DOI:
10.1039/D1RA01886H
(Paper)
RSC Adv., 2021,
11, 12907-12914
Degradation and mechanism analysis of chloroxylenol in aqueous solution by gas–liquid discharge plasma combined with ozonation†
Received
10th March 2021
, Accepted 16th March 2021
First published on 6th April 2021
Abstract
Gas–liquid discharge non-thermal plasma (NTP) coupled with an ozonation reactor was used to investigate the removal of a broad-spectrum antibacterial agent, chloroxylenol (PCMX), from aqueous solution. Under the same experimental conditions (discharge power of 50.25 W, the initial concentration of PCMX of 60 mg L−1, oxygen flow of 1.0 L min−1 and PCMX solution flow of 150 mL min−1), the PCMX degradation rates in the ozonation-only, NTP-only and NTP/O3 systems were 29.25%, 67.04% and 79.43%, respectively. Correspondingly, the energy efficiency has also been greatly improved, and increased to 0.45, 1.03 and 1.21 g kW−1 h−1. In addition, the effects of the initial concentration of PCMX, initial pH, the flow rate of oxygen, the addition of H2O2 and the addition of a radical scavenger on the degradation rate of PCMX were investigated in the NTP/O3 system. The degradation rate in acidic solutions was higher than that in alkaline solutions. During the removal process of PCMX, the rate of degradation was strongly increased with the addition of H2O2 and acutely decreased with the addition of the radical scavenger. Compared with deionized water the degradation rates of PCMX in secondary effluent were inhibited. Four main intermediates of PCMX degradation by the NTP/O3 system were identified by gas chromatography-mass spectrometry (GC-MS) and a possible degradation pathway of PCMX was proposed. The changes in toxicity of the PCMX solution during the NTP/O3 system oxidation process were also evaluated using bioluminescent bacteria and Quantitative Structure Activity Relationship (QSAR) models with the help of the ECOSAR software.
1 Introduction
In recent years, pharmaceutical and personal care products (PPCPs) as important emerging contaminants (ECs) have received widespread attention because their existence in water and wastewater causes significant damage to the ecosystem and human beings.1,2 The PPCPs in the environment are characterized by their extreme volatility, strong thermal stability, and good solubility in water and various organic solvents.3
Chloroxylenol (PCMX) is a broad-spectrum antibacterial agent that may kills most bacterial propagules and fungi.4 With its high antibacterial activity and good skin compatibility, PCMX has been widely applied in the production of personal care, medicine and daily consumer products, such as hand soap, laundry detergent, bactericides, and cosmetics. Owing to its mass production and application, this disinfectant compound can be discharged directly or through urban sewage systems into aquatic systems. The degradation rate of PCMX by municipal sewage treatment plants is only 80% in Baltimore, Maryland, USA.5 In an aquatic environment, the toxicity of PCMX varies by species, it is essentially non-toxic to humans and mammals, but exhibits toxicity to freshwater invertebrates and fish.4,6 Therefore, it is becoming increasingly urgent to remove PPCPs from the water using effective approaches. Many advanced processes have been used to remove PCMX from water and wastewater, such as electrochemical oxidation,7 ozonation, ultraviolet irradiation,8 adsorption and combinations of these techniques.
Advanced oxidation processes have attracted wide attention as an environmentally friendly and efficient technology for treating water and wastewater containing organic pollutants. The gas–liquid discharge non-thermal plasma (NTP) process as a typical advanced oxidation process has gained increasing attention for its organic pollutant abatement from water and wastewater due to its high efficiency and minimal secondary impact on the environment and ecosystems. It is regarded as a promising technology for water treatment. Plasma technology is a hybrid advanced oxidation technology that combines both physical and chemical effects of high-energy electronic collision, reactive species, ultraviolet light and shock waves.9 In the plasma discharge process, a rich variety of active species such as H2O2, ˙H, ˙O, ˙OH and O3 can be formed.10,11 These species play a vital role in pollution abatement processes. Among these existing active species, ˙OH, one of the strongest oxidant with a higher reduction potential (2.80 eV), which is able to oxidize non-selectively a broad range of organic contaminants.12 NTP oxidation of various refractory organic pollutants have been widely researched, such as the oxidation of textile dyes,13 pharmaceuticals,14 endocrine disrupting chemicals,15 biotoxin16 and pesticides.17
The process of NTP including various physical and chemical effects. Some various oxidizing species with high redox ability, which could degrade most organic pollutants effectively and non-selectively. When air or oxygen is used as the discharge gas, a large amount of ozone is generated. Due to the reactor design and mass transfer of gas–liquid two phases, most of the ozone will be discharged along with the exhaust gas, resulting in reducing energy utilization of NTP and increasing environmental risk. How to use ozone and improve energy efficiency has become a common concern.
In this study, the influencing factors such as initial concentration of PCMX, pH, applied oxygen flow rate and the addition of radical scavenger on the removal of PCMX were studied. Hydrogen peroxide as a free radical promoter was added to the NTP/O3 system to investigate its effect on organic degradation. The contribution of hydroxyl radicals to the removal of PCMX was also indirectly investigated with the addition of radical scavengers tert-butyl alcohol (TBA). Additionally, the degradation products under NTP/O3 system were detected and analysed with Gas Chromatography and Mass Spectrometry (GC-MS), the possible degradation pathways and mechanisms were also proposed. The change of toxicity of PCMX solution during NTP/O3 system oxidation process was also evaluated.
2 Experimental materials and methods
2.1 Materials
PCMX, (purity ≥ 99.8%) was purchased from Yousuo Chemistry Co., Ltd (Shandong, China). tert-Butyl alcohol (TBA) and methylene chloride were obtained from Aladdin (Shanghai, China). Other regents and solvents (analytical grade) used in this research were purchased from Sinopharm Chemical Reagent Co., Ltd., China. All of the solutions used in this study were prepared by distilled-deionized water with a Millipore Milli-Q system.
2.2 Experimental setup
In this research, three experimental operating systems were designed, including plasma-only system, ozonation-only system and plasma/O3 system. The schemes of the three reactors are shown in Fig. 1. The operating conditions were as follows: discharge power of 50.25 W, the initial concentration of PCMX of 60 mg L−1, oxygen flow of 1 L min−1. The total volume of the PCMX solution was 300 mL, and the circulated flow rate was 150 mL min−1. In the NTP/O3 system, the volumes of PCMX solution in the NTP discharge reactor and O3 oxidation reactor were about 100 mL and 110 mL respectively. Unless otherwise specified, the test conditions in the text did not change.
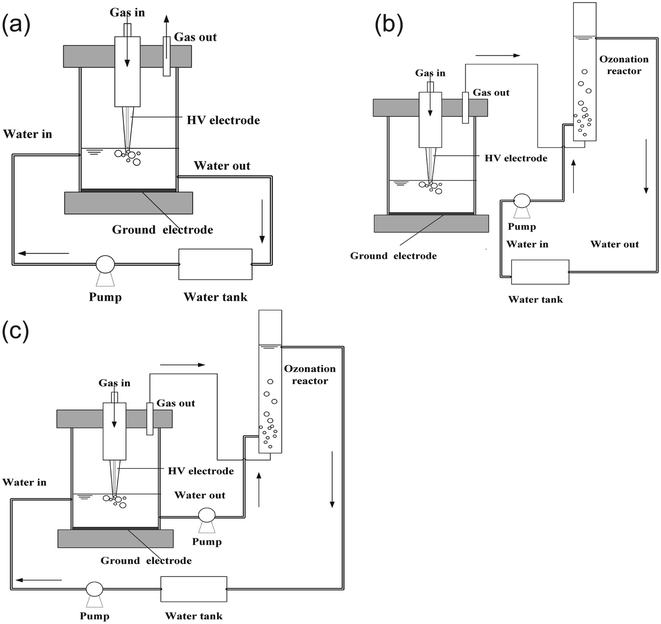 |
| Fig. 1 Experimental setups. (a) NTP-only, (b) O3-only, and (c) NTP/O3 systems. | |
The NTP-only system is shown in Fig. 1(a). The reactor was composed of glass with an inner diameter (I. D.) of 80 mm and a height of 100 mm. The plasma generator was composed of a hollow gold needle (I. D. = 0.6 mm), a concentric dielectric cone-shaped glass tube (upper I. D. = 50 mm, lower I. D. = 0.8 mm) that enclosed the needle, and a copper plate (diameter = 30 mm) beneath them. The hollow gold needle doubled as the powered electrode and the working gas inlet channel. Its tip was 10 mm away from the end of the tube nozzle in the axial direction. The copper plate and the downstream solution both served as the ground electrode. The discharge generator was powered by a sinusoidal excitation voltage (Vp = 0–40 kV) with a frequency of 0–30 kHz. The applied voltage and discharge current were measured by a high-voltage probe (Tektronix P6015A) and a current probe (Tektronix TCP150) via a digital oscilloscope (Tektronix DPO4034). The solution was circulated continuously at the speed of 150 mL min−1 by a peristaltic pump (BT100-2J) connected to the inlet.
As shown in Fig. 1(b), an aeration device was added to the base of the plasma reactor, this combined device was defined as NTP/O3 system. The aeration device consisted of a glass tube with a diameter of 3 cm and a height of 35 cm. The effective volume in the aeration tube was approximately 100 mL. In this system, the PCMX solution flowed through the aeration device and the activated exhaust (O3) which generated in the discharge was introduced to the solution. In the NTP/O3 device, as shown in Fig. 1(c), the PXMX solution not only flowed through the discharge region, but also entered the aeration device, and the activated exhaust was also introduced to the solution.
2.3 Analytical methods
The concentration of PCMX in the solution was monitored and quantified by high-performance liquid chromatography (HPLC-Waters Corporation model 515, Agilent, USA) with a C18 column (4.6 × 250 cm, 5 μm, Waters, USA), and a UV detector set at 283 nm. The mobile phase consisted of acetonitrile
:
water solution (70
:
30), and the flow rate of the mobile phase was controlled at 1.0 mL min−1. The solution pH was adjusted by 0.1 M H2SO4 or 0.1 M NaOH, which was determined by a multi-parameter portable meter (MultiLine® Multi 3620 IDS, Germany). The mineralization of the PCMX was monitored by the removal of total organic carbon (TOC), which was monitored by a TOC analyzer (TOC-V CPH, Shimadzu, Japan) by TOC = total carbon − inorganic carbon. The concentration of H2O2 in the solution was measured by the potassium titanium(IV) oxalate method.18 The concentration of O3, which was dissolved in the solution, was detected by the indigo method.19 The intermediates of PCMX during NTP/O3 oxidation were monitored by GC-MS (7890A/5975C, Agilent, USA) with a DB-5MS capillary column. Data shown are the average of three determination results. Error bars show the standard deviation.
The PCMX degradation rate (DE) was measured by the following eqn (1).
|
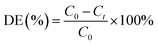 | (1) |
where
C0 and
Ct is the initial and discharge treatment time
t concentration of PCMX, mg L
−1; the degradation of PCMX by gas–liquid discharge plasma was in accordance with the pseudo-first-order kinetic model, which was calculated by the following
eqn (2).
|
 | (2) |
where
C0 and
Ct are the same definitions as
eqn (1);
k is the reaction rate constant, min
−1;
t is the reaction time, min.
The following equation was used to calculated and expressed the energy yield (defined as the amount of pollutions degraded per energy consumed) of the three different systems,20 which was calculated by the following eqn (3).
|
 | (3) |
where
C0 and
Ct are the same definitions as
eqn (1),
V is the volume of the solution, (L),
p is the average power in the discharge (kW),
t is the treatment time (h). The details of bioluminescence activity inhibition experiments are described in previous reports,
21,22 and the bioluminescence activity inhibition ration was calculated according to
eqn (4).
|
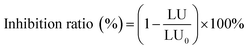 | (4) |
where LU
0 and LU was the luminescence intensities of the blank control (recovery liquid) and the tested sample, respectively.
3 Results and discussion
3.1 Chloroxylenol degradation in three different systems
The comparison of the degradation efficiency of PCMX in the three systems under the same conditions is shown in Fig. 2(a). Other experimental parameters were controlled as follows: discharge power of 50.25 W, the initial concentration of PCMX of 60 mg L−1, the initial pH of 6.34 (not adjusted), oxygen flow of 1.0 L min−1 and PCMX solution flow of 150 mL min−1. The PCMX degradation rate in the NTP/O3 system was more efficient than that in other systems. The PCMX degradation rates were 29.25% and 67.04% in the ozonation-only and NTP-only systems, respectively, after 14 min treatment. Compared with that of NTP-only system, the simultaneous use of the NTP/O3 system increased the PCMX degradation rate from 67.04% to 79.40%. The contribution of O3 to PCMX degradation was 29.25% under good mass transfer conditions, which was much lower than the 67.04% of the NTP treatment alone. To a certain extent, the O3 generated during plasma discharge does not play a decisive role in the degradation of PCMX. However, O3 has significant potential for application in plasma systems. Based on these results, we concluded that the O3 aerator improved the degradation rate of PCMX. As shown in Fig. S1,† the reaction rate constants were 0.0919, 0.0710 and 0.0234 min−1 in the NTP/O3, NTP and O3 systems, respectively. The energy yield of PCMX degradation and the TOC removal rate of the different systems were calculated, and the results as shown in Fig. 2(b). The highest energy yield and TOC removal rate were also obtained in the NTP/O3 system. These results further confirmed the significant synergistic effect of NTP/O3 on the degradation of PCMX and energy efficiency.
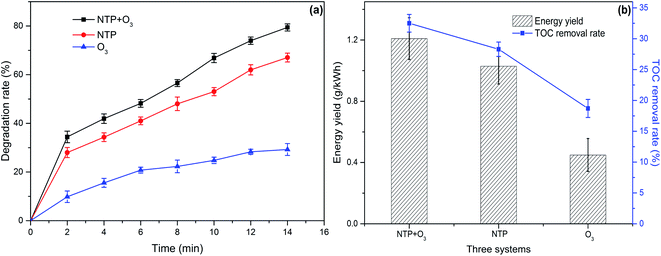 |
| Fig. 2 (a) Chloroxylenol solution degradation rate and (b) energy yield and TOC removal in the three different systems. | |
3.2 Effect of the initial concentration of chloroxylenol
The effect of the initial concentration on PCMX removal in the NTP/O3 system was investigated. As illustrated in Fig. 3(a) and S2,† under the same experimental conditions, with the increasing initial concentration of PCMX, the degradation rates and the kinetic constants decreased. The highest PCMX degradation rate was 83.7% in 40 mg L−1 solution with 14 min treatment time, which was 4.3% and 10.4% higher than that in the 60 mg L−1 and 80 mg L−1 solution, respectively. The kinetic constants at initial PCMX concentrations of 40, 60 and 80 mg L−1 were calculated as 0.1235, 0.1099 and 0.0919 mg−1, respectively. This phenomenon could be explained by the fact that under the same discharge conditions, the amounts and the types of active species were similar. When the initial concentration of PCMX increased, more intermediate products were generated, which would have stronger competition for reaction with the active radicals between PCMX molecules. However, the energy efficiency was increased with the increasing initial PCMX concentration because a higher initial concentration of PCMX meant greater collision efficiency between the active species and contaminant molecules, thus, the energy utilization efficiency was improved. For example, when the initial PCMX concentration increased from 40, 60 to 90 mg L−1, the energy yields increased from 0.8, 1.2 to 1.5 g kW−1 h−1, respectively.
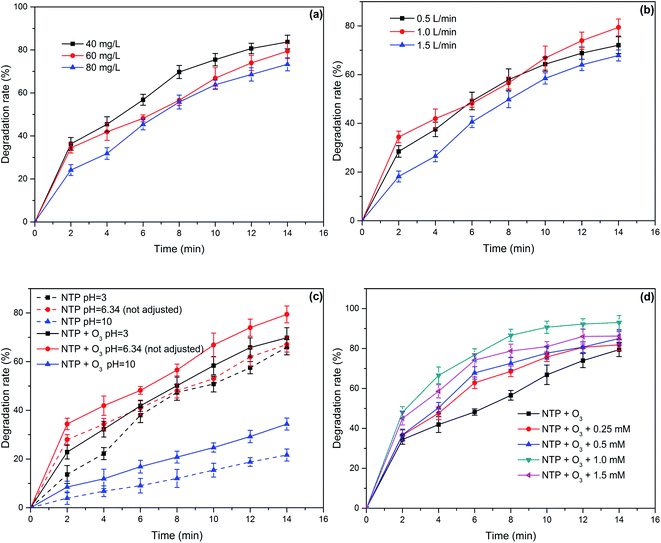 |
| Fig. 3 Effect of degradation conditions on chloroxylenol removal in NTP/O3 system. (a) The initial concentration of chloroxylenol, (b) the flow rate of oxygen, (c) the initial pH of solution, (d) the addition of H2O2. | |
3.3 Effect of oxygen flow rate
In the NTP/O3 system, the amount of activated species and the mass transfer of the activated species could be affected by gas flow rate.23 In order to illustrate the effect of gas flow rate on the PCMX degradation in the NTP/O3 system, the degradation of PCMX under different g flow rates was investigated. The other discharge parameters were consistent with the parameters listed in Section 3.1. The removal efficiency of PCMX at different oxygen flow rates are presented in Fig. 3(b). The degradation efficiency of PCMX showed no significant change when the flow rate increased from 0.5 L min−1 to 1.0 L min−1, (degradation efficiency of 89.1% and 79.4%, respectively). However, the degradation efficiency decreased to 67.9% when the flow rate was increased from 1.0 to 1.5 L min−1. During the NTP oxidation process, the active species were produced in the gas phase, and then transferred into the aqueous solution to react with PCMX molecules. As reported, in a certain flow rate range, the increase in the flow rate is beneficial to the mass transfer of the active species, which generated in the gas phase and transferred to the liquid phase promoting the degradation of the organic pollutants. However, when the flow rate is increased to a certain value, the mass transfer balance of the active species between the gas–liquid two phases will be destroyed. The residence time of the active species in the system will also be shortened.24,25
3.4 Effect of initial solution pH
The pH of the solution is a vital parameter for the gas–liquid discharge plasma process, because the pH affects the existing state of organic compounds and the generation of activated species.26 The active species' redox potential can also be influenced by the solution's pH. When O3 is involved in the degradation reaction, the solution's pH has an important influence on the chemical stability of O3. Especially in alkaline environment, O3 is more likely to decompose to form ˙OH. Therefore, it is necessary to investigate the effect of pH on the degradation rate of PCMX in NTP-only and NTP/O3 systems. The selected initial pH values of the PCMX aqueous solution were 3.0, 6.34 (not adjusted) and 10.0. The other discharge parameters were consistent with the parameters listed in Section 3.1. The PCMX degradation efficiencies with different pH values in the two systems are shown in Fig. 3(c). Under different initial pH conditions, the degradation rates of PCMX in the NTP/O3 system were higher than those of the NTP-only system. This was mainly due to the addition of aeration devices prolonging the residence time of O3 and promoting its mass transfer of ozone. In order to investigate these results, deionized water with different initial pH was used as discharge solutions. Compared with the NTP-only system, the concentration of O3 in aqueous solution in the NTP/O3 system was significantly increased under the same discharge conditions as shown in Fig. S3.† Especially under weak acid and alkaline conditions, the concentration of O3 in aqueous solution increased by 1.9 times and 3.3 times, respectively.
Regardless of the type of reaction system, the highest PCMX degradation rate was obtained in acidic or weakly acidic solution, and a lower PCMX removal rate occurred in alkaline environment within 14 min of plasma discharge. For example, the PCMX removal rates with initial pH of 3.0, 6.34 and 10.0 were 65.9%,69.8% and 34.7% after 14 min of treatment in the NTP/O3 system, respectively. This phenomenon could be explained by the fact that ·OH radical would be annihilated by OH− ions in a relatively higher pH environment.24,27 On the other hand, with the mineralization of organic matter in the alkaline solution, carbonate and bicarbonate could be formed, which are scavengers of ˙OH, as shown in eqn (5)–(7).28,29
|
CO32− + ·OH → CO3˙− + OH−
| (5) |
|
HCO32− + ·OH → CO3˙− + H2O
| (6) |
|
·OH + OH− → O˙− + H2O
| (7) |
3.5 Effect of the addition of H2O2
Owing to the special characteristics of H2O2, it does not significantly react with most organic compounds.30 However, H2O2 can be excited by UV light or high-energy electrons to generate ˙OH, which promotes the degradation of organic compounds, as shown in eqn (8)–(10). In order to evaluate the effect of the addition of H2O2 on the degradation ratio of PCMX in the NTP/O3 system, the removal efficiency of PCMX at different H2O2 concentrations were researched and the results are depicted in Fig. 3(d). As demonstrated in Fig. 3(d), the degradation ratio increased with the treatment time, and the performance of NTP/O3/H2O2 was much more superior than that of NTP/O3 with the same discharge conditions.
Compared with the NTP/O3 system, when the concentration of H2O2 increased from 0.25, 0.50 to 1.00 mM, the PCMX degradation rate increased from 81.8%, 85.1% to 93.1%, respectively. However, it decreased to 86.1% at the concentration of H2O2 concentration of 1.5 mM with the same treatment time. These results demonstrated that there was higher synergistic effect when H2O2 was added to the NTP/O3 system. During plasma discharge, H2O2 can be excited by high-energy electrons, UV light, or reacted with O3 in an aqueous solution to form ˙OH with higher redox potential. On the other hand, when the H2O2 exceeds a certain concentration, the degradation efficiency of PCMX was decreased. The main reason for this might have been that when excessive H2O2 reacts with hydroxyl radicals,
, which has lower oxidation, is formed, as shown in eqn (11).31
|
H2O2 + e− → OH− + ·OH
| (8) |
|
 | (9) |
|
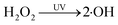 | (10) |
|
 | (11) |
3.6 Effect of the radical scavenger addition on chloroxylenol removal
TBA is a typical radical scavenger that has a higher reaction constant (6 × 108 M−1 s−1) with ˙OH than that (3 × 10−3 M−1 s−1) with O3.22 To investigated the contribution of ˙OH to the degradation of PCMX, TBA was added as the scavenger of ˙OH. In this study, the different concentrations of TBA with 1.0, 0.5 and 0.25 mM were used, the results are shown in Fig. 4. It was observed that the degradation rate of PCMX decreased significantly with the increase of TBA concentration during 14 min of plasma treatment, which further demonstrated the production of ˙OH. The degradation rate was 79.4% after 14 min of reaction without TBA, and it was diminished to 47.1%, 43.8% and 34.8% after adding 1.0, 0.5 and 0.25 mM TBA, respectively. Correspondingly, the kinetic constants decreased from 0.0919 to 0.0404, 0.0375 and 0.0360 mg−1, as shown in Fig. S4.† The experimental results suggested that ˙OH played a significant role in PCMX degradation in the NTP/O3 oxidation system.
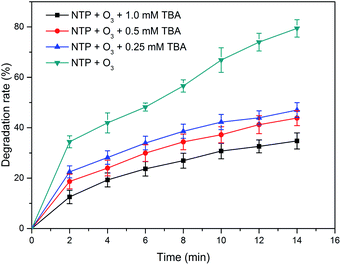 |
| Fig. 4 Effect of radical scavenger addition on chloroxylenol degradation in NTP/O3 system. | |
3.7 Degradation of PCMX in secondary effluent
In order to evaluate the potential of the NTP/O3 system for the degradation of PCMX with actual wastewater, the PCMX solution with a concentration of 60 mg L−1 with deionized water (DW) and secondary effluent (SE) was prepared. SE used in this research was collected from a wastewater treatment plant (WWTP) in Beijing. The main water quality characteristics of SE are listed in Tab. S1.†
Under the same discharge conditions, the experimental results are presented in Fig. 5. The results obtained clearly showed that compared within DW, the degradation rates of PCMX in SE were inhabited. The degradation rate was reduced from 79.43% to 65.26%. This inhibition can be mainly attributed to the fact that (a) dissolved organic matter (DOM) in SE could compete for active species with PCMX, and radicals would be captured by NOM because of its electron-rich sites, its second-order rate constant (108 to 109 M−1 s−1), (b) some inorganic anions in the SE such as CO32−, HCO3−, Cl−, which could act as scavengers of active species, and (c) high conductivity has an impact on the plasma discharge channel, which was not conducive to the production of active species.31
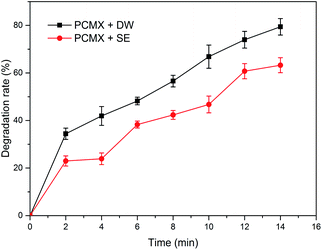 |
| Fig. 5 Degradation of PCMX in deionized water (DW) and secondary effluent (SE). | |
3.8 Possible degradation pathway
After 14 min of treatment in the NTP/O3 oxidation process, the PCMX and its intermediates were detected by GC-MS. As reported, hydroxylated products can be produced by the electrophilic attack of on the aromatic ring of PCMX.32 According to the results obtained by GC-MS and prior reports, a possible degradation pathway for PCMX in the NTP/O3 process was proposed, as shown in Fig. 6. It was observed that the generation of 2,6-dimethylhydroquinone might have been the first step of the degradation of PCMX, and the Cl position of PCMX was replaced by an OH group to form a dechlorinated aromatic product, followed by dehydrogenation, aromatic ring oxidation and mineralization of other by-products. Mass spectrograms of the intermediates are illustrated in SM Fig. 5. A similar pathway of PCMX degradation was reported by Skoumal et al.7 with PCMX removal by electrochemical oxidation processes and Sun et al.33 through thermally activated persulfate. During the NTP/O3 treatment of PCMX, the pH of the solution decreased from 6.34 to 4.02 in 15 min. This result confirmed that some simple short-chain carboxylic acids might have been generated as degradation intermediates of PCMX. Song's studies suggested that there were formic acid, maleic acid, oxalic acid or acetic acid generated during the treatment of PXMC by O3 in combination with UV irradiation.8
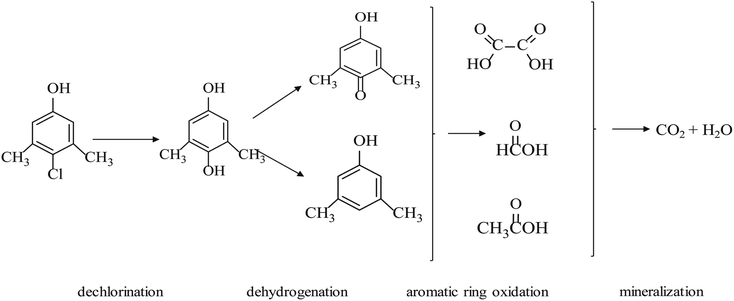 |
| Fig. 6 Possible degradation pathway of chloroxylenol by the NTP/O3 system. | |
3.9 Estimation of intermediate toxicity during PCMX degradation
PCMX has a little toxic effect on mammalian organisms.34 However, it exhibits different biological toxicity to aquatic organisms. It is necessary to investigate the changes in toxicity of PCMX and its intermediates.4,35 In this study, the toxicity of PCMX aqueous solution with 0, 2, 6, 10, and 14 min treatment by the NTP/O3 process was evaluated by luminescent bacteria based on the determination of the inhibition of the bioluminescence of the bacteria.
Without dilution, the inhibition rate of bioluminescence activity of the PCMX solution before (60 mg L−1) and after (12.3 mg L−1) NTP/O3 treatment was 99–100%. In order to accurately determine the change in toxicity change of the PCMX aqueous solution during treatment, all water samples were diluted 20 times. The pH of samples was adjusted to approximately 7 by 0.1 M NaOH before the test, thereby avoiding the effects of pH on microbial activity. As illustrated in Fig. 7, the bioluminescence inhibition ratio increased with the treatment time (from 32% to 50%) during the first 12 min of the PCMX NTP/O3 oxidation, and decreased to 37% after 14 min. This result could be explained by the fact that during the NTP/O3 degradation process, more toxic intermediates were produced, such as 3,5-dimethylphenol. The main components and intermediates were assessed by QSAR with the help of the software of the ECOSAR program. The results of ECOSAR based on fish, daphnia and green algae reflected the toxicity level of the target compound, which was used in this study to demonstrate the toxicity of PCMX and its four major by-products, as shown in Tal. S2.† Compared with that of the initial compounds of PCMX, the toxicity of 2,6-dimethylhydroquinone and 2,6-dimethylbenzoquinone was decreased, however, the toxicity of 3,5-dimethylphenol was increased.
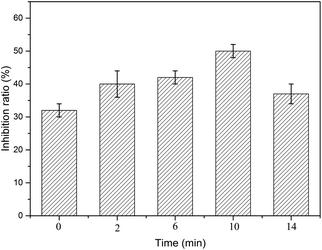 |
| Fig. 7 Change in toxicity during the chloroxylenol degradation by the NTP/O3 oxidation process. | |
4 Conclusions
Gas–liquid discharge non-thermal plasma coupled with ozonation reactor was proved effective for the removal of PCMX in aqueous solution, which exhibited an obvious synergistic enhancement rather than a single simple additive effect from the combination of plasma and ozonation. Within a certain range, lower initial concentration, lower oxygen flow rate, acidic or weakly acidic condition and addition of H2O2 could improve the degradation rate of PCMX in the NTP/O3 system. The presence of radical scavenger significantly inhibited the removal of PCMX, which proved that ˙OH played a significant role in PCMX degradation in the NTP/O3 system. The main components and intermediates were detected and analyzed with GC-MS, the possible reaction pathways and mechanisms were also proposed. Through bacterial inhibition test and ECOSAR software evaluation, more toxic intermediate products were detected.
Author contributions
Keke Ma contributed to the conception of the study and performed the experiment and the data analyses and wrote the manuscript; Lu Zhou, Yu Bai, Yiying Xin, Mingru Chen contributed to analysis and manuscript preparation; Heping Li, Yucheng Bao and Yuexi Zhou helped perform the analysis with constructive discussions.
Conflicts of interest
There are no conflicts to declare.
Acknowledgements
This work was supported by the National Natural Science Foundation of China (No. 51578309).
Notes and references
- M. S. Kostich, A. L. Batt and J. M. Lazorchak, Environ. Pollut., 2014, 184, 354–359 CrossRef CAS PubMed.
- R. Xu, P. Zhang, Q. Wang, X. Wang, K. Yu, T. Xue and X. Wen, Sep. Purif. Technol., 2019, 212, 299–306 CrossRef CAS.
- N. Bolong, A. F. Ismail, M. R. Salim and T. Matsuura, Desalination, 2009, 239, 229–246 CrossRef CAS.
- E. Capkin, T. Ozcelep, S. Kayis and I. Altinok, Chemosphere, 2017, 182, 720–729 CrossRef CAS PubMed.
- J. T. Yu, E. J. Bouwer and M. Coelhan, Agric. Water Manag., 2006, 86, 72–80 CrossRef.
- M. Brycht, A. Leniart, J. Zavašnik, A. Nosal–Wiercińska, K. Wasiński, P. Półrolniczak, S. Skrzypek and K. Kalcher, Electrochim. Acta, 2018, 282, 233–241 CrossRef CAS.
- M. Skoumal, C. Arias, P. L. Cabot, F. Centellas, J. A. Garrido, R. M. Rodriguez and E. Brillas, Chemosphere, 2008, 71, 1718–1729 CrossRef CAS PubMed.
- S. Song, Z. Liu, Z. He, Y. Li, J. Chen and C. Li, Chemosphere, 2009, 77, 1043–1051 CrossRef CAS PubMed.
- S. Tang, D. Yuan, Y. Rao, J. Zhang, Y. Qu and J. Gu, J. Environ. Manage., 2018, 226, 22–29 CrossRef CAS PubMed.
- N. Jiang, L. Guo, C. Qiu, Y. Zhang, K. Shang, N. Lu, J. Li and Y. Wu, Chem. Eng. J., 2018, 350, 12–19 CrossRef CAS.
- H. Xu, R. Ma, Y. Zhu, M. Du, H. Zhang and Z. Jiao, Sci. Total Environ., 2020, 703, 134965 CrossRef CAS PubMed.
- C. Zhang, L. Gu, Y. Lin, Y. Wang, D. Fu and Z. Gu, J. Photochem. Photobiol., A, 2009, 207, 66–72 CrossRef CAS.
- S. K. Pankaj, Z. Wan, W. Colonna and K. M. Keener, Water Sci. Technol., 2017, 76, 567–574 CrossRef CAS PubMed.
- M. Markovic, M. Jovic, D. M. Stankovic, V. V. Kovacevic, G. Roglic, G. Gojgiccvijovic and D. Manojlovic, Sci. Total Environ., 2015, 505, 1148–1155 CrossRef CAS PubMed.
- H. Lee, Y. K. Park, J. S. Kim, Y. H. Park and S. C. Jung, Environ. Res., 2019, 169, 256–260 CrossRef CAS PubMed.
- Y. Zhang, H. Wei, Q. Xin, M. Wang, Q. Wang, Q. Wang and Y. Cong, J. Environ. Manage., 2016, 183, 726–732 CrossRef CAS PubMed.
- T. Mitrovic, S. Lazovic, B. Nastasijevic, I. A. Pasti, V. Vasic and T. Lazarevic-Pasti, J. Environ. Manage., 2019, 246, 63–70 CrossRef CAS PubMed.
- P. Vanraes, G. Willems, N. Daels, S. W. H. Van Hulle, K. De Clerck, P. Surmont, F. Lynen, J. Vandamme, J. Van Durme, A. Nikiforov and C. Leys, Water Res., 2015, 72, 361–371 CrossRef CAS PubMed.
- H. Bader and J. Hoigné, Water Res., 1981, 15, 449–456 CrossRef CAS.
- Z. Yu, Y. Sun, G. Zhang and C. Zhang, Chem. Eng. J., 2017, 317, 90–102 CrossRef CAS.
- H. Liu, P. Sun, H. Liu, S. Yang, L. Wang and Z. Wang, Chemosphere, 2015, 135, 182–188 CrossRef CAS PubMed.
- J. Wu, L. Ma, Y. Chen, Y. Cheng, Y. Liu and X. Zha, Water Res., 2016, 92, 140–148 CrossRef CAS PubMed.
- M. Magureanu, N. B. Mandache and V. I. Parvulescu, Water Res., 2015, 81, 124–136 CrossRef CAS PubMed.
- S. Tang, D. Yuan, Y. Rao, N. Li, J. Qi, T. Cheng, Z. Sun, J. Gu and H. Huang, Chem. Eng. J., 2018, 337, 446–454 CrossRef CAS.
- B. Sun, M. Gao, M. Arowo, J. Wang and S. Lei, Ind. Eng. Chem. Res., 2014, 53, 19071–19076 CrossRef CAS.
- M. Markovic, M. Jović, D. Stanković, J. Mutic, G. Roglic and D. Manojlovic, Pol. J. Environ. Stud., 2014, 23, 2103–2109 CAS.
- S. Mededovic and B. R. Locke, Ind. Eng. Chem. Res., 2007, 46, 2702–2709 CrossRef CAS.
- Y. Xiong, C. He, H. T. Karlsson and X. Zhu, Chemosphere, 2003, 50, 131–136 CrossRef CAS PubMed.
- K. C. Hsieh, R. J. Wandell, S. Bresch and B. R. Locke, Plasma Processes Polym., 2017, 14 Search PubMed.
- B. Jiang, J. Zheng, S. Qiu, M. Wu, Q. Zhang, Z. Yan and Q. Xue, Chem. Eng. J., 2014, 236, 348–368 CrossRef CAS.
- Y. Liu, X. He, Y. Fu and D. D. Dionysiou, Chem. Eng. J., 2016, 284, 1317–1327 CrossRef CAS.
- Y. Zhang, M. Zhou, X. Hao and L. Lei, Chemosphere, 2007, 67, 702–711 CrossRef CAS PubMed.
- Y. Sun, J. Zhao, B.-T. Zhang, J. Li, Y. Shi and Y. Zhang, Chem. Eng. J., 2019, 368, 553–563 CrossRef CAS.
- L. J. Yost, J. D. Rodricks, D. Turnbull, P. C. DeLeo, J. F. Nash, A. Quinones-Rivera and P. A. Carlson, Regul. Toxicol. Pharmacol., 2016, 80, 116–124 CrossRef CAS PubMed.
- J. Wang, D. Zhi, H. Zhou, X. He and D. Zhang, Water Res., 2018, 137, 324–334 CrossRef CAS PubMed.
Footnote |
† Electronic supplementary information (ESI) available. See DOI: 10.1039/d1ra01886h |
|
This journal is © The Royal Society of Chemistry 2021 |