DOI:
10.1039/D1RA02734D
(Paper)
RSC Adv., 2021,
11, 17346-17351
A new family of decanuclear Ln7Cr3 clusters exhibiting a magnetocaloric effect†
Received
8th April 2021
, Accepted 5th May 2021
First published on 12th May 2021
Abstract
Two dimeric Ln–Cr clusters with formula {Ln(H2O)8[Ln6Cr3(L)6(CH3COO)6(μ3-OH)12(H2O)12]}·(ClO4)6·xH2O (Ln = Gd, x = 35 for 1 and Ln = Dy, x = 45 for 2, HL = 2-pyrazinecarboxylic acid) were obtained by a ligand-controlled hydrolytic method with a mixed ligand system (2-pyrazinecarboxylic acid and acetate). Single crystal structure analysis showed that two trigonal bipyramids of [Gd3Cr2(μ3-OH)6]9+ worked as building blocks in constructing the metal-oxo cluster core of [Gd6Cr3(μ3-OH)12]15+ by sharing a common top – a Cr3+ ion. Additionally, compound 1 forms a three-dimensional framework with a one-dimensional nanopore channel along the a-axis through a hydrogen-bond interaction between the cationic cluster core and the free mononuclear cation [Gd(H2O)8]3+ and the π-bond interactions of the pyrazine groups on the two cationic cluster cores. Magnetic calculations indicated a weak ferromagnetic coupling interaction for Gd⋯Gd and Gd⋯Cr in compound 1, with its magnetic entropy change (−ΔSm) reaching 21.1 J kg−1 K−1 at 5 K, 7 T, while compound 2 displayed an obvious frequency-dependency at Hdc = 2000 Oe.
1. Introduction
Due to its existence for many years, the chemistry of lanthanide-transition metal clusters is no longer an emerging discipline, but researchers' enthusiasm for high-nuclearity lanthanide-transition metal clusters is still unabated. Specifically, researchers focused initially on their beautiful and nearly perfect molecular structures (such as Keplerate-type Ln20Ni30,1 nesting doll-like Ln54Ni54
2 and Ln60Ni76),3,4 later on exploration and promotion of their properties in optics,5,6 magnetism7–11 and catalysis,12–15 and recently on their assembly mechanism.16–19 These reports indicate that high-nuclearity lanthanide-transition metal clusters are still an interesting research field for further investigation.
Compared with other frequently reported mature lanthanide-transition metal clusters,20 there are relatively few reports on lanthanide–chromium (Ln–Cr) clusters, especially those with more than ten metals.21–28 The combination of Cr3+ ions of negative magnetic anisotropy and a large spin ground state value with Ln3+ ions can form Ln–Cr polymetallic complexes with good magnetic properties, such as single molecule magnet (SMM)29–32 and magnetocaloric effect (MCE).27,33–36 Cryogenic magnetic refrigerant depends on MCE to achieve the cooling purpose in the low temperature zone. High-nuclearity Ln–Cr clusters have a potential application value as cryogenic magnetic cooling materials due to the advantages of a large spin ground state value, high magnetic density, and negative magnetic anisotropy. However, the inert nature of Cr3+ ions makes it difficult to combine with Ln3+ ions, suggesting the necessity of a suitable ligand or synthetic strategy for constructing high-nuclearity Ln–Cr clusters. Additionally, MCE is closely related with magnetic coupling between metal ions.36,37 The magnetic exchange interaction is slightly stronger between 3d–4f metal ions than between 4f–4f metal ions, which can avoid the strong magnetic exchange interaction between 3d–3d metal ions. Thus far, few studies have been performed regarding the magnetic exchange between metal ions in high-nuclearity Ln–Cr clusters.22,26,27
Ligand-controlled hydrolysis is shown as an effective strategy for synthesizing high-nuclearity lanthanide-containing metal clusters,38,39 due to the hydrolysis control of metal ions by ligands, and their protection and stabilization of the metal core. Hard–Soft Acid–Base (HSAB) theory reveals the chelating ligand containing N and O groups as the first candidate for synthesizing high-nuclearity lanthanide-transition metal clusters. Here, we selected 2-pyrazinecarboxylic acid as the primary ligand and acetate as co-ligand to control the degree of hydrolysis of metal ions, and obtained two dimeric Ln–Cr clusters with the formulas {Ln(H2O)8[Ln6Cr3(L)6(CH3COO)6(μ3-OH)12(H2O)12]}·(ClO4)6·xH2O (Ln = Gd, x = 35 for 1 and Ln = Dy, x = 45 for 2, HL = 2-pyrazinecarboxylic acid). Single crystal structure analysis showed the formation of the metal core {Ln6Cr3} from two trigonal bipyramids {Ln3Cr2} sharing a common top of Cr3+ ion. Magnetic calculations indicated a weak ferromagnetic coupling interaction for Gd⋯Gd and Gd⋯Cr in compound 1, with its magnetic entropy change (−ΔSm) reaching 21.1 J kg−1 K−1 at 5 K, 7 T, while compound 2 displayed an obvious frequency-dependency at Hdc = 2000 Oe.
2. Experimental section
2.1 Materials and methods
In this study, all commercially purchased reagents were of analytical grade and used as purchased without further purification. Ln2O3 (Ln = Gd/Dy) was dissolved by slowly adding perchloric acid aqueous solution (70.0–72.0%, 64.0 mL) which was performed in the fume cupboard. Aqueous solution of Ln(ClO4)3 (1.0 M) was obtained by diluting the concentrated solution to 250 mL (pH ∼ 0.25). The magnetic susceptibility data (2–300 K) of powder samples were obtained with a SQUID magnetometer (Quantum Design MPMSXL). A Vario EL-3 elemental analyzer was used for elemental analysis (EA) of C, H, and N in the powder samples after drying for one week in an air dryer, and a NICOLET iS50 FT-IR spectrophotometer with pressed KBr pellets was used to record the infrared spectra. Meanwhile, a NETZSCH TG209F3 thermal analyzer was used for thermogravimetric analysis (TGA) at a heating rate of 2.0 °C min−1 and an air flow of 20.0 L min−1. The purity of the products was checked by X-ray powder diffraction on a BRUKER D2 PHASER powder X-ray diffractometer. The powder samples used here were obtained by milling the hexagonal flake crystals. UV-Vis absorption spectra was performed by TU1810 DPC ultraviolet spectrograph.
2.2 Synthesis of {Gd(H2O)8[Gd6Cr3(L)6(CH3COO)6(μ3-OH)12(H2O)12]}·(ClO4)6·35H2O (1)
Briefly, Gd(ClO4)3 (4.0 mmol, 4.0 mL, 1.0 M), Cr(OAc)3 (0.5 mmol, 114.5 mg) and 2-pyrazinecarboxylic acid (HL, 1.0 mmol, 124.1 mg) were dissolved in the round-bottom flask with EtOH (10.0 mL), followed by adjusting pH to about 5.5 with Et3N (1.5 mmol, 0.2 mL), and then heating and stirring through the reflux condenser tube at 90 °C for 2 h. After filtration, the mixture was placed in a breaker and evaporated in air for 3 days, and then light purple hexagonal flake crystals were collected; yield ∼15% based on Gd(ClO4)3. Anal. calcd (%) for C42H158N12Cl6Cr3Gd7O115: C, 12.19; H, 3.96; N, 3.74. Found (%): C, 12.15; H, 3.89; N, 3.80. IR (KBr, cm−1): 3400 (s), 2361 (w), 2341 (w), 2021 (w), 1625 (m), 1587 (m), 1524 (w), 1528 (w), 1474 (w), 1430 (s), 1381 (s), 1347 (m), 1290 (s), 1144 (w), 1110 (w), 1089 (w), 1033 (s), 939 (s), 862 (w), 790 (m), 736 (w), 721 (w), 676 (m), 636 (w), 626 (s), 549 (m), 455 (m).
2.3 Synthesis of {Dy(H2O)8[Dy6Cr3(L)6(CH3COO)6(μ3-OH)12(H2O)12]}·(ClO4)6·45H2O (2)
The synthesis was performed using a procedure similar to that for 1 but with Dy(ClO4)3 (4.0 mmol, 4.0 mL, 1.0 M) to replace Gd(ClO4)3 (4.0 mmol, 4.0 mL, 1.0 M). After 3 days of air evaporation, light purple hexagonal flake crystals were obtained. (Yield ∼ 15%) anal. calcd (%) for C42H178N12Cl6Cr3Dy7O125: C, 11.51; H, 3.70; N, 3.56. Found (%): C, 11.55; H, 3.65; N, 3.60. IR (KBr, cm−1): 3400 (s), 2361 (w), 2341 (w), 2021 (w), 1625 (m), 1587 (m), 1524 (w), 1528 (w), 1474 (w), 1430 (s), 1381 (s), 1347 (m), 1290 (s), 1144 (w), 1110 (w), 1089 (w), 1033 (s), 939 (s), 862 (w), 790 (m), 736 (w), 721 (w), 676 (m), 636 (w), 626 (s), 549 (m), 455 (m).
2.4 X-ray crystal structure analysis
A STOE STADIVARI detector was used to collect the crystal X-ray diffraction data of compounds 1–2 with Cu Kα radiation (λ = 1.54184 Å) at 120 K, with the multi-scan program STOE LANA being applied for their absorption corrections, and the Olex2 program for the solution of the structures by the direct method and the anisotropical refinement of non-hydrogen atoms by full-matrix least-squares on F2.40–43 The H atoms of the organic ligand were positioned geometrically with C–H = 0.96 Å. The crystal data and the detailed information about the collection and refinement of the complexes are presented in Table S1,† and the selected bonds are shown in Tables S2 and S3.† Charge balance, EA and TGA analyses revealed 6 ClO4− and 35 guest water molecules in compound 1 while 6 ClO4− and 45 guest water molecules in compound 2, which were all removed using SQUEEZE due to disorder.44 In this paper, CCDC contains the ESI crystallographic data submitted to the Cambridge Crystallographic Data Centre with the deposition number of 1964545 and 1964546 for 1–2, respectively.†
3. Results and discussion
3.1 Synthesis strategy and crystal structure analysis
Ligand-controlled hydrolysis is an effective strategy for synthesis of high-nuclearity metal clusters, and small ligands are the first choice due to their small steric hindrance effect. For high-nuclearity lanthanide-transition metal clusters, the results may be surprising for the hydrolysis of metal ions controlled by the mixed ligands containing both N and O groups and small carboxylic ligands. Ln7Cr3 was prepared through the reaction of HL, Cr(OAc)3 and Ln(ClO4)3 (Ln = Gd/Dy) with a ratio of 0.5
:
0.5
:
2. Meanwhile, the yield of the products was increased when the molar ratio was changed to 1
:
0.5
:
4. However, Ln7Cr3 could not be obtained by substituting Cr(OAc)3 with Cr(NO3)3·9H2O in the same procedure, suggesting that acetate plays an important role as co-ligand in the formation of Ln7Cr3.
Compounds 1 and 2 are isomorphic and crystalize in the monoclinic space group C2/c, and thus the structure of compound 1 is used as a representative example for detailed description (Fig. 1). Compound 1 consists of a cationic cluster core of [Gd6Cr3(L)6(CH3COO)6(μ3-OH)12(H2O)12]3+, a free mononuclear cation of [Gd(H2O)8]3+, 6 ClO4− anions and 35 guest water molecules. Two cationic cluster units of [Gd3Cr2(L)3(CH3COO)3(μ3-OH)6]3+ (Fig. 2a) featuring a trigonal bipyramid configuration share a common vertex of the central Cr3+ ion and generate the dimeric cationic cluster core of [Gd6Cr3(L)6(CH3COO)6(μ3-OH)12]3+ (Fig. 2b). Interestingly, each ligand L− in 1 is only coordinated with one Gd3+ ion by one O atom on the carboxyl and one neighboring N group (Fig. S1†), and the two N groups contained therein are not chelated with any Cr3+ ion. For the trigonal bipyramid unit of [Gd3Cr2(L)3(CH3COO)3(μ3-OH)6(H2O)6]3+, Gd3+ and Cr3+ ions are linked by one acetate and two μ3-OH groups, and three Gd3+ ions lie in the same plane and are connected to each other through three μ3-OH groups. Building block strategy is commonly used in metal–organic frameworks.45 However, investigations on high-nuclearity lanthanide-containing clusters found that theirs structures are usually assembled by simple building blocks,46 with tetrahedron and tetrapyramid as common basic building blocks in high-nuclearity lanthanide-transition metal clusters, while trigonal bipyramid as building block is rarely reported.47–49 For compound 1, the two trigonal bipyramids of [Gd3Cr2(μ3-OH)6]9+ (Fig. 2c) can be regarded as the building blocks for constructing the metal-oxo cluster core of [Gd6Cr3(μ3-OH)12]15+ (Fig. 2d). The three Gd3+ ions in the trigonal bipyramid {Ln3Cr2} (Fig. 2e) are located in the same plane, with the upper and lower metal core units of {Ln3Cr2} sharing the vertices in a centrally symmetrical manner to produce the metal core of {Ln6Cr3} (Fig. 2f). The typical trigonal bipyramid (TBP) geometry for the metal core of {Ln3Cr2} was further confirmed by the structural index parameter τ = 0.01 (α = 104.3 and β = 103.7°) obtained from the equation τ = (β − α)/60 reported by Addison et al.50 The distances of Gd⋯Gd and Gd⋯Cr are in the range of 3.9962–4.0102 Å and 3.4630–3.5209 Å, respectively, which agree with the previous report.27 Additionally, compound 1 forms a three-dimensional framework with one-dimensional nanopore channel along the a-axis (Fig. 3) through the hydrogen-bond interaction of coordinated water molecules between the cationic cluster core of [Gd6Cr3(L)6(CH3COO)6(μ3-OH)12(H2O)12]3+ and the free mononuclear cation of [Gd(H2O)8]3+ (Fig. S2a†) and the π-bond interactions of the pyrazine groups on the two cationic cluster cores (Fig. S2b†). The guest water molecules play a role in filling the nanopore channel in the framework. In addition, UV-Vis absorption spectra of HL and compounds 1–2 indicates that the main peak at 270 nm belongs to the conjugated transition of the ligand pyrazine ring π–π*.
 |
| Fig. 1 Ball and stick views of the cationic cluster of {Gd(H2O)8[Gd6Cr3(L)6(CH3COO)6(μ3-OH)12(H2O)12]}6+. | |
 |
| Fig. 2 (a) The cationic cluster unit of [Gd3Cr2(L)3(CH3COO)3(μ3-OH)6]3+. (b) The dimeric cationic cluster core of [Gd6Cr3(L)6(CH3COO)6(μ3-OH)12]3+. (c) Trigonal bipyramid of [Gd3Cr2(μ3-OH)6]9+ as the basic building block. (d) The metal-oxo cluster core of [Gd6Cr3(μ3-OH)12]15+. (e) and (f) The metal arrangement of {Ln3Cr2} and {Ln6Cr3}, respectively. | |
 |
| Fig. 3 The three-dimensional framework with one-dimensional nanopore channel along the a-axis of compound 1. | |
All of the Cr3+ ions are in the hexa-coordinate mode with an octahedral configuration (Fig. S3a†). There are two coordination environments for Gd3+ ions in compound 1. Six Gd3+ ions of the cationic cluster core of [Gd6Cr3(L)6(CH3COO)6(μ3-OH)12(H2O)12]3+ are in the nona-coordinate mode with a capped square antiprism geometry (Fig. S3b†),51 and the Gd3+ ion from the free mononuclear cation of [Gd(H2O)8]3+ is in the octa-coordinate mode with a biaugmented trigonal prism geometry (Fig. S3c†).52 The geometrical configurations of Gd3+ ions are further proved by the continuous shape measurement values (CShM) calculated by using the SHAPE program of Alvarez.20 (Table S4†).53 The bond distances of Gd⋯O and Cr⋯O are 2.3371–2.4778 Å and 1.9479–2.0131 Å, respectively, which are similar to the previously reported values for Ln–Cr clusters.27
3.2 Magnetic properties
The magnetic susceptibility was measured for compounds 1–2 with the dried powder samples in the temperature range of 2–300 K and in a magnetic field of 1000 Oe (Fig. 4). The χMT values for 1 and 2 at 300 K were found to be 58.7 and 99.8 cm3 K mol−1, respectively, close to the theoretical values of 60.8 and 104.8 cm3 K mol−1 based on isolated spin centers containing 3 Cr3+ (S = 3/2, g = 2) and 7 Ln3+ (Gd3+: J = 7/2, g = 2, Dy3+: J = 15/2, g = 4/3) ions. As temperature decreased, the χMT values remained almost unchanged until 100 K, followed by a gradual increase in the temperature range of 100 K and 10 K and then a sharp increase to the maximum of 174.5 and 184.9 cm3 K mol−1 at 2 K for 1 and 2, respectively, which might be attributed to weak ferromagnetic coupling interaction in the low temperature range. After fitting the χMT vs. T curves by Curie–Weiss law (Fig. S4a†), compounds 1 and 2 showed the Weiss constant θ = 4.05 and 0.40 K and Curie constant C = 59.2 and 100.9 cm3 K mol−1, respectively. The positive Weiss constant values (4.05 and 1.99 K) displayed the dominance of weak ferromagnetic coupling interaction in 1–2.
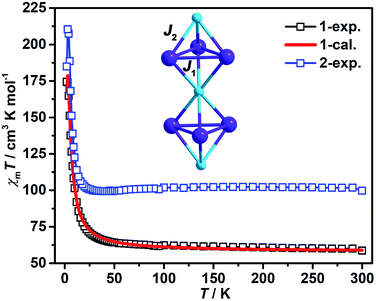 |
| Fig. 4 The magnetic susceptibility of 1–2 was measured using the dried powder samples in the temperature range of 2–300 K and in a magnetic field of 1000 Oe. Experimental values are shown by the point plots and the fitting results by the red line. Insert shows the interaction diagram between metal ions and 2 J models. | |
The magnetic coupling interaction in compound 1 was further investigated by using the Quantum Monte Carlo (QMC) method to calculate the magnetic exchange constant J between metal ions by fitting the χMT vs. T curve. As shown in Fig. 4, there are two magnetic coupling paths in 1, which are labeled as J1 and J2, with J1 for interaction between 2 Gd3+ ions in the distance range of 3.9962–4.0101 Å linked by 2 μ3-OH groups, while J2 for mixed magnetic interaction between 1 μ2-OAc and 2 μ3-OH bridges for Gd⋯Cr in the distance range of 3.5029–3.5523 Å. The optimal calculated results are J1 = 0.367 cm−1, J2 = 0.431 cm−1, D1 = 0.000 cm−1, D2 = 1.190 cm−1, g = 2.092, zJ = 0.000 cm−1, and R = 3.546 × 10−4 (R is the least reliability factor). The positive values of J1 and J2 (0.367 cm−1 and 0.431 cm−1) indicate the dominance of weak ferromagnetic coupling interactions of Gd⋯Gd and Gd⋯Cr in 1, which are consistent with the experimental results.
Based on the large spin ground state value and negative magnetic anisotropy of compound 1, the field-dependent magnetization was measured in the temperature range of 2.0–10.0 K and in the magnetic field range of 0–7 T (Fig. 5a). According to the Maxwell equation
54 compound 1 showed the maximal −ΔSm value of 21.1 J kg−1 K−1 at 5 K, 7 T (Fig. 5b), which was obviously lower than the estimated value of 37.57 J kg−1 K−1 (calculated by equation −ΔSm = nR
ln(2S + 1)), which can be attributed to the small magnetic density induced by the large organic ligand L− in compound 1. Meanwhile, the temperature dependence of ac magnetic susceptibility was measured for 2 with Hdc = 2000 Oe at a different frequency. As shown Fig. 5c, frequency-dependent signals were observed before 10 K, implying slow relaxation of magnetization. However, no obvious peaks were observed because of quantum tunneling of magnetization (QTM).55,56 The relaxation time and energy barrier could be obtained by the simplified Debye function ln(χ′′/χ′) = ln(2πfτ0) + U/kT.57,58 Their values were shown as τ0 = 3.98 × 10−6 s and Ueff = 1.15 K, which are close to the anticipated characteristic energy gaps of 10−5–10−12 s for Dy-containing cluster-based SMMs.59,60
 |
| Fig. 5 (a) Magnetization for 1 in the temperature range of 2 and 10 K and the magnetic field range of 0–7 T. (b) −ΔSm obtained from magnetization data of 1. (c) Frequency dependence of χ′′ and χ′ (insert) with Hdc = 2000 Oe. (d) Results calculated by the simplified Debye function in the temperature range of 2.0–3.0 K. | |
4. Conclusions
In summary, two decanuclear Ln–Cr clusters of Ln7Cr3 were prepared based on 2-pyrazinecarboxylic acid and acetate as mixed protecting ligands. Structure analysis showed that two trigonal bipyramids {Ln3Cr2} formed the metal core of {Ln6Cr3} as building blocks by sharing a common vertex of Cr3+ ion. Moreover, compound Ln7Cr3 could form the three-dimensional framework with one-dimensional nanopore channel along the a-axis through the hydrogen-bond interaction between the cationic cluster core and the free mononuclear cation of [Ln(H2O)8]3+ and the π-bond interaction between the pyrazine groups on the two cationic cluster cores. Magnetic experiment and calculation results indicated the dominance of weak ferromagnetic coupling interaction of Gd⋯Gd and Gd⋯Cr in 1. Meanwhile, compound 1 showed the maximal −ΔSm value of 21.1 J kg−1 K−1 at 5 K, 7 T, and compound 2 displayed obvious frequency-dependency but no obvious peaks due to QTM. Related work is ongoing by this group.
Conflicts of interest
There are no conflicts to declare.
Acknowledgements
The financial support for this work includes the National Natural Science Foundation of China (Grant No. 21802010, 21901002, 22022108) and the Natural Science Foundation of Anhui Province (Grant No. 1908085QB44) as well as the Open Project of the State Key Laboratory of Physical Chemistry of the Solid Surface of Xiamen University (Grant No. 201822).
Notes and references
- X.-J. Kong, Y.-P. Ren, L.-S. Long, Z. Zheng, R.-B. Huang and L.-S. Zheng, J. Am. Chem. Soc., 2007, 129, 7016–7017 CrossRef CAS PubMed.
- X. J. Kong, Y. P. Ren, W. X. Chen, L. S. Long, Z. Zheng, R. B. Huang and L. S. Zheng, Angew. Chem., Int. Ed., 2008, 47, 2398–2401 CrossRef CAS PubMed.
- X. J. Kong, L. S. Long, R. B. Huang, L. S. Zheng, T. D. Harris and Z. P. Zheng, Chem. Commun., 2009, 4354–4356 RSC.
- X.-J. Kong, L.-S. Long, Z. Zheng, R.-B. Huang and L.-S. Zheng, Acc. Chem. Res., 2009, 43, 201–209 CrossRef PubMed.
- X. Yang, D. Schipper, R. A. Jones, L. A. Lytwak, B. J. Holliday and S. Huang, J. Am. Chem. Soc., 2013, 135, 8468–8471 CrossRef CAS PubMed.
- Y. Xing, L. Q. Chen, Y. R. Zhao, X. Y. Zheng, Y. J. Zhang, X. J. Kong, L. S. Long and L. S. Zheng, Inorg. Chem., 2019, 58, 8494–8499 CrossRef CAS PubMed.
- J. B. Peng, Q. C. Zhang, X. J. Kong, Y. P. Ren, L. S. Long, R. B. Huang, L. S. Zheng and Z. Zheng, Angew. Chem., Int. Ed., 2011, 50, 10649–10652 CrossRef CAS PubMed.
- J. B. Peng, Q. C. Zhang, X. J. Kong, Y. Z. Zheng, Y. P. Ren, L. S. Long, R. B. Huang, L. S. Zheng and Z. Zheng, J. Am. Chem. Soc., 2012, 134, 3314–3317 CrossRef CAS PubMed.
- W. P. Chen, P. Q. Liao, Y. Yu, Z. Zheng, X. M. Chen and Y. Z. Zheng, Angew. Chem., Int. Ed., 2016, 55, 9375–9379 CrossRef CAS PubMed.
- W. P. Chen, J. Singleton, L. Qin, A. Camon, L. Engelhardt, F. Luis, R. E. P. Winpenny and Y. Z. Zheng, Nat. Commun., 2018, 9, 2107–2112 CrossRef PubMed.
- D. P. Liu, X. P. Lin, H. Zhang, X. Y. Zheng, G. L. Zhuang, X. J. Kong, L. S. Long and L. S. Zheng, Angew. Chem., Int. Ed., 2016, 55, 4532–4536 CrossRef CAS PubMed.
- R. Chen, Z. H. Yan, X. J. Kong, L. S. Long and L. S. Zheng, Angew. Chem., Int. Ed., 2018, 57, 16796–16800 CrossRef CAS PubMed.
- W.-P. Chen, P.-Q. Liao, P.-B. Jin, L. Zhang, B.-K. Ling, S.-C. Wang, Y.-T. Chan, X.-M. Chen and Y.-Z. Zheng, J. Am. Chem. Soc., 2020, 142, 4663–4670 CrossRef CAS PubMed.
- R. Chen, G.-L. Zhuang, Z.-Y. Wang, Y.-J. Gao, Z. Li, C. Wang, Y. Zhou, M.-H. Du, S. Zeng, L.-S. Long, X.-J. Kong and L.-S. Zheng, Natl. Sci. Rev., 2020, nwaa234 CrossRef.
- R. Chen, C.-L. Chen, M.-H. Du, X. Wang, C. Wang, L.-S. Long, X.-J. Kong and L.-S. Zheng, Chem. Commun., 2021, 57, 3611–3614 RSC.
- H. Zheng, M.-H. Du, S.-C. Lin, Z.-C. Tang, X.-J. Kong, L.-S. Long and L.-S. Zheng, Angew. Chem., Int. Ed., 2018, 57, 10976–10979 CrossRef CAS PubMed.
- X.-Y. Zheng, M.-H. Du, M. Amiri, M. Nyman, Q. Liu, T. Liu, X.-J. Kong, L.-S. Long and L.-S. Zheng, Chem.–Eur. J., 2020, 26, 1388–1395 CrossRef CAS PubMed.
- M.-H. Du, X.-Y. Zheng, X.-J. Kong, L.-S. Long and L.-S. Zheng, Matter, 2020, 3, 1–16 CrossRef.
- X. Y. Zheng, M. T. Chen, M. H. Du, R. J. Wei, X. J. Kong, L. S. Long and L. S. Zheng, Chem.–Eur. J., 2020, 26, 11985–11988 CrossRef CAS PubMed.
- X.-Y. Zheng, X.-J. Kong and L.-S. Long, in Recent Development in Clusters of Rare Earths and Actinides: Chemistry and Materials, ed. Z. Zheng, Springer Berlin Heidelberg, Berlin, Heidelberg, 2017, pp. 51–96 Search PubMed.
- S. Schmitz, J. van Leusen, N. V. Izarova, Y. Lan, W. Wernsdorfer, P. Kögerler and K. Y. Monakhov, Dalton Trans., 2016, 45, 16148–16152 RSC.
- L. Qin, J. Singleton, W. P. Chen, H. Nojiri, L. Engelhardt, R. E. P. Winpenny and Y. Z. Zheng, Angew. Chem., Int. Ed., 2017, 56, 16571–16574 CrossRef CAS PubMed.
- Y.-M. Han, N.-F. Li, Y.-Z. Yu, J.-P. Cao, M.-X. Yang, Y.-L. Hong, R.-K. Kang, P. Yuan and Y. Xu, RSC Adv., 2020, 10, 11365–11370 RSC.
- Y.-N. Gu, H. Yu, L.-D. Lin, Y.-L. Wu, Z. Li, W.-Y. Pan, J. He, L. Chen, Q. Li and X.-X. Li, New J. Chem., 2019, 43, 3011–3016 RSC.
- S. Chen, V. Mereacre, Z. Zhao, W. Zhang, M. Zhang and Z. He, Dalton Trans., 2018, 47, 7456–7462 RSC.
- Z. Fu, L. Qin, K. Sun, L. Hao, Y.-Z. Zheng, W. Lohstroh, G. Günther, M. Russina, Y. Liu, Y. Xiao, W. Jin and D. Chen, npj Quantum Mater., 2020, 5, 32–37 CrossRef CAS.
- J. J. Yin, C. Chen, G. L. Zhuang, J. Zheng, X. Y. Zheng and X. J. Kong, Inorg. Chem., 2020, 59, 1959–1966 CrossRef CAS PubMed.
- L. Qin, H.-L. Zhang, Y.-Q. Zhai, H. Nojiri, C. Schröder and Y.-Z. Zheng, iScience, 2021, 24, 102350 CrossRef PubMed.
- J. Rinck, G. Novitchi, W. Van den Heuvel, L. Ungur, Y. Lan, W. Wernsdorfer, C. E. Anson, L. F. Chibotaru and A. K. Powell, Angew. Chem., Int. Ed., 2010, 49, 7583–7587 CrossRef CAS PubMed.
- X.-Q. Wang, Z.-Y. Li, Z.-X. Zhu, J. Zhu, S.-Q. Liu, J. Ni and J.-J. Zhang, Eur. J. Inorg. Chem., 2013, 2013, 5153–5160 CrossRef CAS.
- K. R. Vignesh, S. K. Langley, A. Swain, B. Moubaraki, M. Damjanović, W. Wernsdorfer, G. Rajaraman and K. S. Murray, Angew. Chem., Int. Ed., 2018, 57, 779–784 CrossRef CAS PubMed.
- S. K. Langley, D. P. Wielechowski, V. Vieru, N. F. Chilton, B. Moubaraki, B. F. Abrahams, L. F. Chibotaru and K. S. Murray, Angew. Chem., Int. Ed., 2013, 52, 12014–12019 CrossRef CAS PubMed.
- Y. Yu, X. Pan, C. Cui, X. Luo, N. Li, H. Mei and Y. Xu, Inorg. Chem., 2020, 59, 5593–5599 CrossRef CAS PubMed.
- J.-J. Yin, T.-Q. Lu, C. Chen, G.-L. Zhuang, J. Zheng, X.-Y. Zheng and F. Shao, Cryst. Growth Des., 2020, 20, 4005–4012 CrossRef CAS.
- Z.-Y. Li, J.-J. Zhang, S.-Q. Liu, H. Zhang, Y.-J. Sun, X.-Y. Liu and B. Zhai, Cryst. Growth Des., 2018, 18, 7335–7342 CrossRef CAS.
- C. Cui, J. P. Cao, X. M. Luo, Q. F. Lin and Y. Xu, Chem.–Eur. J., 2018, 24, 15295–15302 CrossRef CAS PubMed.
- J.-L. Liu, Y.-C. Chen, F.-S. Guo and M.-L. Tong, Coord. Chem. Rev., 2014, 281, 26–49 CrossRef CAS.
- Z. Zheng, Chem. Commun., 2001, 2521–2529 RSC.
- X. Y. Zheng, X. J. Kong, Z. Zheng, L. S. Long and L. S. Zheng, Acc. Chem. Res., 2018, 51, 517–525 CrossRef CAS.
- O. V. Dolomanov, L. J. Bourhis, R. J. Gildea, J. A. K. Howard and H. Puschmann, J. Appl. Crystallogr., 2009, 42, 339–341 CrossRef CAS.
- M. Fugel, D. Jayatilaka, E. Hupf, J. Overgaard, V. R. Hathwar, P. Macchi, M. J. Turner, J. A. K. Howard, O. V. Dolomanov, H. Puschmann, B. B. Iversen, H.-B. Bürgi and S. Grabowsky, IUCrJ, 2018, 5, 32–44 CrossRef CAS PubMed.
- G. M. Sheldrick, Acta Crystallogr., Sect. A: Found. Adv., 2015, 71, 3–8 CrossRef PubMed.
- G. M. Sheldrick, Acta Crystallogr., Sect. C: Struct. Chem., 2015, 71, 3–8 Search PubMed.
- A. Spek, Acta Crystallogr., Sect. C: Struct. Chem., 2015, 71, 9–18 CrossRef CAS PubMed.
- L. Cao, T. Wang and C. Wang, Chin. J. Chem., 2018, 36, 754–764 CrossRef CAS.
- X.-Y. Zheng, J. Xie, X.-J. Kong, L.-S. Long and L.-S. Zheng, Coord. Chem. Rev., 2019, 378, 222–236 CrossRef CAS.
- H.-J. Lun, X.-J. Kong, L.-S. Long and L.-S. Zheng, Dalton Trans., 2020, 49, 2421–2425 RSC.
- S. K. Langley, L. Ungur, N. F. Chilton, B. Moubaraki, L. F. Chibotaru and K. S. Murray, Chem.–Eur. J., 2011, 17, 9209–9218 CrossRef CAS PubMed.
- S. K. Langley, N. F. Chilton, B. Moubaraki, T. Hooper, E. K. Brechin, M. Evangelisti and K. S. Murray, Chem. Sci., 2011, 2, 1166–1169 RSC.
- A. W. Addison, T. N. Rao, J. Reedijk, J. van Rijn and G. C. Verschoor, J. Chem. Soc., Dalton Trans., 1984, 1349–1356 RSC.
- A. Ruiz-Martinez, D. Casanova and S. Alvarez, Chem.–Eur. J., 2008, 14, 1291–1303 CrossRef CAS PubMed.
- D. Casanova, M. Llunell, P. Alemany and S. Alvarez, Chem.–Eur. J., 2005, 11, 1479–1494 CrossRef CAS PubMed.
- M. Pinsky and D. Avnir, Inorg. Chem., 1998, 37, 5575–5582 CrossRef CAS PubMed.
- M. Evangelisti and E. K. Brechin, Dalton Trans., 2010, 39, 4672–4676 RSC.
- V. S. Parmar, F. Ortu, X. Ma, N. F. Chilton, R. Clerac, D. P. Mills and R. E. P. Winpenny, Chem.–Eur. J., 2020, 26, 7774–7778 CrossRef CAS PubMed.
- Y. S. Ding, K. X. Yu, D. Reta, F. Ortu, R. E. P. Winpenny, Y. Z. Zheng and N. F. Chilton, Nat. Commun., 2018, 9, 3134–3143 CrossRef PubMed.
- D. Reta and N. F. Chilton, Phys. Chem. Chem. Phys., 2019, 21, 23567–23575 RSC.
- K. S. Cole and R. H. Cole, J. Chem. Phys., 1941, 9, 341–351 CrossRef CAS.
- J. Cai, R. Ye, X. Liu, L. Guo and X. Qiao, Dalton Trans., 2020, 49, 16954–16961 RSC.
- K. Zhang, V. Montigaud, O. Cador, G.-P. Li, B. Le Guennic, J.-K. Tang and Y.-Y. Wang, Inorg. Chem., 2018, 57, 8550–8557 CrossRef CAS PubMed.
Footnotes |
† Electronic supplementary information (ESI) available: IR, TGA, Fig. S1–S8 and Tables S1–S4. CCDC 1964545 and 1964546. For ESI and crystallographic data in CIF or other electronic format see DOI: 10.1039/d1ra02734d |
‡ These authors contributed equally to this work. |
|
This journal is © The Royal Society of Chemistry 2021 |
Click here to see how this site uses Cookies. View our privacy policy here.