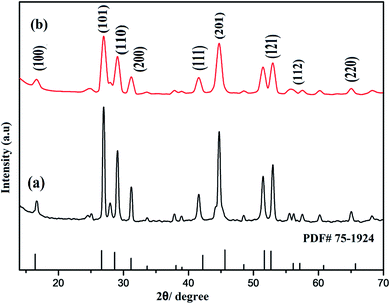 |
| Fig. 2 Comparison of PXRD patterns of prepared nanostructures: (a) NaCeF4:Tb3+/Eu3+ (Eu-7%) (b) PEI@ NaCeF4:Tb3+/Eu3+ (Eu-7%). | |
Scherrer's eqn (1) was applied to calculate the crystallite sizes of the as-synthesized nanomaterial:49
|
 | (1) |
Here,
L (nm) is the crystallite size,
K is the Scherrer constant (equals to 0.89),
λ (nm) is the wavelength of the Cu Kα radiant,
λ = 0.15405 nm, 2
θ is the diffraction angle,
β is the full-width at half-maximum (FWHM) of the diffraction peak. The average crystallite size was calculated by using the major diffraction peaks of nanocrystals. The average crystallite size of NaCeF
4:Tb
3+/Eu
3+ for nanostructures were observed in the range of 15 to 21 nm.
Furthermore, the XRD pattern of R-GO was systematically examined. A strong characteristics peak centered at 2θ = 23° corresponding to (002) plane and additional small peak around 2θ = 45° were observed as shown the Fig. 3. The presence of wide peak width clearly demonstrates the small crystallite size and short layered stacked structure of R-GO.50
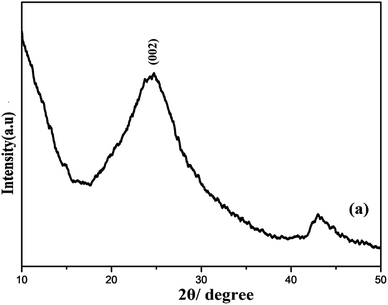 |
| Fig. 3 PXRD pattern of (a) R-GO. | |
Energy dispersive X-ray spectroscopic analysis
The energy dispersive X-ray spectroscopy (EDS) analysis was used to determine the exact elemental content in the prepared samples. The result obtained is tabulated in Tables S1 and S2: ESI.† The spatial distribution of elements involves Na, Ce, Tb, Eu, and F in the as-prepared NaCeF4:Tb3+ and NaCeF4:Tb3+/Eu3+ nanophosphors. Furthermore, the presence of additional elements like N, O and C in the EDS spectrum validates the formation of PEI-functionalized nanoparticles. The presence of crucial element like C in the R-GO and R-GO@PEI@NaCeF4:Tb3+/Eu3+ nanocomposite samples confirms the formation of carbon based R-GO and R-GO doped nanocomposite. The spectral peaks of samples computing the presence of Y, Ce, Gd, Eu, Tb, Na, C, N, O and F elements are shown in Fig. S2–S4: ESI.†
Scanning electron microscopy and high-resolution transmission electron microscopy
The Field emission Scanning Electron Microscopy (FE-SEM) Transmission Electron Microscopy (TEM) and high-resolution Transmission Electron Microscopy (HR-TEM) analysis was utilized to monitor the shape, size and morphology of nanomaterials. Fig. 4 displays the FE-SEM micrographs of synthesized NaCeF4:Tb3+ at varying reaction parameters. The morphology of the nanophosphors was systematically tuned by heating temperature from 120 °C to 180 °C. During the growth mechanism, NaCeF4:Tb3+ exhibited two different crystal morphologies and phase transformation occurred from kinetically stable cubic shape (α-phase) at 120 °C to thermodynamically stable hexagonal shape (β-phase) at around 160 °C.51 It was observed from the micrographs that the 160 °C is a suitable temperature resulting in smooth-surfaced pure hexagonal-shaped nanoparticles. To further monitor the effect of Eu3+ ions, as-synthesized NaCeF4:Tb3+/Eu3+ aliquots were analyzed (with varying Eu3+ contents from 1 to 7%) and results are shown in Fig. 5. The Eu3+ ion concentration caused tunable effect on the morphology and size of the resultant nanoparticles architecture, ranging from smooth-surfaced 2D hexagonal plate-like morphology in the early stage (1–3%) to aggregated broad sized hexagonal rods at the later stage of the reaction bearing a higher concentration of Eu3+ (7%). The overall result showed thatEu3+ ion exhibits dual-mode character, showing active luminescent property and also notable morphological agent for the synthesized nanostructure reaction. Hence, the reaction temperatures as well as metal ion concentration emerge as important factors in adapting an improved morphology and shape of nanomaterial. Fig. S5: ESI† displays the TEM micrographs of NaCeF4:Tb3+/Eu3+ showing hexagonal shape and PEI-NaCeF4:Tb3+/Eu3+ confirming the presence of a aggregated layer-like structures on to the surface of PEI capped hexagonal nanophosphor.52
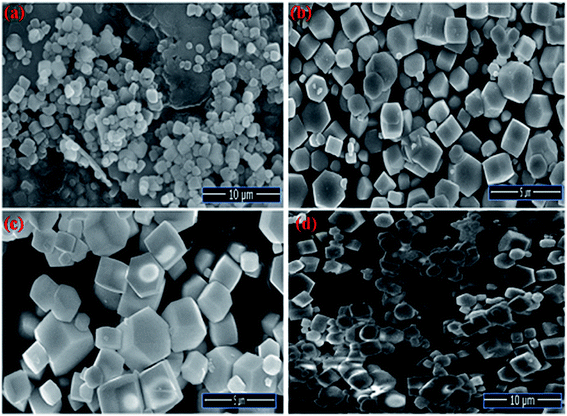 |
| Fig. 4 FESEM micrographs depicting the variation in the morphology of nanophosphors from cubic to hexagonal NaCeF4:Tb3+ at different temperatures (a) 120 °C (b) 140 °C (c) 160 °C (d) 180 °C. | |
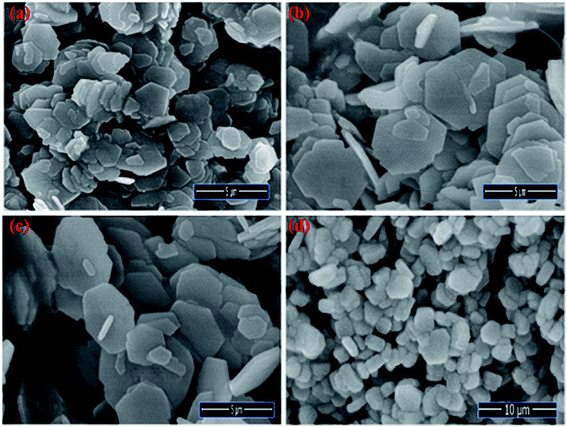 |
| Fig. 5 FE-SEM images visualizing the morphological effect of Eu3+ ion contents on the hexagonal NaCeF4:Tb3+/Eu3+ nanophosphors (a) 1% (b) 3% (c) 5% (d) 7%. | |
Furthermore, the FE-SEM and TEM micrographs of composites of R-GO with nanophosphor were also analyzed to explore the morphological effect of sheets like R-GO on the luminescent nanophosphor as shown in Fig. 6 and 7. The result noticed the transparent crumbled sheet like the layered structure of R-GO. Fig. 6(b) and 7(b) display the FE-SEM and TEM micrographs of the synthesized R-GO@PEI-NaCeF4:Tb3+/Eu3+ nanocomposite depicting the exquisite doping of hexagonal-shaped PEI-NaCeF4:Tb3+/Eu3+ nanoparticles onto the R-GO sheets.
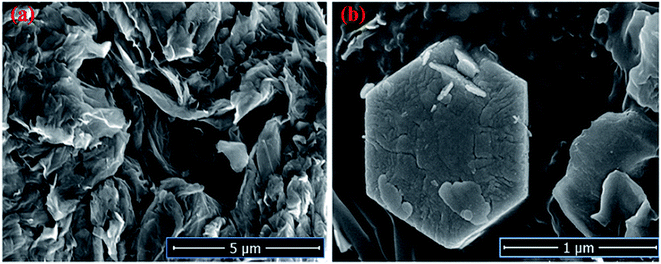 |
| Fig. 6 FE-SEM micrographs of (a) R-GO (b) RGO@PEI@NaCeF4:Tb3+/Eu3+ (Eu-7%) nanocomposite. | |
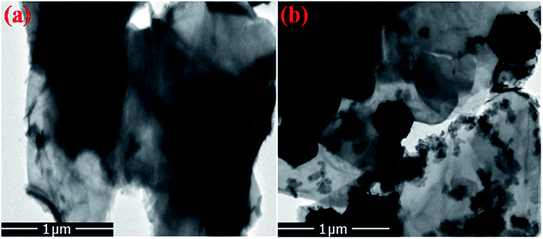 |
| Fig. 7 TEM images of (a) R-GO (b) RGO@PEI@NaCeF4:Tb3+/Eu3+ (Eu-7%) nanocomposite showing the presence of hexagonal nanoparticles anchored on the R-GO sheets. | |
The high-resolution transmission electron microscopy (HR-TEM) images confirm the high crystallinity of the prepared nanophosphors (see Fig. S6 and S7: ESI†). From the micrographs (shown in Fig. 8), it is indicated that the lattice fringe spacing between interlayer planes lies in the range of 0.27 nm to 0.3 nm, which is well indexed with the (101) lattice plane of the hexagonal NaCeF4 structure.53 The existence of ordered lattice fringes corresponding to the hexagonal phase is in good agreement with the results of the X-ray diffraction (XRD) patterns. The nanophosphors result in preferential growth orientation along the 002 direction.
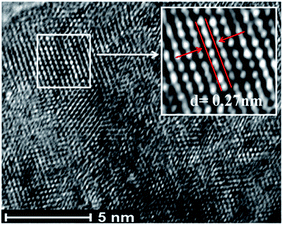 |
| Fig. 8 HR-TEM micrograph showing PEI-functionalized NaCeF4:Tb3+/Eu3+ (Eu-7%) nanostructure. | |
Infrared spectroscopy
FT-IR spectroscopy was used to explore the presence of functional groups in the synthesized NaCeF4:Tb3+/Eu3+ nanocrystals as well as for the PEI functionalized NaCeF4:Tb3+/Eu3+ nanophosphors as shown in Fig. S8: ESI.† The FT-IR spectra were selectively recorded within range of the wave number 4000–400 cm−1. The IR spectra revealed intense broad absorption band at 3400 cm−1 assigning to stretching vibration of the adsorbed –OH molecules on the surface of NaCeF4:Tb3+/Eu3+ nanophosphors. The bands at 2924 and 2850 cm−1 correspond to asymmetrical and symmetrical stretching vibration modes of the methylene group (–CH2) respectively. The bands around 1640 and 1400 cm−1 are attributed to asymmetrical and symmetrical stretching of the carboxylate (–COO) group.54 The appearance of these IR bands confirms the presence of EDTA in the NaCeF4:Tb3+ and NaCeF4:Tb3+/Eu3+ nanocrystals.
Meanwhile, to monitor the proper functionalization of PEI onto the surface of nanoparticles the FT-IR spectrum of the prepared sample was recorded and compared with that of pure NaCeF4:Tb3+/Eu3+ nanocrystals fabricated without PEI Fig. S9: ESI.† The existence of PEI is confirmed by the presence of the characteristic absorption peaks at 3389 cm−1 corresponds to N–H stretching vibration of the NH2 group. The band around 1380–1630 cm−1 is due to vibration of the amide group.55 For the additional bonding with any external ligand, the presence of free amine groups on the surface of the nanoparticles is of extreme importance as these can bind with the outer molecules. The band at 1527 cm−1 (Fig. S9: ESI†) corresponding to amine groups confirms the presence of free amine groups still vacant on the surface nanoparticles for further attachment.56 An absorption band around 1391 cm−1 is attributed to stretching vibrations of the C–N bond of amino acid (see Fig. S9: ESI†). Meanwhile, it was interesting to note that all the above bands were not observed in the pure EDTA capped nanocrystals confirming the successful functionalization of PEI on to the nanophosphor.
Additionally, FT-IR spectrum was further recorded to investigate the presence of functional groups and bonding interaction of the GO, R-GO and the PEI-NaCeF4:Tb3+/Eu3+@R-GO nanocomposite as depicted in Fig. S10: ESI.† The occurrence of characteristic peak at 3430 cm−1 is attributed to the stretching vibration of hydroxyl groups (–OH) present on the GO sheets. The IR spectrum of GO demonstrates the presence of abundant oxygen containing functional moieties including C
O functional groups (band around 1734 cm−1) and epoxy groups (asymmetric vibration at 1232 cm−1).57 There occurs a drastic change in the IR spectrum of R-GO where shift in bands is observed from 1628 cm−1 to around 1586 cm−1 due to the reduction process.58 Fig. S11: ESI† displays the FT-IR spectrum of the fabricated PEI-NaCeF4:Tb3+/Eu3+@R-GO nanocomposite confirming the successfully binding interaction of various functional moieties remaining on the R-GO sheets with the nanophosphor. The shift in the band positions confirms the sufficient anchoring of metal nanophosphor onto the R-GO layered structure.
Photoluminescent properties
The photoluminescence spectra of the synthesized Tb3+ doped NaCeF4 nanostructures was monitored under single excitation wavelength 247 nm as shown in Fig. S12: ESI.† The emission peaks centred at 490, 545, 585 and 621 nm are corresponding to various transitions resulting from excited state 5D4 to ground state 7FJ (J = 6, 5, 4, 3) respectively. The presence of strong peak at 545 nm i.e., 5D4 → 7F5 originates due to the magnetic-dipole and electric-dipole allowed transition.59 The emission from Tb3+ is sensitized by Ce3+.
The photoluminescence spectra of NaCeF4:Tb3+/Eu3+ nanophosphors with varying Eu3+ concentrations (1%, 3%, 5% and 7%) were also recorded to investigate the luminescence behaviour and effect of different Eu3+ ion concentrations on the PL intensity as displayed in Fig. 9. The Ce3+ ion (having 4f–5d allowed transition) can also sensitize Eu3+ ion which can act as an activator and improve the luminescence competency of the nanophosphors.60 However, direct sensitization of Eu3+ from Ce3+ is prohibited due to metal to metal electron charge transfer (MMCT) i.e., Ce3+ + Eu3+ → Ce4+ + Eu2+ resulting in abrupt fall off photoluminescence intensity.61 Tb3+ ion (green-emitter) can be employed as bridging material for improved energy transfer from the Ce3+ ion to Eu3+ ion with minimum quenching effect. The results observed indicate effective energy transfer with superior luminescence emission.62 Thus, apart from being an efficient activator, Tb3+ can also be employed as a competent mediator between the sensitizer (Ce3+) and activator (Eu3+) resulting in minimizing the MMCT quenching.63 Consequently, there occur well-built green orange emissions under ultraviolet excitation occurring due to energy transfer (ET) from Ce3+ → Tb3+ and Tb3+ → Eu3+.
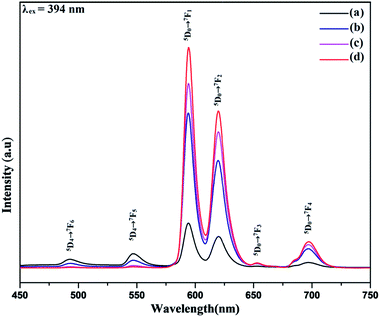 |
| Fig. 9 Room-temperature photoluminescent emission spectra of NaCeF4:Tb3+/Eu3+ displaying different emissions at varying Eu3+ concentrations (a) 1% (b) 3% (c) 5% (d) 7%. | |
The Photoluminescence spectra of the NaCeF4:Tb3+/Eu3+ with different Eu3+ concentrations displayed in Fig. 9 reveal the existence of four characteristics emission peaks (under 394 nm excitation) arising from different transitions centered at 594 (5D0 → 7F1), 624 (5D0 → 7F2), 657 (5D0 → 7F3), 700 (5D0 → 7F4). The peak centered at 657 nm (5D0 → 7F3) and 700 nm (5D0 → 7F4) may be due to magnetic and electric-dipole forbidden transitions of NaCeF4:Tb3+/Eu3+ nanophosphors.64 The PL intensity varies with the change in Eu3+ ion concentrations. The PL spectrum (Fig. 9) shows an enhancement in the luminescence intensity with the increase in the Eu3+ concentrations ranging from 1% to 7% as displayed. From the graph it is observed that sample having Eu3+ ion 7% content achieved maximum luminescence intensity showing efficient energy transfer to the Eu3+ ion. It is quite interesting to note that the peaks occurring at 493 and 548 nm correspond to the characteristic emission peaks of Tb3+. The decrease in the Tb3+ peaks with increase in the Eu3+ concentration manifest the effective energy transfer from Tb3+ to Eu3+ ions as shown in Fig. 9.
Furthermore, the PL spectrum of the PEI functionalized nanophosphor was also investigated showing improved photoluminescence intensity of the nanophosphor as depicted in Fig. 10. The PL emission spectrum shows an enhancement in the emission intensity as compared to the NaCeF4:Tb3+/Eu3+ nanophosphor. In order to investigate the energy transfer process in the nanophosphor the life time decay curve was measured. The lifetime of NaCeF4:Tb3+ and NaCeF4:Tb3+/Eu3+ nanocrystals were recorded by taking into consideration the excitation and emission wavelength at 394 and 610 nm respectively (see Fig. S13 and S14: ESI†). The luminescence decay curves NaCeF4:Tb3+/Eu3+ are in good agreement with the single exponential function I = I0 + A
exp(t/τ) (where I and I0 are luminescence intensities at the time ‘t’ and ‘0’, the term τ stands for photoluminescence decay time). The lifetimes of NaCeF4:Tb3+ measured are in the range of 125 ms to 128 ms while the lifetimes of the Eu3+ doped NaCeF4:Tb3+ (at different Eu3+ concentrations) and PEI functionalized NaCeF4:Tb3+/Eu3+ varies from 131 ms to 138 ms. The NaCeF4:Tb3+/Eu3+ exhibit much longer lifetime as compared to the Tb3+ doped nanophosphors suggesting an improved energy transfer process between the adjacent metals ions.
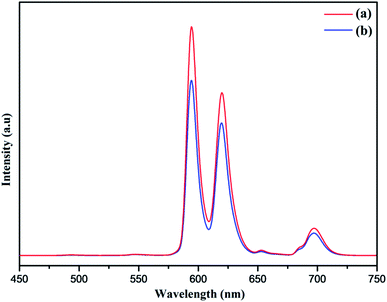 |
| Fig. 10 Room-temperature photoluminescent emission spectra of (a) PEI-NaCeF4:Tb3+/Eu3+ (Eu-7%) (b) NaCeF4:Tb3+/Eu3+ (Eu-7%). | |
Luminescent behavior and sensing properties
Lanthanide-doped nanocrystals due to their unique luminescence properties (such as their excellent chemical stability, narrow bandwidth emission, photo stability, tunable emission color) turn out promising candidates for luminescent based materials.65 The photoluminescent spectra of synthesized PEI functionalized NaCeF4:Tb3+/Eu3+ nanophosphor was explored for the fluorescence detection of toxic metal ions and hazardous nitro-aromatics compounds. It has been systematically demonstrated that PEI-functionalized NaCeF4:Tb3+/Eu3+ nanophosphor can easily and efficiently sense the aromatic nitro compounds as well as toxic heavy metal ions even at trace level. All the photo luminescent spectra were recorded at room temperature using water as the dispersion medium. As shown in Fig. 10, PEI capped NaCeF4:Tb3+/Eu3+ nanophosphor displays a sharp emission peak centered at 594 and 624 nm under λex = 394 nm when dispersed in water.
Sensing of toxic heavy metal ions
To unfold the fluorescence sensing ability of the synthesized nanophosphor utilized for the detection of toxic heavy metal ions (Cd2+, Fe3+, Cr3+, Co2+) a series of luminescence-based experiments were performed. Equal amounts of the metals ions were added to the dispersed solution of nanophosphor. The suspension was taken in a 4 mm cuvette for luminescence measurement and compared with the luminescence intensity of the bare sample without the addition of analytes. Similar experiments were performed with other metal ions. The results demonstrated that the luminescence emission intensity of nanophosphor gets significantly influenced with the addition of different metal analytes bearing different concentrations ranging from 0 to 100 ppm. It was observed that the PL intensity is significantly quenched by the addition of metals ions and results obtained are displayed in Fig. 11 to 14 (for various metals analytes). The detection mechanism was strictly based on the interaction of the lone pair of NH2 group present on the surface of nanophosphor with the metals ions leading to charge transfer from the electron rich nanophosphor material to the vacant metal ions resulting in luminescence quenching and ultimate metal sensing. Meanwhile, it was observed that 100 ppm solution of metal ion completely quenched the luminescence intensity of the nanophosphor.
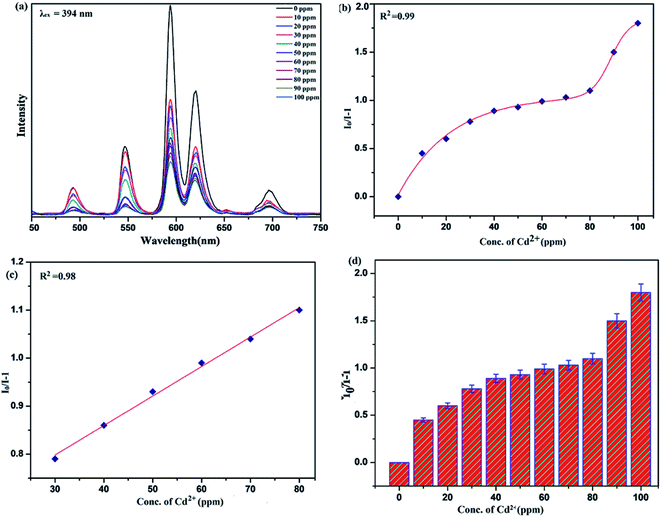 |
| Fig. 11 Variation in photoluminescence intensity of nanosensor in presence of different Cd2+ concentrations (from 0 to 100 ppm) selective excitation at λex = 394 nm: (a) emission spectra of nanophosphors with addition of Cd2+ ion (b) nonlinear Stern–Volmer plot of I0/I − 1 versus (Cd2+) concentrations (c) linear Stern–Volmer fitting and (d) error bar. | |
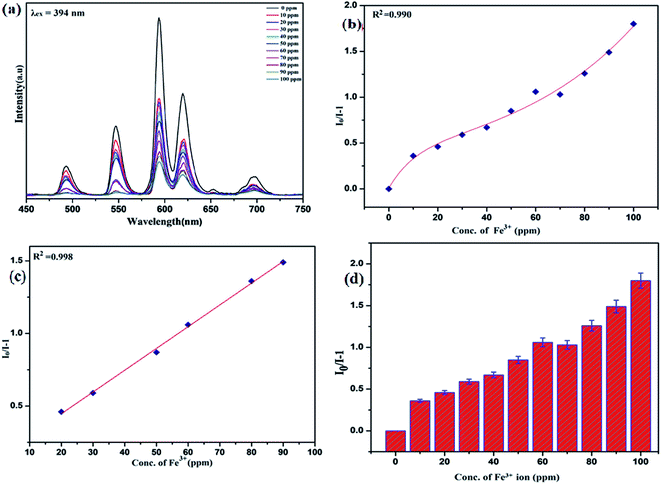 |
| Fig. 12 Variation in photoluminescence intensity of nanosensor in presence of different Fe3+ concentrations (from 0 to 100 ppm) selective excitation at λex = 394 nm: (a) emission spectra of nanophosphors with addition of Fe3+ ion (b) nonlinear Stern–Volmer plot of I0/I − 1 versus (Fe3+) concentrations (c) linear Stern–Volmer fitting and (d) error bar. | |
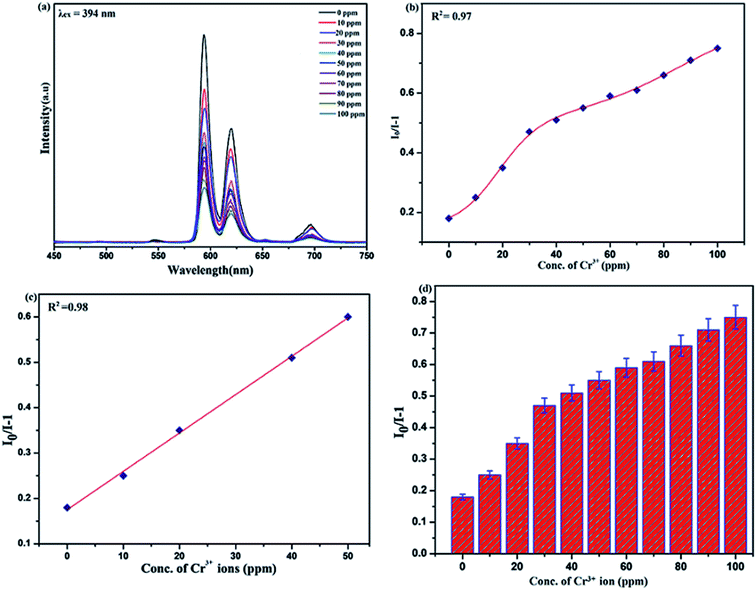 |
| Fig. 13 Variation in photoluminescence intensity of nanosensor in presence of different Cr3+ concentrations (from 0 to 100 ppm) selective excitation at λex = 394 nm (a) emission spectra of nanophosphors with addition of Cr3+ ion (b) nonlinear Stern–Volmer plot of I0/I − 1 versus (Cr3+) concentrations (c) linear Stern–Volmer fitting and (d) error bar. | |
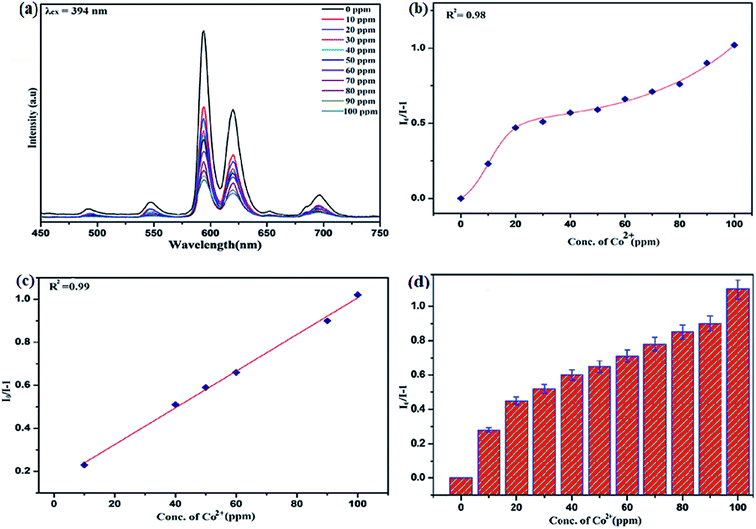 |
| Fig. 14 Variation in photoluminescence intensity of nanosensor in presence of different Co2+ concentrations (from 0 to 100 ppm) selective excitation at λex = 394 nm (a) emission spectra of nanophosphors with addition of Co2+ ion (b) nonlinear Stern–Volmer plot of I0/I − 1 versus (Co2+) concentrations (c) linear Stern–Volmer fitting and (d) error bar. | |
The gradual increased luminescence quenching with increase in the concentration of analytes was analyzed by using the Stern–Volmer (SV) equation.66
Here,
I0 is the luminescence intensity in the absence of analytes, and
I is the luminescence intensity in the presence of metals ions,
Q is the concentration of analytes and
KSV is the Stern–Volmer (SV) quenching constant,
kq is the bimolecular quenching rate constant and
τf is radiative life time. The affinity between PEI-functionalized nanophosphor (Eu-7%) and analytes (heavy metal ions and aromatic nitrocompounds) corresponding to
KSV follows the order Fe
3+ > Cr
3+ > Co
2+ > Cd
2+. The calculated
KSV values for Fe
3+, Cr
3+, Co
2+, Cd
2+ are 3.8 × 10
4 M
−1, 2.6 × 10
4 M
−1, 1.8 × 10
4 M
−1, 0.85 × 10
4 M
−1 respectively. The Fe
3+ ions exhibited highest
KSV value as compared to other metals ions which suggest high selectivity of the PEI-functionalized NaCeF
4:Tb
3+/Eu
3+ (Eu-7%) nanophosphors for Fe
3+ ion. The quenching efficiency for investigated heavy metal ions is found in a range of 73 to 90.02% (see Fig. S13: ESI
†).
Sensing of nitro-aromatic compounds
Benefiting from the above sensing properties of fabricated nanophosphor which could significantly quench the emission in aqueous media, the ability of this nanoprobe was again investigated to sense harmful nitro aromatics at even very low concentration. Similar detection experiment were performed considering selected toxic aromatic nitro compounds like picric acid (PA), para-nitrophenol (p-NP) para-nitroaniline (p-NA), and 4-nitrotoluene (4-NT). The photoluminescence results clearly indicate the selective and sensitive detection of nitro analytes by PEI-functionalized NaCeF4:Tb3+/Eu3+ nanophosphor at trace level. The sensing principle is purely based on the charge transfer from the lone pairs of the amine group present on the nanophosphor with the electron deficient aromatic nitro compounds. This electronic interaction between the electron rich and electron deficient moieties leads to photoluminescence quenching. The effect of these different moieties on photo physical properties of nanophosphor was studied, by analyzing the emission spectra of PEI-functionalized compound in distilled water excited at 394 nm in presence of varying concentrations of analytes. It was interestingly noticed that the luminescent intensity of the nanophosphor was significantly affected by addition of various concentration of aromatic nitro-compound ranging from 0 to 100 ppm. The experiments were also performed under similar conditions with NaCeF4:Tb3+/Eu3+ (Eu-7%) nanophosphor without PEI functionalization. The results showed subdued response of luminescence intensity of nanophosphor in presence of analytes. Furthermore, nitro compounds lead to significant quenching of the luminescence intensity of the PEI functionalized nanophosphor and the results obtained are displayed in Figs. 15 to 18 (for different nitro analytes). It is again observed that for 100 ppm solution of picric acid the quenching efficiency is about 97.05%. Thus, when compared with the other nitro analyte solutions, picric acid causes maximum quenching of the luminescence intensity as depicted in Fig. S14: ESI.† Consequently, it can be postulated that the PEI-functionalized Tb3+/Eu3+ co-doped NaCeF4 nanophosphor can act as an effective chemo sensors for the sensing of ultra trace amount of harmful picric acid analyte in aqueous solution. The calculated KSV of nitrocompounds follow the order PA (2.25 × 105 M−1) > p-NP (1.8 × 105 M−1) > p-NA (1.1 × 105 M−1) > 4-NT (0.88 × 105 M−1). The high KSV value for PA suggest the high selectivity of synthesized nanocrystals towards PA. Consequently, it was concluded that the KSV of Fe3+ and PA is quite high as compared to other analyte compounds suggesting superior selectivity of the chemosensor for Fe3+ and PA.
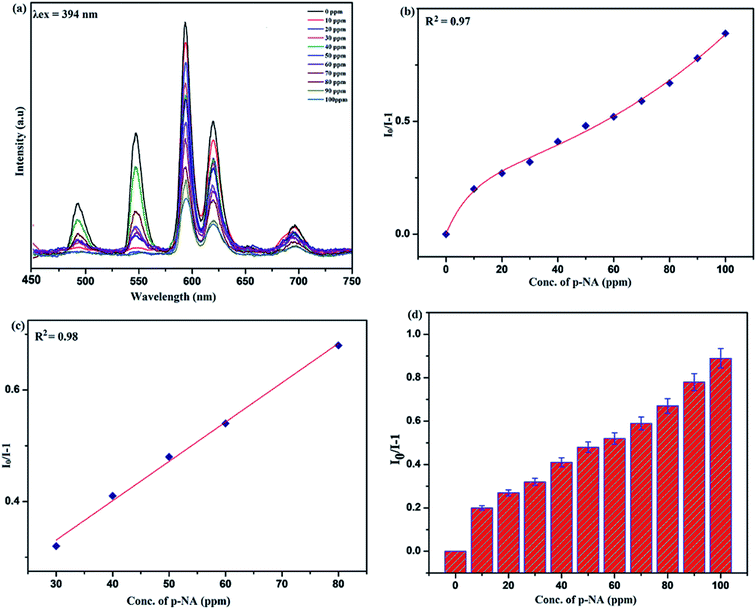 |
| Fig. 15 Variation in photoluminescence intensity of nanosensor in presence of different p-NA concentrations (from 0 to 100 ppm) selective excitation at λex = 394 nm: (a) emission spectra of nanophosphors with addition of p-NA (b) nonlinear Stern–Volmer plot of I0/I − 1 versus (p-NA) concentrations (c) linear Stern–Volmer fitting and (d) error bar. | |
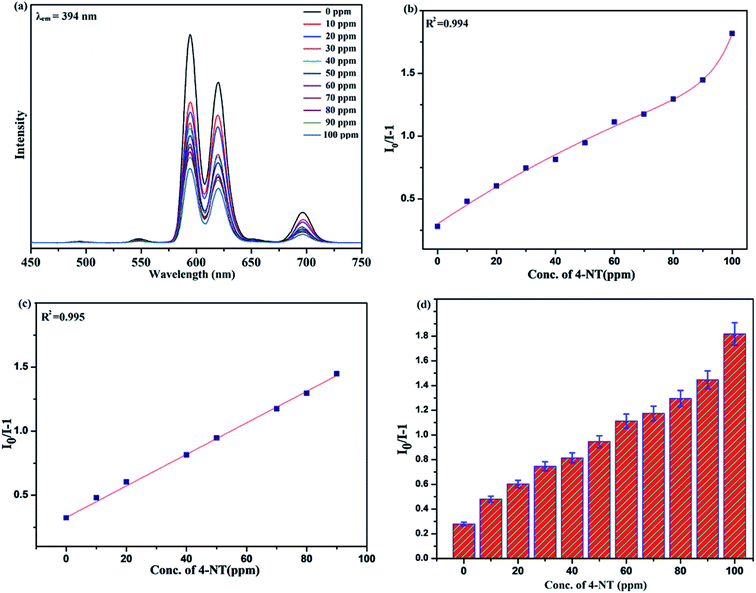 |
| Fig. 16 Variation in photoluminescence intensity of nanosensor in presence of different 4-NT concentrations (from 0 to 100 ppm) selective excitation at λex = 394 nm (a) emission spectra of nanophosphors with addition of 4-NT (b) nonlinear Stern–Volmer plot of I0/I − 1 versus (4-NT) concentrations (c) linear Stern–Volmer fitting and (d) error bar. | |
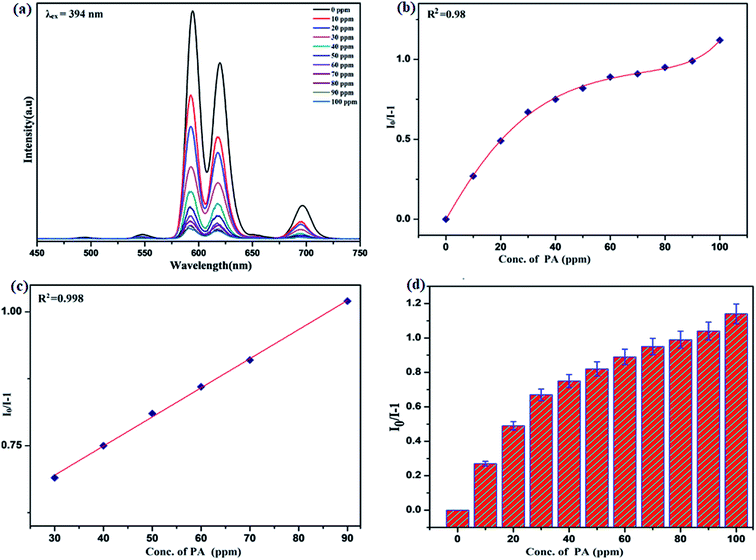 |
| Fig. 17 Variation in photoluminescence intensity of nanosensor in presence of different PA concentrations (from 0 to 100 ppm) selective excitation at λex = 394 nm: (a) emission spectra of nanophosphors with addition of PA (b) nonlinear Stern–Volmer plot of I0/I − 1 versus (PA) concentrations (c) linear Stern–Volmer fitting and (d) error bar. | |
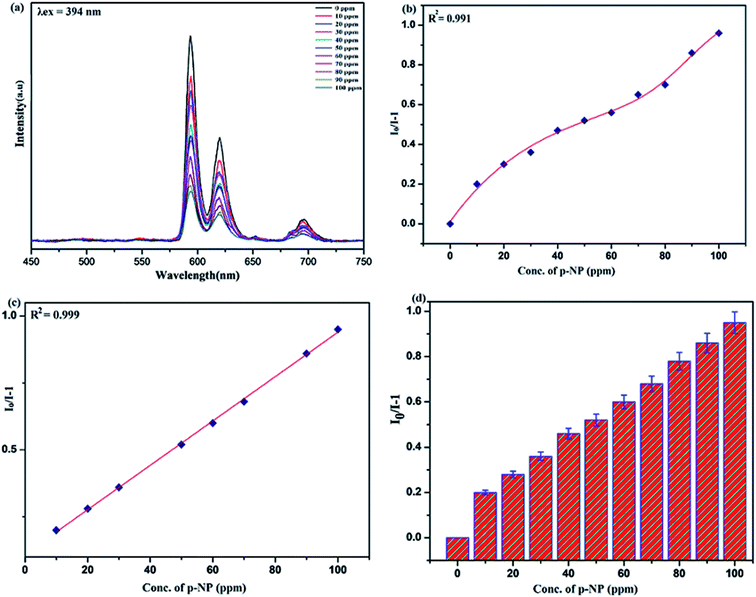 |
| Fig. 18 Variation in photoluminescence intensity of nanosensor in presence of different p-NP concentrations (from 0 to 100 ppm) selective excitation at λex = 394 nm: (a) emission spectra of nanophosphors with addition of p-NP (b) nonlinear Stern–Volmer plot of I0/I − 1 versus (p-NP) concentrations (c) linear Stern–Volmer fitting and (d) error bar. | |
By using linear regression method detection limit (LOD) for the sensing of different analytes were calculated using formula 3σ/S where 3 denotes the 95% confidence level factor; S is the slope of linear calibration curve and σ is the standard deviation of the observed intensity for the blank PEI-functionalized NaCeF4:Tb3+/Eu3+ (Eu-7%) nanostructure. The LOD for different aromatic nitrocompounds and heavy metal ions are as follow: 1.32 ppm (PA), 2.88 ppm (P-NP), 4.24 ppm (P-NA), 5.59 ppm (4-NT), 1.39 ppm (Fe3+), 2.58 ppm (Cr3+), 3.85 ppm (Co2+) and 7.48 ppm (Cd2+) respectively. The PA and Fe exhibit low detection limit as compare to that of other analytes and thereby suggested high sensitivity of the nanoprobe. As shown in Tables 1 and 2, the corresponding KSV and LOD of PA and Fe is higher than those of some previously reported.67–76
Table 1 Detection of nitroaromatic compounds by various methods from different nanomaterials
Sensing method |
Materials |
Detection of NACs |
KSV (M−1) |
LOD |
Reference |
Electrochemical method |
Cu2O |
PA |
— |
39 μM |
67 |
Electrochemical method |
Nano-gold/glassy carbon |
NP |
— |
8 μM |
68 |
Electrochemical method |
AuBFE |
PA |
— |
13.1 μM |
69 |
Fluorescence method |
TPE-CMPs |
2,4-DNT |
1.84 × 103 |
— |
70 |
2,6-DNT |
1.70 × 103 |
TNP |
1.19 × 104 |
Fluorescence method |
Tb-MOFs |
PA |
7.47 × 104 |
— |
71 |
Fluorescence method |
FIrPicOMPBHCz |
PA |
1.9 × 104 |
10 μM |
72 |
p-NP |
5.6 × 103 |
44 μM |
DNP |
9.1 × 103 |
13 μM |
DNT |
1.2 × 103 |
44 μM |
NP |
5.6 × 103 |
44 μM |
NBA |
2.1 × 103 |
25 μM |
Fluorescence method |
[CH3-dpb]2[Mg3(1,4-NDC)4(μ H2O)2(CH3OH)(H2O)]·1.5H2O |
PA |
2.8 × 104 |
— |
73 |
2,4-DNA |
0.8 × 104 |
— |
Fluorescence method |
Serine-functionalised NaYF4:Ce3+/Gd3+/Eu3+@NaGdF4:Tb3+ |
PA |
4.364 × 104 |
1.67 ppm |
40 |
p-NP |
1.337 × 104 |
4.57 ppm |
p-NT |
0.824 × 104 |
6.36 ppm |
Fluorescence method |
PEI-functionalised NaCeF4:Tb3+//Eu3+ |
PA |
2.25 × 105 M−1 |
1.32 ppm |
Present work |
p-NP |
1.8 × 105 M−1 |
2.88 ppm |
p-NA |
1.1 × 105 M−1 |
4.24 ppm |
p-NT |
0.88 × 105 M−1 |
5.59 ppm |
Table 2 Detection of heavy metal ions by various methods from different nanomaterials
Sensing method |
Materials |
Detection of heavy metal ions |
KSV (M−1) |
LOD |
Reference |
Electrochemical method |
RGO/NiWO4 nanocomposite |
Cd2+ |
— |
1.11 × 10−10 M |
74 |
Pb2+ |
2.04 × 10−10 M |
Cu2+ |
2.80 × 10−10 M |
Hg2+ |
3.05 × 10−10 M |
Fluorescence method |
[(CH3)2NH2]·[Tb(bptc)] |
Fe3+ |
— |
76.7 ppm |
75 |
Fluorescence method |
[CH3-dpb]2[Mg3(1,4-NDC)4(μ H2O)2(CH3OH)(H2O)]·1.5H2O |
Fe3+ |
1.12 × 104 |
— |
73 |
Cr3+ |
0.15 × 104 |
— |
Fluorescence method |
Water-soluble graphene oxide (GO) |
Fe2+ |
5.6 × 103 |
— |
76 |
Hg2+ |
2.1 × 103 |
Fluorescence method |
PEI-functionalised NaCeF4:Tb3+//Eu3+ |
Fe3+ |
3.8 × 104 |
1.39 ppm |
Present work |
Cr3+ |
2.6 × 104 |
2.58 ppm |
Cd2+ |
1.8 × 104 |
3.85 ppm |
Co2+ |
0.88 × 104 |
7.48 ppm |
Quenching mechanism of metal ions and nitro-aromatic analytes
The possible quenching mechanism for the detection of analytes is based on the donor–acceptor interaction between PEI-functionalized NaCeF4:Tb3+/Eu3+ (Eu-7%) nanophosphor with different concentrations of analytes (nitroaromatics and heavy metal ions). The electron deficient nitro groups on the nitro aromatics interact with the lone pairs of the amine group present on the nanophosphor. Consequently, charge transfer takes place between the electron donor and electron acceptor group resulting in formation of stable acid–base Jackson Meisenheimer complex having negative charge delocalized over the ring of nitro analytes.77 The stable σ-complex so formed consequently leads to luminescence quenching due to energy transfer from luminescence nanophosphor to analyte.78 The incessant decrease in the luminescence intensity induces ultimate detection of the nitroaromatic analytes. For the detection of heavy metal ions similar donor–acceptor charge transfer mechanism is considered to account for the luminescence quenching effect.79 The charge transfer occurs from the electron rich PEI functionalized nanophosphor having lone pair of electrons to the electron deficient metals ions having vacant orbitals. The charge migration between the luminophore to the acceptor result in the quenching of luminescence intensity and ultimate detection of heavy metal ions by the PEI doped nanophosphor. The above results thus, specify PEI functionalized nanophosphor as a good candidate for the exquisite detection of nitro aromatics as well as for the heavy metals ions.
Photoluminescence spectra of R-GO@PEI@NaCeF4:Tb3+/Eu3+ nanocomposite
Additionally, to unravel the impact of reduced graphene oxide on photoluminescence behavior and quenching phenomenon of the nanophosphor, the composites of R-GO and nanophosphor was investigated. Firstly, the photoluminescence spectrum of the RGO@NaCeF4:Tb3+/Eu3+ was recorded at room temperature at excitation wavelength of 394 nm as shown in Fig. 19. Complete quenching of luminescence of the nanocomposite was observed due to the strong quenching effect of reduced graphene oxide.80 Interestingly, the PL spectrum of RGO@PEI@NaCeF4:Tb3+/Eu3+ (Eu-7%) functionalized nanocomposite was recorded at excitation wavelength of 394 nm. It was interestingly observed that PL intensity of the nanophosphor recovers in the functionalized nanocomposite which was earlier quenched. The recovery of the PL intensity may be due to the presence of the PEI ligand which was capped around the nanophosphor surface. The PEI acts as bridging ligand which interconnects reduced graphene oxide and nanophosphor core. It was inferred that the PEI functionalized luminescent nanophosphor does not directly communicate with the graphene oxide. The quenching process taking place in the pure nanophosphor can be explained because of the direct interaction of core material with the RGO sheets leading to energy transfer and ultimate quenching of the PL in nanophosphor. On the other hand in the functionalized nanocomposite the PEI@NaCeF4:Tb3+/Eu3+ (Eu-7%) complex is anchored on the RGO via the electrostatic interaction as well as hydrogen bonding between the NH2 group of PEI and the functional moieties of RGO. This interaction keeps the core materials at far distance from the oxygen functionalizing group and thus reducing the quenching effect.81 It may be assumed that firstly, there occur strong direct interactions between oxygen functionality of RGO and core materials leading to quenching of the luminescence intensity. However, when the PEI was used as the additional ligand, the NH2 group present on the PEI molecule strongly interacts with the different functional moieties present on the negatively charged RGO sheets thus weakening the interaction between core materials and ultimately inhibiting quenching leading to recovery of the PL in nanophosphor.
In conclusion, the self assembly of the nanophosphor with the RGO not only achieves recovery in the photoluminescence intensity but there occur red PL emission from the NaCeF4:Tb3+/Eu3+ as shown in Fig. 19. The red emission of the nanocomposite was confirmed by the presence of highly intense peak at 624 nm occurring due to transition from 5D0 → 7F2 responsible for red emission in the PL spectrum.82 Thus, culminated that the binding of PEI as capping agent not only minimizes the quenching of luminescence intensity of nanophosphor but also results in the red emission of the luminescence nanocomposite providing it as a novel candidate for emerging applications as optical sensors.
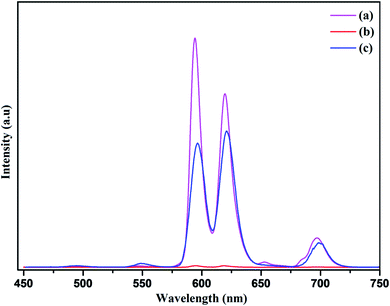 |
| Fig. 19 Photoluminescence emission spectra of (a) NaCeF4:Tb3+/Eu3+ (Eu-7%) nanophosphor (b) R-GO@NaCeF4:Tb3+/Eu3+ (Eu-7%) (c) R-GO@ PEI@NaCeF4:Tb3+/Eu3+ (Eu-7%). | |
Conclusions
In summary, we have elaborately designed a highly selective and rapid luminescence nanosensor that can effectively detect the toxic analytes in the waste water. The presence of amine groups on the surface of PEI functionalized NaCeF4:Tb3+/Eu3+ nanostructure enhance the efficiency of the detection of heavy metal ions and nitroaromatic compounds extensively. Additionally, with increased amounts of heavy metal ions and nitroaromatics, the luminescence emission of the fabricated nanoparticles is exponentially quenched. The quenching efficiency for the Fe3+ ion and PA are found to be 90.02% and 97.05% respectively. Furthermore, KSV follows the order of (Fe3+ > Cr3+ > Co2+ > Cd2+ with highest KSV value for Fe3+) and for aromatic nitro compound as (PA > p-NP > p-NA > 4-NT). The data suggests high selectivity of novel PEI functionalizedNaCeF4:Tb3+/Eu3+ nanophosphor for the detection of Fe3+ and picric acid (PA).
Author contributions
Richa Singhaal: conceptualization, formal analysis, investigation & writing original draft. Lobzang Tashi: methodology and writing. Zaib ul Nisa: data curation & writing. Nargis Akhter Ashashi: software, review & editing. Charanjeet Sen and Swaita Devi: resources & validation. Haq Nawaz Sheikh: project administration & supervision.
Conflicts of interest
The authors declare no competing financial interest.
Acknowledgements
We acknowledge financial support from DST-PURSE-2 for photoluminescence facility. R. S acknowledges the support from Council of Scientific and Industrial Research New Delhi India (reference no. 09/100(0233)/2019-EMR-I) for JRF Fellowship. The authors also acknowledge the support from Advanced Material Research Centre (AMRC), IIT Mandi for FE-SEM analysis, Sophisticated Analytical Instrumentation Facility (SAIF), IIT Bombay for TEM and HR-TEM analysis and Sophisticated Analytical Instrumentation Facility (SAIF) Panjab University for PXRD and EDS.
References
- C. Li, Z. Hou, Y. Dai, D. Yang, Z. Cheng and J. Lin, Biomater, 2013, 1, 213–223 RSC.
- D. Wang, T. Xie and Y. Li, Nano Res., 2009, 2, 30–46 CrossRef CAS.
- G. Wang, Q. Peng and Y. Li, Acc. Chem. Res., 2011, 44, 322–332 CrossRef CAS PubMed.
- W. Abbas, I. Ahmad, M. Kanwal, G. Murtaza, I. Ali, M. A. Khan, M. N. Akhtar and M. Ahmad, J. Magn. Magn. Mater., 2015, 374, 187–191 CrossRef CAS.
- G. Chen, T. Y. Ohulchanskyy, S. Liu, W. C. Law, F. Wu, M. T. Swihart, H. Ågren and P. N. Prasad, ACS Nano, 2012, 6, 2969–2977 CrossRef CAS PubMed.
- L. Tashi, M. Kumar, Z. Nisa, N. Nelofar and H. N. Sheikh, New J. Chem., 2020, 44, 1009–1020 RSC.
- S. Sarkar, M. Chatti, V. NKB Adusumalli and V. Mahalingam, ACS Appl. Mater. Interfaces, 2015, 7, 25702–25708 CrossRef CAS PubMed.
- R. Tu, B. Liu, Z. Wang, D. Gao, F. Wang, Q. Fang and Z. Zhang, Anal. Chem., 2008, 80, 3458–3465 CrossRef CAS PubMed.
- A. Efligenir, S. Déon, P. Fievet, C. Druart, N. M. Crini and G. Crini, Chem. Eng. J., 2014, 258, 309–319 CrossRef CAS.
- Y. Guan, C. Shao and M. Ju, Int. J. Environ. Res. Public Health, 2014, 11, 7286–7303 CrossRef CAS PubMed.
- Y. Zou, X. Wang, A. Khan, P. Wang, Y. Liu, A. Alsaedi, T. Hayat and X. Wang, Environ. Sci. Technol., 2016, 50, 7290–7304 CrossRef CAS PubMed.
- A. Sepúlveda, M. Schluep, F. G. Renaud, M. Streicher, R. Kuehr, C. Hagelüken and A. C. Gerecke, Environ. Impact Assess. Rev., 2010, 30, 28–41 CrossRef.
- H. K. Vardhan, P. S. Kumar and R. C. Panda, J. Mol. Liq., 2019, 290, 111197 CrossRef.
- A. B. Lansdown, Met Ions Life Sci, 2011, 8, 187–246 CAS.
- S. Lambert and M. Wagner, Chem. Soc. Rev., 2017, 46, 6855–6871 RSC.
- J. S. Becker, U. Breuer, H. F. Hsieh, T. Osterholt, U. Kumtabtim, B. Wu, A. Matusch, J. A. Caruso and Z. Qin, Anal. Chem., 2010, 82, 9528–9533 CrossRef CAS PubMed.
- Y. Lei, F. Zhang, P. Guan, P. Guo and G. Wang, New J. Chem., 2020, 44, 14299–14305 RSC.
- J. Akhoundzadeh, M. Chamsaz, S. R. Yazdinezhad and M. H. Arbaz-zavvar, Anal. Methods, 2013, 5, 778–783 RSC.
- M. Najarro, M. E. D. Morris, M. E. Staymates, z. R. Fletcher and G. Gillen, Analyst, 2012, 137, 2614–2622 RSC.
- I. Bruzas, W. Lum, Z. Gorunmez and L. Sagle, Analyst, 2018, 143, 3990–4008 RSC.
- B. C. Blount, K. E. Milgram, M. J. Silva, N. A. Malek, J. A. Reidy, L. L. Needham and J. W. Brock, Anal. Chem., 2000, 72, 4127–4134 CrossRef CAS PubMed.
- J. L. Bricks, A. Kovalchuk, C. Trieflinger, M. Nofz, M. Büschel, A. I. Tolmachev, J. Daub and K. Rurack, J. Am. Chem. Soc., 2005, 127, 13522–13529 CrossRef CAS PubMed.
- M. G. Skalnaya and A. V. Skalny, Essential trace elements in human health: a physician's view, Publishing House of Tomsk State University, Tomsk, 2018, p. 224 Search PubMed.
- E. Grunwald and E. Price, J. Am. Chem. Soc., 1964, 86, 4517–4525 CrossRef CAS.
- J. A. Young, J. Chem. Edu., 2008, 85, 492 CrossRef CAS.
- W. Feng and X. Liu, Chem. Soc. Rev., 2009, 38, 976–989 RSC.
- W. Zheng, P. Huang, D. Tu, E. Ma, H. Zhu and X. Chen, Chem. Soc. Rev., 2015, 44, 1379–1415 RSC.
- B. Huang, H. Dong, K. L. Wong, L. D. Sun and C. H. Yan, J. Phys. Chem., 2016, 120, 18858–18870 CAS.
- J. Zhou, J. L. Leano, Z. Liu, D. Jinn, K. L. Wong, R. S. Liu and J. G. Bunzli, Small, 2018, 14(1–29), 1801882 CrossRef PubMed.
- D. Hudry, I. A. Howard, R. Popescu and D. Gerthsen, Adv. Mater., 2019, 31, 1900623 CrossRef PubMed.
- X. Liu and D. Yuan, Sci. Rep., 2015, 5, 1–13 Search PubMed.
- R. Naccache, Q. Yu and J. A. Capobianco, Adv. Opt. Mater., 2015, 3, 482–509 CrossRef CAS.
- J. L. Mason, H. Hassan, J. E. Topolski, H. P. Hratchian and C. C. Jarrold, Acc. Chem. Res., 2019, 52, 3265–3273 CrossRef CAS PubMed.
- M. Runowski, A. Shyichuk, A. Tymiński, T. Grzyb, V. Lavín and S. Lis, ACS Appl. Mater. Interface, 2018, 10, 17269–17279 CrossRef CAS PubMed.
- O. J. Sovers and T. Yoshioka, J. Chem. Phys., 1969, 51, 5330–5336 CrossRef CAS.
- H. E. Townley, Curr. Nanosci., 2013, 9, 686–691 CrossRef CAS.
- M. Runowski, A. Bartkowiak, M. Majewska, I. R. Martín and S. Lis, J. Lumin., 2018, 201, 104–109 CrossRef CAS.
- C. Yan, H. Zhao, D. F. Perepichka and F. Rosei, Small, 2016, 12, 3888–3907 CrossRef CAS PubMed.
- M. Malik, P. Padhye and P. Poddar, ACS Omega, 2019, 4, 4259–4268 CrossRef CAS PubMed.
- L. Tashi, R. Singhaal, M. Kumar and H. N. Sheikh, New J. Chem., 2020, 44, 19908–19923 RSC.
- W. Abbas, N. Akhtar, Q. Liu, T. Li, I. Zada, L. Yao, R. Naz, W. Zang, M. E. Mazhan, D. Zhang, D. Ma and J. Gu, Sens. Actuators, B, 2019, 282, 617–625 CrossRef CAS.
- A. S. Saleemi, M. Hafeez, A. Munawar, N. Akhtar, W. Abbas, M. E. Mazhar, Z. Shafiq, A. P. Davis and S. L. Lee, J. Mater. Chem. C, 2020, 8, 12984–12992 RSC.
- W. Abbas, Q. Liu, N. Akhtar, J. Ahmad, M. E. Mazhar, T. Li, I. Zada, L. Yao, R. Naz, M. Imtiaz, W. Zhang, A. Amjad, D. Zhang and J. Gu, J. Electroanal. Chem., 2019, 853, 113560–113598 CrossRef CAS.
- P. K. Sarkar, N. Polley, S. Chakrabarti, P. Lemmens and S. K. Pal, ACS Sens, 2016, 1, 789–797 CrossRef CAS.
- L. Shahriary and A. A. Athawale, Int. J. Energy Environ. Eng., 2014, 2, 58–63 Search PubMed.
- S. Park, J. An, J. R. Potts, A. Velamakanni, S. Murali and R. S. Ruoff, Carbon, 2011, 49, 3019–3023 CrossRef CAS.
- J. Ladol, H. Khajuria, R. Singh, V. Kumar and H. N. Sheikh, J. Mater. Sci.: Mater. Electron., 2017, 28, 11671–11681 CrossRef CAS.
- H. Lian, Y. Dai, D. Yang, Z. Cheng, C. Li, Z. Hou, M. Shang and J. Lin, Nanoscale, 2014, 6, 9703–9712 RSC.
- S. Calvin, S. X. Luo, C. C. Broadbridge, J. K. McGuinness, E. Anderson, A. Lehman, K. H. Wee, S. A. Morrison and L. K. Kurihara, Appl. Phys. Lett., 2005, 87, 233102 CrossRef.
- K. Huang, M. Lei, Y. J. Wang, C. Liang, C. X. Ye, X. S. Zhao, Y. F. Li, R. Zhang, D. Y. Fan and Y. G. Wang, Powder Diffr., 2014, 29, 8–13 CrossRef CAS.
- X. Qu, H. K. Yang, G. Pan, J. W. Chung, B. K. Moon, B. C. Choi and J. H. Jeong, Inorg. Chem., 2011, 50, 3387–3393 CrossRef CAS PubMed.
- X. Lei, R. Li, D. Tu, X. Shang, Y. Liu, W. You, C. Sun, F. Zhang and X. Chen, Chem. Sci., 2018, 9, 4682–4688 RSC.
- X. Zhong, X. Wang, G. Zhan, Y. Tang, Y. Yao, Z. Dong, L. Hou, H. Zhao, S. Zeng, J. Hu and L. Cheng, Nano Lett., 2019, 19, 8234–8244 CrossRef CAS PubMed.
- T. Ji, P. Fan, X. Li, Z. Mei, Y. Mao and Y. Tian, RSC Adv., 2019, 9, 10645–10650 RSC.
- Y. C. Chen, Y. C. Wu, D. Y. Wang and T. M. Chen, J. Mater. Chem. A, 2012, 16, 7961–7969 RSC.
- H. Cai, X. An, J. Cui, J. Li, S. Wen, K. Li, M. Shen, L. Zheng, G. Zhang and X. Shi, ACS Appl. Mater. Interfaces, 2013, 5, 1722–1731 CrossRef CAS PubMed.
- D. R. Dreyer, S. Park, C. W. Bielawski and R. S. Ruoff, Chem. Soc. Rev., 2010, 39, 228–240 RSC.
- P. G. Ren, D. X. Yan, X. Ji, T. Chen and Z. M. Li, Nanotechnology, 2011, 22, 55705 CrossRef PubMed.
- X. Qu, H. K. Yang, G. Pan, J. W. Chung, B. K. Moon, B. C. Choi and J. H. Jeong, Inorg. Chem., 2011, 50, 3387–3393 CrossRef CAS PubMed.
- S. Fischer and T. Jüstel, J. Lumin., 2020, 223, 117232 CrossRef CAS.
- M. Shang, S. Liang, H. Lian and J. Lin, Inorg. Chem., 2017, 56, 6131–6140 CrossRef CAS PubMed.
- F. N. Sayed, V. Grover, S. V. Godbole and A. K. Tyagi, RSC Adv., 2012, 2, 1161–1167 RSC.
- J. Huo, L. Dong, W. Lü, B. Shao and H. You, Phys. Chem. Chem. Phys., 2017, 19, 17314–17323 RSC.
- H. Guan, G. Liu, J. Wang, X. Dong and W. Yu, New J. Chem., 2014, 38, 4901–4907 RSC.
- S. Gai, C. Li, P. Yang and J. Lin, Chem. Rev., 2014, 114, 2343–2389 CrossRef CAS PubMed.
- Z. u. Nisa, L. Tashi, C. Sen, N. A. Ashashi, S. C. Sahoo and H. N. Sheikh, New J. Chem., 2020, 44, 8125–8137 RSC.
- S. James, B. Chishti, S. A. Ansari, O. Y. Alothman, H. Fouad, Z. A. Ansari and S. G. Ansari, J. Electron. Mater., 2018, 47, 7505–7513 CrossRef CAS.
- L. Chu, L. Han and X. Zhang, J. Appl. Electrochem., 2011, 41, 687–694 CrossRef CAS.
- V. Prchal, V. Vyskocil and J. Barek, J. Electroche. Soc., 2010, 164, H316–H320 CrossRef.
- H. Namgung, J. J. Lee, Y. J. Gwon and T. S. Lee, RSC Adv., 2018, 8, 34291–34296 RSC.
- L. H. Cao, F. Shi, W. M. Zhang, S. Q. Zang and T. C. W. Mak, Chem.–Eur. J., 2015, 21, 15705–15712 CrossRef CAS PubMed.
- W. Dong, Q. Ma, Z. Ma, Q. Duan, X. Lü, N. Qiu and Z. Su, Dyes Pigm., 2020, 172, 107799 CrossRef CAS.
- Z. F. Wu, L. K. Gong and X. Y. Huang, Inorg. Chem., 2017, 56, 7397–7403 CrossRef CAS PubMed.
- R. Kumar, T. Bhuvana and A. Sharma, RSC Adv., 2017, 7, 42146–42158 RSC.
- X. L. Zhao, D. Tian, Q. Gao, H. W. Sun, J. Xu and X. H. Bu, Dalton Trans., 2016, 45, 1040–1046 RSC.
- D. Y. Wang, D. W. Wang, H. A. Chen, T. R. Chen, S. S. Li, Y. C. Yeh, T. R. Kuo, J. H. Liao, Y. C. Chang, W. T. Chen and S. H. Wu, Carbon, 2015, 82, 24–30 CrossRef CAS.
- T. Das, A. Pramanik and D. Haldar, Sci. Rep., 2017, 7, 1–12 CrossRef PubMed.
- S. Sarkar, L. G. Suárez, W. Verboom, V. Mahalingam and J. Huskens, ChemNanoMat, 2016, 2, 805–809 CrossRef CAS.
- M. Li, H. Gou, I. A. Ogaidi and N. Wu, ACS Sustain. Chem. Eng., 2013, 1, 713–723 CrossRef CAS.
- M. C. Hsiao, S. H. Liao, M. Y. Yen, P. I. Liu, N. W. Pu, C. A. Wang and C.
C. M. Ma, ACS Appl. Mater. Interfaces, 2010, 2, 3092–3099 CrossRef CAS PubMed.
- X. Zhang, W. Zhang, Y. Li and C. Li, Dyes Pigm., 2017, 140, 150–156 CrossRef CAS.
- C. Li, Z. Quan, J. Yang, P. Yang and J. Lin, Inorg. Chem., 2007, 46, 6329–6337 CrossRef CAS PubMed.
Footnote |
† Electronic supplementary information (ESI) available. See DOI: 10.1039/d1ra02910j |
|
This journal is © The Royal Society of Chemistry 2021 |
Click here to see how this site uses Cookies. View our privacy policy here.