DOI:
10.1039/D1RA02827H
(Paper)
RSC Adv., 2021,
11, 23550-23556
Retracted Article: In situ Raman investigation of the phase transition of NaVO2F2 under variable temperature conditions
Received
12th April 2021
, Accepted 19th June 2021
First published on 5th July 2021
Abstract
In this study, the phase transition of NaVO2F2 was measured at different temperatures via in situ Raman spectroscopy. The NaVO2F2 compounds were synthesized by a hydrothermal method and were identified to be monoclinic with the P21/c space group at room temperature by XRD. Accordingly, the variations of Raman shifts and intensities of the characteristic peaks for NaVO2F2 associated with temperature were obtained and investigated. It was confirmed that NaVO2F2 had three types of phase transitions, which occurred in the temperature region from 78 K to 573 K. Further, the results indicate that transition from a low-temperature phase (I) to another low-temperature phase (II), low-temperature phase (II) to P21/c phase and P21/c phase to P21/m phase occurred near the three temperature points of 93 K, 233 K, and 453 K, respectively, during the heating process. Therefore, a novel characterization method was provided for further research on the phase transition theory and performance of vanadate compounds.
1 Introduction
In recent years, vanadate compounds have received widespread attention due to their low synthesis temperature, stable chemical properties and good thermal stability.1,2 Metal vanadate nanomaterials are obviously different from traditional bulk materials, which have a broad application prospect in energy storage and conversion, catalysis, photoelectricity and magnetic fields.3–7 As a type of metal vanadate, NaVO2F2 is mainly used in the preparation of fluorescent materials, laser materials and rechargeable lithium battery cathode materials, so researching the structure of NaVO2F2 has great significance because the prerequisite for understanding material properties is to grasp the structure. This is accompanied by the difference in the microstructure of the material before and after the phase change, which makes the material change to a large extent in terms of physical and chemical properties, thereby determining the application range of the material. Currently, the detection of the phase change by Raman spectroscopy has been extensively used in numerous industries. For example, Raman spectroscopy is used to analyze the structural changes of substances after doping elements.8–10 Furthermore, Raman spectroscopy is also used in medical systems to detect cancer, diseases related to the human nervous system and other diseases.11–15 However, in situ Raman spectroscopy can more accurately characterize the transformation law of the structural characteristics of the objects. Numerous scholars have used the in situ Raman method to investigate the phase transition process of ceramics, crystals and methane hydrates under different environmental conditions.16–19
Investigating the phase transition of NaVO2F2 monocrystalline–monocrystalline transformation is of great significance to further study the relationship between the structure and properties of this crystal. NaVO2F2 is a single crystal with very special properties and the report of the phase transition is scarce. There are two reasons: on the one hand, since its phase transition is not as rapid and obvious as that of common substances, such as diamond and iron; in addition, the phase transition of NaVO2F2 is a gradual process with the increase in temperature that the bond lengths of some chemical bonds change gradually. The phase transition temperature is a range rather than a temperature point. On the other hand, the [VF2O2] octahedron is different from the common fluoro-oxygen tetrahedral structure, which can be seen everywhere; furthermore, the component elements of the [VF2O2] octahedron are very simple without any signal interference from other elements; thus, its research results are more representative and can be studied as a standardized substance.
Up to now, some researchers assessed the phase structure of NaVO2F2 from low temperature to high temperature by DSC, DTA and XRD experimental methods,20–24 proving that this compound has the P21/c phase with a symmetric center and the P21 phase with an asymmetric center simultaneously. Yu23 proved by XRD that NaVO2F2 has four crystal phases, namely P21(I), P21/m(II), P21/c(III) and another low temperature phase. The first principles calculations demonstrate that the substructures of P21(I) and P21/c(III) were more stable than the substructure of P21/m(II). Moreover, the substructure of P21/c(III) was the most stable of three structures. DSC tests show that the compound will undergo more than one first-order phase transition, when the temperature is lower than 139 K. However, the single crystal data under low temperatures failed to be analyzed due to the intensity error of the diffraction point caused by the occurrence of twin crystals.23 DTA tests show that one or two transitions occur at low temperatures, but the temperature range cannot be accurately measured.23 In addition, a few XRD consequences proclaimed that there is a reversible phase transition of NaVO2F2 at high temperatures.21–23 However, although the examination of the crystal structure of NaVO2F2 has been carried out via different methods, these are limited only to theoretical speculation or ex situ experiments, and the in situ measurements of the crystal structure and phase transformation for NaVO2F2 are still lacking.
Raman spectroscopy has the advantages of in situ and nondestructive characteristics for observing the phase transition. Particularly for single crystal samples, Raman spectroscopy can easily distinguish the phase structures corresponding to different optical films. So far, there is no report about the effects of temperature on the phase transition of the NaVO2F2 single crystal structure via in situ Raman spectroscopy. In this study, for the first time, the in situ Raman spectroscopy was used to observe and study the NaVO2F2 phase transformation process with the gradual increase in temperature from 78 K to 573 K; therefore, a new test method was created to confirm that NaVO2F2 has different phase transitions at different temperatures.
2 Experimental details
2.1 Materials and synthesis of NaVO2F2
Various hydrothermal methods to synthesize vanadates have been reported after synthesizing the first borovanadate by the hydrothermal method in 1997.25 Moreover, our approach for the synthesis of NaVO2F2 single crystals also by a hydrothermal method has been reported.23 Briefly, the description of materials and the synthetic procedure is shown in Fig. 1.
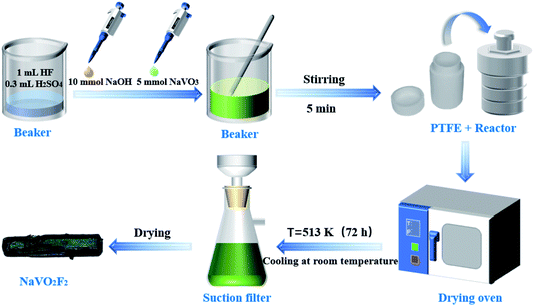 |
| Fig. 1 Schematic of the process for preparing NaVO2F2. | |
The reactants including 10 mmol NaOH and 5 mmol NaVO3 were added to a beaker, which contained 1 mL HF (40% mass fraction formulation to 20 mmol) and 0.3 mL H2SO4 (98% mass fraction formulation to 5.5 mmol). Next, the mixture was transferred to the reactor, which had a PTFE liner immediately after stirring for 5 min. Then, we put the reactor into an oven maintaining at 513 K for 72 h; afterwards, it was cooled at room temperature. Finally, the product was filtered, and dried to obtain dark green crystals.
2.2 Characterization
The details of the XRD measurements and analytical procedures performed were the following. The X-ray diffraction (XRD, Bruker-D8-A25) of the powder sample was carried out on a Rigaku D/Max-RC Target X ray diffractometer from the Neo-Confucianism Company of Japan. The experimental conditions for the determination of the initial crystal structure were as follows: Cu Kα radiation (λ = 0.15405 nm), and working voltage and working current were 40 kV and 40 mA, respectively, and the scanning step and scanning speed were 0.02° per step and 6° min−1, and the scanning range was 10° ≤ 2θ ≤ 90°. The morphology of the samples was inspected using a scanning electron microscope (SEM, Hitachi, SU-70).
The in situ microscopic Raman spectroscopic tests of the specimens were performed using a TriVista CRS557 three-stage confocal microscopy Raman spectrometer produced by Princeton Instruments with a 532 nm laser of 100 MW power. The exposure time was 10 s, and five sampling times were accumulated in order to improve the signal-to-noise ratio (SNR) of the spectrum. The temperature change was controlled by a THMS600 high and low-temperature sample stand produced by LINKAM, which can be changed from a low temperature of 78 K to a high temperature of 873 K; moreover, the temperature fluctuation could be controlled within the range of 0.1 K. In this study, the test temperature range was 78–573 K, and the heating rate was 20 K min−1. The Raman spectra obtained were each at 20 K after 2 min of stabilization at each temperature. The peaks were fitted according to the standard procedure reported widely in the literature.26,27 Succinctly, the Raman spectrum was fitted by the superposition of Lorentzian functions according to the following equation:28
|
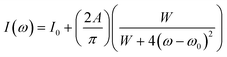 | (1) |
where
ω is the phonon frequency of the peak,
I0 is the intensity of the background,
A is the normalization constant,
W is the full width at half maxima (FWHM), and
ω0 is the maximum phonon frequency of the peak, respectively.
3 Results and discussion
3.1 Structure and composition of NaVO2F2
The NaVO2F2 single crystal was obtained according to the above hydrothermal method; furthermore, diffraction lines, as shown in Fig. 2(a), were collected by the powder diffraction method. It is well known that the properties of the compounds depend on the structure, and the corresponding data can be obtained by searching the spectra that have already existed in the database.
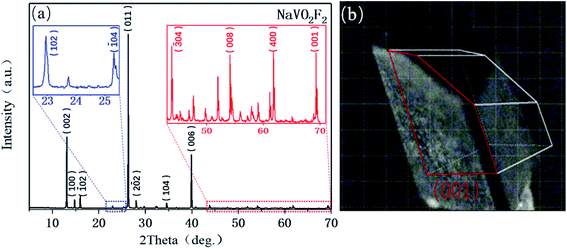 |
| Fig. 2 (a) XRD profiles and (b) (001) plane of NaVO2F2. | |
The analysis and refinement of the full matrix structure were completed by the direct method and least-square method, respectively, using the SHELX97 program. The specific results obtained by refinement were as follows: measurement results of the crystal structure and parameters: NaVO2F2 is a monoclinic crystal system, P21/c of the space group, a = 6.3977(10), b = 3.5168(10), c = 14.4409(17), β (°) = 110.275(15), V (Å3) = 304.78, Z = 4, R1 = 0.026, wR2 = 0.079 after revised. Fig. 2(b) represents that the largest crystal plane is the (001) plane, where the light source is also incident from the (001) plane during the in situ Raman test.
It can be observed that the appearance of NaVO2F2 is a lath crystal with a uniform size and good crystallinity, as shown in Fig. 3(a). In addition, it can be demonstrated that the compound contained Na, V, O, and F elements according to Fig. 3(b), and the atomic percentage of oxygen is about twice that of sodium and vanadium. Moreover, the element percentage shows that the number of atoms about sodium and vanadium is almost the same in spite of the low content of fluorine due to the particularity of its elements. Overall, the ratio of these elements correspond to the structural characteristics of the crystal.
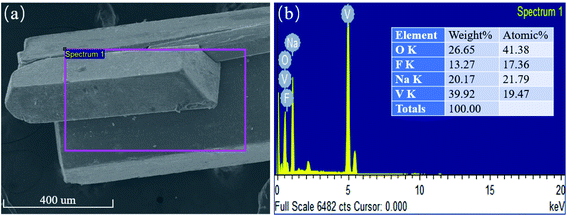 |
| Fig. 3 (a) Microstructure and (b) EDS analysis of the NaVO2F2 sample. | |
3.2 Analysis of the Raman spectral shift and phase transitions
Fig. 4 presents the characteristic in situ Raman spectra from a low temperature (78 K) to a high temperature (573 K) for the single crystal of NaVO2F2. In this study, the shift due to anharmonicity with temperature is usually much less; therefore, the influence of this part is not considered. It is found that the peak shape, peak intensity and peak position at frequencies of 300, 440, 730 and 1010 cm−1 were changed obviously during heating process from 78 K to 573 K, where the characteristic peaks were denoted as 1, 2, 3 and 4 for the next analysis conveniently. In order to further analyze, the different peaks of the obtained Raman spectrum were fitted and investigated for the purpose of obtaining the Raman shift of each characteristic peak in the Raman spectrum. The data points of Raman shift are the average values of three points taken at the corresponding temperature, and the error range of each three points ranged from 1–2 cm−1. The graph of the Raman shift curve diagram for each characteristic peak along with the temperature is shown in Fig. 5.
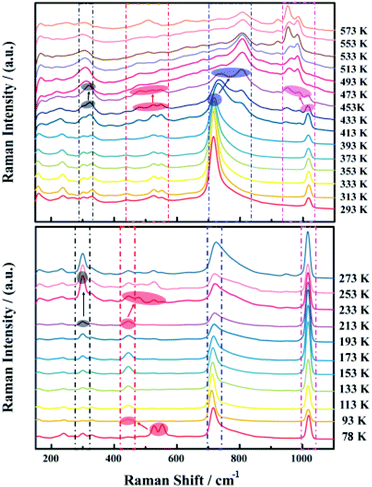 |
| Fig. 4 In situ Raman spectra of NaVO2F2. (a) Below 273 K and (b) above 273 K. | |
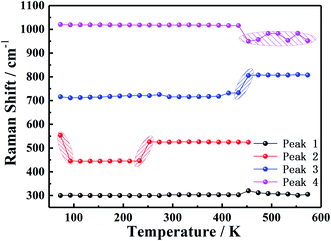 |
| Fig. 5 Raman shift of each characteristic peak varies with temperature. | |
The variation of the characteristic peaks in the Raman spectrum indicates the corresponding changes of lattice vibration and structural symmetry. More importantly, the vibration mode of NaVO2F2 is mainly attributed to the vibration of the VO6 octahedron. The vibrational spectra of simple vanadate-containing vanadium minerals show that the corresponding relationship between the vibration of the VO6 octahedron and the Raman spectra are as follows:29–32 the region of 300–400 cm−1 was the counterpart of the V–O bending vibration, the peak at 500 cm−1 corresponds to the V–O–V symmetric stretching vibrations of the bridge bond, the peak at 700 cm−1 corresponds to the V–O–V antisymmetric stretching vibration of the bridge bond and the area of 900–1000 cm−1 corresponds to the V
O terminal connection bond, respectively. It can be inferred that the characteristic peak 1 at 300 cm−1 corresponds to the V–O bending vibration, the characteristic peak 2 at 553 cm−1 is the counterpart of the symmetric stretching vibration of the V–O–V bridge bond, the characteristic peak 3 at 715 cm−1 corresponds to the antisymmetric stretching vibration of the V–O–V bridge bond and the characteristic peak 4 at 1020 cm−1 corresponds to the V
O terminal connection bond as compared to the in situ Raman spectrum of NaVO2F2 at 78 K. In addition, in order to distinguish the types of bonds more intuitively, the molecular structure diagram in Fig. 6 is drawn using the diamond software, and the molecular structure diagram and morphology of NaVO2F2 (P21/c) at 295 K are shown in Fig. 6.
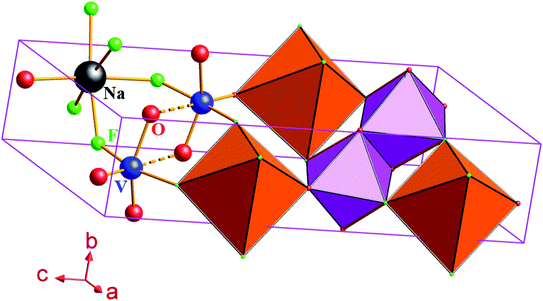 |
| Fig. 6 Molecular structure of NaVO2F2 at 295 K. | |
We listed the changes in the Raman spectrum for the NaVO2F2 single crystal in the temperature variation region from 78 K to 573 K in Table 1 based on the above analysis and Fig. 5, and the following phenomena can be seen.
Table 1 In situ Raman transformation of characteristic peaks at different temperatures for NaVO2F2 (RS is Raman shift, while RI is Raman intensity)
Characteristic peaks |
1 |
2 |
3 |
4 |
Raman shift |
300 cm−1 |
440 cm−1 |
730 cm−1 |
1010 cm−1 |
Type of bond |
V–O |
V–O–V |
V–O–V |
V O |
93(RI) |
|
Double to single |
|
|
93(RS) |
|
553 cm−1 to 445 cm−1 |
|
|
233 K(RI) |
Peak sharped and increased |
Peak split |
|
|
233 K(RS) |
|
445 cm−1 to 526 cm−1 |
|
|
453 K(RI) |
Two small peaks merged |
Peak intensity decreased |
Peak split |
Peak split |
453 K(RS) |
|
|
733 cm−1 to 805 cm−1 |
1015 cm−1 to 950 cm−1 |
At a low temperature of 93 K, the characteristic peak 2 merged from double peaks into a single peak and accompanied by a Raman shift decrease from 553 cm−1 to 445 cm−1, which indicates that the mode of the V–O–V symmetric stretching vibration of the bridge bond has changed, and led the symmetry of the crystal structure to be transformed concurrently. This is also consistent with the DSC test results reported by Yu,23 where a first-order phase transition occurred when the temperature was lower than 139 K.
The Raman intensity of the characteristic peak 2 weakened and dispersed from one peak to several small peaks, while the Raman shift increased from 445 cm−1 to 526 cm−1 when the temperature at 233 K, which manifested the mode of the V–O–V symmetric stretching vibration of the bridge bond and the symmetry of the crystal structure both altered. However, on the basis of the single crystal diffraction data measured in literature,23 it was confirmed that the P21/c phase existed in the temperature region of 295 K; in this case, this mutation of the Raman shift and Raman intensity probably corresponds to the transformation from a low temperature phase to the P21/c phase, which was not observed by any previous researcher.23
When the high temperature was 453 K, the characteristic peak 3 split from a single peak into two small broad peaks, and the Raman shift increased from 733 cm−1 to 805 cm−1 simultaneously. It is shown that the V–O–V antisymmetric stretching vibration of the bridge bond transformed, leading to the change in the symmetry of the crystal structure. Moreover, the characteristic peak 4 from a single peak separated into three smaller peaks suddenly, and the Raman shift decreased from 1015 cm−1 to 950 cm−1 simultaneously, indicating that the mode of the V
O terminal connection bond and the symmetry of the crystal structure all changed. This is the same temperature at which the P21/c phase transformed into a new phase P21/m reported in the literature,23 so it could be considered that the phase change at this temperature corresponded to the transition from the P21/c phase to the P21/m phase. This means that the P21/c phase and P21/m phase are distinguished in the variable temperature in situ Raman spectrum, and the existence of the P21/c phase to P21/m phase transition was confirmed.
4 Conclusion
The occurrence of phase transition for NaVO2F2 is often excited by ambient temperature along with a special gradual process. The expected microstructure and structure can be obtained by controlling the different modes of phase transitions at different temperatures, so that the potential of the material can be fully exploited. Therefore, it is very significant to determine the phase transition temperature. In the present study, the shift and peak intensity of Raman characteristic spectra for the NaVO2F2 crystal change with temperature were analyzed by observing the in situ Raman spectra, creating a new observation and analysis method for the phase transition of the NaVO2F2 crystal. The results indicated that different phase transitions occurred near the temperature points of 93 K, 233 K and 453 K during the heating process, and it was confirmed that the transition from the P21/c phase to the P21/m phase took place near the temperature point of 453 K, which provided a novel characterization method of the theoretical research based on phase transitions of NaVO2F2.
Author contributions
Sa Zhang: Data curation. Yan Li: Writing-original draft. Liuqing Huang: Software. Liuying Huang: Project administration. Xuetao Luo: Resources, Project administration.
Conflicts of interest
The authors declared no potential conflicts of interest with respect to the research, authorship and/or publication of the article.
Funding
The authors gratefully acknowledge the support from the National Natural Science Foundation of China (51904265).
Acknowledgements
The authors would like to thank Prof. Jinxiao Mi of Department of Materials Science and Engineering of Xiamen University for his assistance with the crystal structure of NaVO2F2.
References
- H. Kaur and M. Jayasimhadri, Color tunable photoluminescence properties in Eu3+ doped calcium bismuth vanadate phosphors for luminescent devices, Ceram. Int., 2019, 45(12), 15385–15393 CrossRef CAS.
- M. Yu, J. lin and J. Fang, Silica Spheres Coated with YVO4:Eu3+ Layers via Sol-Gel Process: A Simple Method To Obtain Spherical Core-Shell Phosphors, Chem. Mater., 2005, 17, 1783–1791 CrossRef CAS.
- S. Bach, A. Boudaoud, N. Emery, R. Baddour-Hadjean and J. P. Pereira-Ramos, l Li intercalation compound as positive electrode material for rechargeable lithium batteries, Electrochim. Acta, 2014, 119, 38–42 CrossRef CAS.
- R. Baddour-Hadjean, S. Bach, N. Emery and J. P. Pereira-Ramos, The peculiar structural behaviour of β-Na0.33V2O5 upon electrochemical lithium insertion, J. Mater. Chem., 2011, 21(30), 11296–11305 RSC.
- F. Cheng and J. Chen, Transition metal vanadium oxides and vanadate materials for lithium batteries, J. Mater. Chem., 2011, 21(27), 9841–9848 RSC.
- L. Deng, X. Niu, G. Ma and Z. Yang, et al., Layered Potassium Vanadate K0.5V2O5 as a Cathode Material for Nonaqueous Potassium Ion Batteries, Adv. Funct. Mater., 2018, 28(49), 1800670 CrossRef.
- H. Y. Xu, H. Wang and Z. Q. Song, et al., Novel chemical method for synthesis of LiV3O8 nanorods as cathode materials for lithium ion batteries, Electrochim. Acta, 2004, 49(2), 349–353 CrossRef CAS.
- C. E. Camayo, S. Gaona J. and C. F. V. Raigoza, Effect of La and Pr substitution on structure and magnetic properties of Pechini synthesized BiFeO3, J. Magn. Magn. Mater., 2021, 527, 167733 CrossRef CAS.
- J. de Lara Andrade, A. G. de Oliveira and L. S. Rodrigues, et al., Al2O3 nanoparticle polymorphs: effects of Zn2+ doping on the structural, optical and cytotoxic properties, Bull. Mater. Sci., 2021, 44, 23 CrossRef CAS.
- T. K. Pani and B. Sundaray, A correlation of lattice distortion with the magnetic properties of calcium doped bismuth ferrite thin films, J. Phys. D: Appl. Phys., 2021, 54(20), 205002 CrossRef CAS.
- L. A. Austin, S. Osseiran and C. L. Evans, Raman technologies in cancer diagnostics, Analyst, 2016, 141(2), 476–503 RSC.
- I. P. Santos, E. M. Barroso and T. C. Bakker Schut, et al., Raman spectroscopy for cancer detection and cancer surgery guidance: translation to the clinics, Analyst, 2017, 142(17), 3025–3047 RSC.
- T. D. Payne, A. S. Moody and A. L. Wood, et al., Raman spectroscopy and neuroscience: from fundamental understanding to disease diagnostics and imaging, Analyst, 2020, 145(10), 3461–3480 RSC.
- G. Donjuan-Loredo, R. Espinosa-Tanguma and F. Leon-Bejarano, et al., Raman Spectroscopy for Adipose Tissue Assessment in Rat Models of Obesity and Type 1 Diabetes, Appl. Spectrosc., 2021, 1–9 Search PubMed.
- M. Zong, L. Zhou and Q. Guan, et al., Comparison of Surface-Enhanced Raman Scattering Properties of Serum and Urine for the Detection of Chronic Kidney Disease in Patients, Appl. Spectrosc., 2020, 1–10 Search PubMed.
- H. Kadobayashi, H. Hirai and K. Suzuki, et al., Sequential in situ Raman spectroscopy for observing dissociation behavior of filled-ice Ih of methane hydrate at high pressure, J. Raman Spectrosc., 2020, 51(12), 2536–2542 CrossRef CAS.
- S. Miro, E. Bordas, L. Thomé and J. M. Costantini, et al., Monitoring of the microstructure of ion-irradiated nuclear ceramics by in situ Raman spectroscopy, J. Raman Spectrosc., 2016, 47(4), 476–485 CrossRef CAS.
- P. Pappas, E. Liarokapis and M. Calamiotou, et al., Magnetic interactions and the puzzling absence of any Raman mode in EuTiO3, J. Raman Spectrosc., 2021, 17(11), 1–11 Search PubMed.
- M. Choi, J. Son and H. Choi, et al., In-situ Raman spectroscopy of current-carrying graphene microbridge, J. Raman Spectrosc., 2014, 45(2), 168–172 CrossRef CAS.
- A. L. Spek, PLATON SQUEEZE: a tool for the calculation
of the disordered solvent contribution to the calculated structure factors”, Acta Crystallogr., Sect. C: Struct. Chem., 2015, 71(1), 9–18 CrossRef CAS.
- W. Sun, Y. X. Huang, Y. Pan and J. X. Mi, Investigation on pseudosymmetry, twinning and disorder in crystal structure determinations: Ba(H2O)M2III[PO3(OH)]4 (M=Fe, V) as examples, J. Solid State Chem., 2012, 187, 89–96 CrossRef CAS.
- W. Sun, Y. X. Huang and Z. Li, et al., Hydrothermal Synthesis and Single-Crystal X-Ray Structure Refinement of Three Borates: Sibirskite, Parasibirskite and Priceite, Can. Mineral., 2011, 49(3), 823–834 CrossRef CAS.
- Z. Q. Yu, J. Q. Wang and Y. X. Huang, et al., Polymorphism of NaVO2F2: a P2(1)/c superstructure with pseudosymmetry of P2(1)/m in the subcell, Acta Crystallogr., Sect. C: Struct. Chem., 2015, 71(6), 440–447 CrossRef CAS PubMed.
- B. C. Zhao, W. Sun and W. J. Ren, et al., Hygroscopic La[B5O8(OH)]NO3·2H2O: Insight into the evolution of borate fundamental building blocks, J. Solid State Chem., 2013, 206, 91–98 CrossRef CAS.
- J. T. Rijssenbeek, D. J. Rose and R. C. Haushalter, et al., Novel Clusters of Transition Metals and Main Group Oxides in the Alkylamine/Oxovanadium/Borate System, Angew. Chem., Int. Ed. Engl., 1997, 36(9), 1008–1010 CrossRef CAS.
- C. Y. Xu, P. X. Zhang and L. Yan, Blue shift of Raman peak from coated TiO2 nanoparticles, J. Raman Spectrosc., 2001, 32(10), 862–865 CrossRef CAS.
- S. M. Ansari, B. B. Sinha and D. Phase, et al., Particle Size, Morphology, and Chemical Composition Controlled CoFe2O4 Nanoparticles with Tunable Magnetic Properties via Oleic Acid Based Solvothermal Synthesis for Application in Electronic Devices, ACS Appl. Nano Mater., 2019, 2(4), 1828–1843 CrossRef CAS.
- S. Roy and C. V. Ramana, Effect of sintering temperature on the chemical bonding, electronic structure and electrical transport properties of β-Ga1.9Fe0.1O3 compounds, J. Mater. Sci., 2021, 67, 135–144 Search PubMed.
- R. L. Frost, M. Crane, P. A. Williams and J. Theo Kloprogge, Isomorphic substitution in vanadinite [Pb5(VO4)3Cl]-a Raman spectroscopic study, J. Raman Spectrosc., 2003, 34(3), 214–220 CrossRef CAS.
- R. L. Frost, K. L. Erickson, M. L. Weier and O. Carmody, Raman and infrared spectroscopy of selected vanadates, Spectrochim. Acta, Part A, 2005, 61(5), 829–834 CrossRef.
- R. L. Frost, P. A. Williams and W. Martens, et al., Raman spectroscopy of the basic copper phosphate minerals cornetite, libethenite, pseudomalachite, reichenbachite and ludjibaite, J. Raman Spectrosc., 2002, 33(4), 260–263 CrossRef CAS.
- R. L. Frost, P. A. Williams and W. Martens, et al., Raman spectroscopy of the minerals boléite, cumengéite, diaboléte and phosgenite-implications for the analysis of cosmetics of antiquity, Mineral. Mag., 2003, 67(1), 103–111 CrossRef CAS.
|
This journal is © The Royal Society of Chemistry 2021 |