DOI:
10.1039/D0RA08727K
(Review Article)
RSC Adv., 2021,
11, 12915-12928
Current advances in ultrasound-combined nanobubbles for cancer-targeted therapy: a review of the current status and future perspectives
Received
13th October 2020
, Accepted 16th March 2021
First published on 6th April 2021
Abstract
The non-specific distribution, non-selectivity towards cancerous cells, and adverse off-target side effects of anticancer drugs and other therapeutic molecules lead to their inferior clinical efficacy. Accordingly, ultrasound-based targeted delivery of therapeutic molecules loaded in smart nanocarriers is currently gaining wider acceptance for the treatment and management of cancer. Nanobubbles (NBs) are nanosize carriers, which are currently used as effective drug/gene delivery systems because they can deliver drugs/genes selectively to target sites. Thus, combining the applications of ultrasound with NBs has recently demonstrated increased localization of anticancer molecules in tumor tissues with triggered release behavior. Consequently, an effective therapeutic concentration of drugs/genes is achieved in target tumor tissues with ultimately increased therapeutic efficacy and minimal side-effects on other non-cancerous tissues. This review illustrates present developments in the field of ultrasound-nanobubble combined strategies for targeted cancer treatment. The first part of this review discusses the composition and the formulation parameters of NBs. Next, we illustrate the interactions and biological effects of combining NBs and ultrasound. Subsequently, we explain the potential of NBs combined with US for targeted cancer therapeutics. Finally, the present and future directions for the improvement of current methods are proposed.
1. Introduction
Cancer is a major health issue worldwide. The global demographic forecasts have projected increasing cases of cancer in the coming decades, with more than 400 million new cases expected annually by 2025.1 In 2017, the USA saw 1.6 million new cases and more than 600
000 cancer deaths were reported.2 Furthermore, 26 million new cancer cases with 17 million deaths globally are forecasted by 2030.3 Existing cancer therapies require surgery, chemotherapeutic medicines, and radiation.4 However, a huge drawback affecting the success of the majority of commonly used antitumor agents is their non-selectivity or non-specificity against cancer cells. This has culminated in these medications causing significant adverse effects on sensitive tissues and organs. The therapeutically active accumulation of medication in the tumor tissue is achieved in cancer therapy at the risk of infection throughout the host body. The non-selective or non-specific treatment of tumor cells and tissues generates toxicological problems, which hinder effective chemotherapy.5 Besides, because of their hydrophobicity, most anticancer medications have low aqueous solubility. This contributes to a lower extraction from biological membranes, and thus reduces their therapeutic effectiveness.6 Thus, to effectively cure and control cancer, it is extremely important to administer anti-cancer drugs specifically and exactly to cancer tissues at enhanced concentrations and to improve their aqueous solubility.
To boost the regulated local release of drugs, strategies involving thermal and mechanical methods such as ultrasound (US)7 have been recently adopted. Considering its noninvasive nature, universal availability, and function of real-time image monitoring in the medical field, the application of ultrasound is greatly appealing. The idea of looking in-depth at what happens at the nanoscale in liquid and colloidal systems has opened up a new arena in the medically related physical–chemical research market. Current studies range from studying the nanobubble (NB) forming processes to investigating their characteristics and potential applications.8 NBs, which are submicron bubbles usually having a diameter in the range of 150–500 nm,9 are an enticing scene for the distribution of therapeutics and have demonstrated improved cancer aggregation and retention compared to microbubbles (MBs).10,11 The synthesis and characterization of NBs using microfluidics12 have previously been recorded using other approaches including sonication and mechanical agitation.13–15 In recent years, NBs have shown exceptional stability,12,16,17 which has increased their use in various biomedical applications.18,19 NBs offer US contrast augmentation, and hence possess significant potential for diagnostic applications.20 Moreover, they are often used either to mediate or cause therapeutic effects.21 Recently, they have also been employed for the transmission of medications, chromosomes, and gas.22,23 NBs can be considered an additional group of US-sensitive nanocarriers that seek to enhance efficiency in vivo. Attractively, NBs fold the route for extravasation into adjacent tissues from the blood vessels, thus increasing the transmission capacity and localization.24 They can be accumulated inside tumor tissues by the increasing retention and permeability effect,25 by targeting, or by attaching antibodies to their surfaces.26 When combined with US, NBs may be used to improve the selective distribution of medication to the target tissues. They may be used for US-induced sonoporation as therapeutic cavitation nuclei, inducing the development of temporary apertures in cell membranes to change the permeability of cells. Consequently, NBs can be directed with a drug or medication may be incorporated inside the NB shell, functioning as a US-mediated cargo to promote product intracellular uptake.27 These techniques have been used to establish tailored delivery systems coupled with ultrasonography for different tissues. Besides, it is remarkable that the bubble-targeted approach may allow the imaging of the distribution and delivery of the loaded material via real-time ultrasonic imaging.28
During the last few years, the use of US for cancer diagnostic and treatment has attracted immense scientific attention. Combining the applications of US and NBs has recently emerged as an interesting field of research for targeted cancer therapy, thus avoiding the off-target effects of anticancer drugs or other therapeutic agents with ultimately improved therapeutic efficacy. This review aims to summarize the current developments in the field of US-NB combined strategies for targeted cancer treatment.
2. Structure and composition of NBs and their preparation and fabrication
There are two major types of NB materials with separate physicochemical properties, which are used to construct the two distinct parts of NBs known as the inner center and the outer layer. The membrane of the outer layer consists primarily of surfactants, polymers, and enzymes, while the inner core includes sulfur hexafluoride and perfluorocarbons (Fig. 1). For the composition of the NBs, regulatory bodies have agreed on the selection of biocompatible, and biodegradable substances.29,30
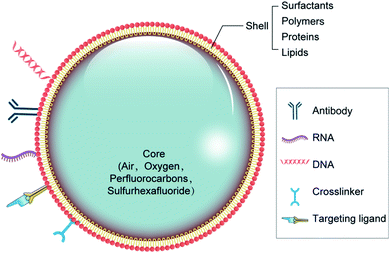 |
| Fig. 1 Structure and possible composition of nanobubbles. | |
The shell structure is a crucial element, which requires various technical considerations during the formulation of bubbles. The NB shell consists primarily of polymers or lipids for improving protection against gas leakage, breakdown, and NB coalescence, and reducing the surface tension contributes to its stability.31 Next, the shell structure may have a substantial outcome on the life of the bubble because it influences the flow of gas from the center to the outer layer. The shell thickness and elasticity also affects the durability of NBs (Table 1).32 As a feature of the NB structure, the acoustic parameters to cause drug release can differ. Notably, the viscoelastic properties of the shell greatly affect the reaction of NBs inside an acoustic field.33 Because of their rapid contraction and extension, exposure to US energy will contribute to the rapid destruction of bubbles by cavitation.
Table 1 Main components of NBs shell
Nature of material |
Shell composition |
Reference |
Surfactants |
TWEEN® |
34
|
SPAN® |
14
|
Proteins |
Avidin |
35
|
Albumin |
36 and 37 |
Lysozyme |
38
|
Polysaccharides (dextran sulfate, dextran, chitosan, glycol chitosan, hyaluronic acid, DEAE dextran) |
39–42
|
Cellulose |
43
|
Pluronics |
44
|
PEG composites (mPEG-PLLA, mPEG-PCL, PEO, PEG-PBCA, mPEG-PLGA) |
45 and 46 |
Lipids |
Phospholipids (DSPC, DPPC, DMPC, DPPA, DPPG, DPP), DSPE-PEG2000, DSPE-PEG2000-biotin |
47–49
|
The shell of NBs helps to reduce the surface tension, which is the most important mechanism responsible for their stability. NBs made up of lipids with long acyl chains exhibit a higher shelf life.50 An approach to improve the resilience of mono-layer-based phospholipid bubbles is to attach polymers to the formulations. For nanobubbles, the effect of an external polymer coating on bubble formation has been commonly studied. Rapoport et al.46 established NBs with a polymer shell utilizing poly(vinyl alcohol) and then increased their stability compared with industrial bubbles with a phospholipid shell such as PEG, PCL, PLGA, composites, polysaccharides, and Pluronics. Cross-linking of the shell, whether chemical or physical, provides another solution to developing NB structures that are secure during US. Chemical cross-linking has been reported to endow polymeric bubbles with stability and elasticity.51 For example, for theranostic purposes, glycol chitosan polymer NBs connected to tri-polyphosphate were established, showing improved stability with time.52
The fabrication of NBs has been documented in several experiments. Nanobubbles consisting of a phospholipid shell and a gas center are known to have optimum contrast enhancement potential. However, the preparation of NBs with a uniform sized has been challenging, where a few studies have shown that the centrifugal state is the main factor influencing the nanobubble diameter,53 but others have suggested that the thickness of the phospholipid film is an important factor in determining the diameter of NBs.54 Herein, we summarize some key factors and studies for the preparation of NBs.
Two main techniques can be considered for the generation of NBs, namely, modification of formulated microbubbles and formulation of nanoscale structures ab initio. Previous processes involve rotating, filtration, condensation, and centrifugation. Using another process, NBs are prepared mainly via thin-layer evaporation, emulsification, sonication, mechanical stirring, and coacervation or coalescence.55 Polymeric NBs were produced by ultrasonics while employing a combination of 40 stearates and polyoxyethylene Span 60, and thus a population of unimodally distributed nano-sized bubbles was produced.14,56
A further approach for creating polymer-based NBs is to layer monodisperse polymer NBs on the shell of surfactant-stabilized NBs when mixed to form a dense thick coat.49 The creation of NBs using microfluidic systems has previously been explored because microfluidics allows the exact estimation of bubble diameter and rate of production via the intervention of liquid flow rate, gas pressure, and system geometry.57–59 Rapoport et al.,46,60 taking advantage of the idea of phase-shift perfluorocarbon (PFC) nanoemulsions for NB processing, produced an amphiphilic block copolymer-stabilizing phase-shift perfluorocarbon nanoemulsions. PFC compounds such as perfluorobutane were injected into solutions of micellar amphiphilic block copolymer (i.e., PEGPLLA or PEG–PCL) and refluxed to form shape-stable NBs in the block copolymer. Another concept based on PFC nanoemulsions has also been employed for the preparation of NBs. The size of the NBs is adjusted by optimizing the polymer composition and the liquid–polymer ratio.61 Indeed, at the interface of NBs, they may be viewed as a hybrid framework, comprising a monolayer of surfactant/cosurfactant, covered by a polymer shell for enhancing their stability.
They are designed based on the principle of adsorption of charged polymers through monolayers with phospholipids. Scalable processing and preparation technologies for NBs are currently under study. Centrifugal forces may cause large-scale droplets and bubbles to form in the spinning liquid. Zhang et al. reveal that centrifugation was easier for the particle size and homogeneity of NBs than controlling the thickness of the phospholipid. Furthermore, 70g may be a reasonably appropriate centrifugal velocity for the preparation of pure NBs, which has a standardized size distribution, remarkable passive targeting capacity for tumor tissues, and potential for treatment.62
3. Biological effects and interactions on combining NBs with ultrasound
With the development of ultrasonic medicine, biotechnology, and nanobubble contrast agents, ultrasound irradiation combined with NBs has attracted wide interest in clinical cancer treatment, where the biological effects and interactions on combining NBs with ultrasound in tumors have become problems to be investigated.
3.1 Biological alterations in tumor tissue play a vital role in the efficiency of NBs
The microvascular endothelial gap is dense and the structure of endothelial cells is complete in normal tissue, whereas in solid tumor tissues, the neovascular endothelial pores are between 380 nm and 780 nm, and the structural integrity of endothelial cells is poor. Therefore molecules or particles of certain sizes tend to gather in tumor tissues more than in normal tissue,63 as shown in Fig. 2A. This phenomenon is called the enhanced permeability and retention (EPR) effect, which is considered to be the mechanism of completing passive targeted therapy of tumor tissues.64 In preclinical trials, drugs or gene delivery-targeted systems based on EPR have shown significant progress in anticancer efficacy compared with traditional chemotherapy.65 A variety of nanomaterials based on the EPR effect have been applied in the past few years, where the sizes of the nanobubbles at the nanoscale can be transformed on account of the sizes of the pores in tumor vessels. Given that different gap sizes exist in endothelial cells for different categories of tumors, a suitable size nanomaterial must be established based on the category of the tumor. Also, the obstruction generated by biological barriers when nanoparticles reach the blood circulation system requires high attention.66 Thus, considering these challenges, to better take advantage of the EPR effect in nanomaterial delivery, various means of treatment have been designed. EPR-based nanoparticle-targeting strategies are primarily committed to adjusting the size of drugs or vectors and/or utilizing ligands that link molecules involving the EPR effect.67
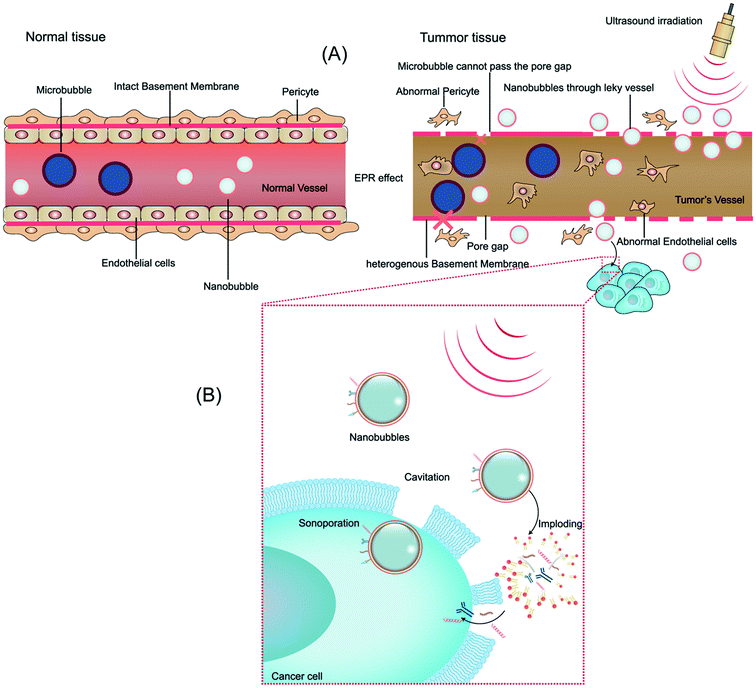 |
| Fig. 2 Schematic illustration showing the effect and interactions of biological factors on ultrasound combined with nanobubbles and description of several mechanisms via NBs for the delivery of drugs/genes to tumor tissues. (A) Mechanism of EPR effect in tumor and normal tissues, where microbubbles cannot pass through the endothelial pore but nanobubbles can in tumor tissues. (B) Biological effect on combining ultrasound with NBs and improved permeability of the cancer cell membrane, concurrently making NBs implode. | |
3.2 Biological effects by ultrasonic exposure combined with NBs
Ultrasonic delivery technology is based on the biophysical process of cell poration by ultrasound combined with NBs and this process is known as sonoporation. Compared with other nanoparticles, NBs have the special property of “collapsing” under insonation by ultrasound energy, resulting in the implosion of NBs and changes in the permeability of the cell membrane.68 When the ultrasonic energy is sufficiently increased, the effect of “ultrasonic cavitation” occurs, that is, the bubbles (cavitation nuclei) in the liquid vibrate and grow, constantly accumulating energy from the acoustic field and collapsing until the energy reaches a certain threshold. Ultrasonic irradiation causes ultrasonic cavitation, leading to gaps in the cell membrane with a diameter of about 300 nm, and stable cavitation is characterized by repetitive, non-collapsing oscillations of nanobubbles, resulting in local flow and shear stress on nearby cells, thus increasing the permeability of blood vessels.69 Furthermore, ultrasonic irradiation is capable of producing thermal and mechanical therapeutic effects. Heating is generated by the mechanical friction of the tissues moving with the passing of the ultrasonic wave. As early as 1987, Dyson noted that tissue must reach a certain temperature from 40 °C to 45 °C for more than 5 min to have a therapeutic effect in nature. The study conducted with nonperfused tissues illustrated that ultrasound irradiation increased the tissue temperature at a rate of 0.86 °C min−1 (1 MHz, 1 W cm−2).70 Nevertheless, numerous questions remain unanswered about the thermal effects of ultrasound and its role in drug delivery. The biological effect of ultrasound irradiation can increase the permeability of the cell membrane, induce gene transfer, improve the intracellular drug concentration, embolize tumors, nourish vessels, and overcome tissue barriers, developing a crucial targeting role.71 Moreover, there are numerous other biological interactions that need to be considered for modulating NBs and US for therapeutic delivery to tumors. We will briefly discuss them in the following sections (Fig. 2B).
4. Formulation of targeted ultrasound combined NBs for targeted cancer therapy
Recently, specific nanocarriers have gained enormous popularity as an important means of drug and gene distribution owing to their properties. Further, they are capable of attaining the maximum concentration of cytotoxic agents in a site-specific manner. Improving the biodistribution and pharmacokinetics of pharmaceutical drugs through targeted delivery via nanocarriers has resulted in improved therapeutic efficacy and less adverse effects on other healthy and non-targeted tissues.72,73 Currently, various targeting strategies are being exploited for the targeted delivery of antitumor medication through nanocarriers. For example, the use of different antibody-conjugated NBs that bind ligands in cancer cells and tissues has been a highly effective anticancer drug-targeted delivery approach. NBs may be combined with Affibody® as a directed probe in the manufacture of nano-sized ultrasonic contrast agents.74,75 In another case, a method of thin-film hydration used to control the thickness of phospholipid films was employed to prepare normal phospholipid-shelled and gas-coded NBs on the nanoscale. Subsequently, the NBs were combined with biotinylated anti-ErbB2 molecules, a developed strong affinity shape, ultra-small protein, exhibiting tumor sensitivity for over-expressed human epidermal growth factor receptor type 2 (HER2) cells. In both the in vivo and in vitro tests, the strong specificity of the NB Affibody ligands for HER2-overexpressing cells was observed, and the successful US improvement in HER2-positive cancer imaging was achieved.74 Ultrasonic NBs equipped with unique anti-PSMA (prostate antigen) nanobodies were fabricated to promote the imaging of prostate cancer through a biotin–streptavidin system.76
4.1 Ultrasound combined with NBs for the delivery of anticancer drugs
The synthesis of drug-associated NBs combined with US was initially suggested for cancer chemotherapy. Suzuki et al.77 reported the use of NBs in conjunction with US to permeabilize 4 forms of tumor cells and to enhance the activity of antitumor drugs. Through the use of NBs and US, the cell susceptibility to cisplatin and 5-FU was successfully improved. Oxygen-trapping NBs may be directed by US to increase the localized effectiveness and targeting for reversal of hypoxia in non-muscle-invasive bladder cancer (NMIBC) tumors. The ability to accurately direct NBs loaded with a payload utilizing non-invasive and stable ultrasonic imaging navigation provides tremendous potential in engineering controlled cargo distribution and reprogramming hypoxia for a broad variety of therapeutic applications.78 Selective apoptosis and growth inhibition in HepG2 cells were effectively achieved via the controlled and drug-laden degradation of NBs through the application of the US. Apatinib-loaded and liver cancer homing peptide (GPC3)-targeted NBs in conjunction with US can be considered a new chemotherapeutic strategy for treating liver cancer.79
NBs carrying doxorubicin (DOX) demonstrated outstanding chemotherapeutic agent encapsulation functionality and potential to kill cancer cells upon application of US. US irradiation combined with DOX-loaded NBs completed the delivery of DOX into tumor cells in vitro. Additionally, NBs carrying DOX efficiently distributed DOX to tumor cells in mammals. Chitosan NBs are ideal materials for the ultrasound-sensitive delivery of DOX, and hence are successful materials for non-invasive and selective drug delivery.80 Perfluorocarbon NBs are innovative nanocarriers that can vaporize in situ by US for transformation into micron-scale gas bubbles. The micron-size gas bubbles will interact intensely with ultrasonic irradiation after PFC nanodroplet vaporization, and the bubbles can be applied to imaging and therapy in tumor tissues. Gao et al.81 developed an NB polymeric device to increase the sensitivity of doxorubicin-related tumor cells when combined with the application of US. The NBs transformed into nano/microbubbles after heating to physiological levels and then the DOX-charged NBs dropped, freeing the encapsulated medication and improving the in vivo cancer treatment effectiveness.81
Rapoport et al.46 utilized polymeric micelles for the encapsulation of doxorubicin-containing liquid perfluoropentane (PFP). Increasing the temperature to 37 °C for this device permitted the PFP nanodrops to vaporize and turn into bigger size bubbles. This indicated that the tumor-irradiated US could make nanobubbles become bigger bubbles, which are several times larger than the droplet size by the coalescence of the vaporized nanodrops and thermodynamic effects after phase transition. They revealed the capability of polymer-stabilized PFP NBs to transfer doxorubicin to xenotransplanted tumors and illuminated that this approach can restrain tumor proliferation.46 In another study, the amphiphilic chain was stabilized by PFP NB copolymers for the transport of paclitaxel. Interesting outcomes were observed both in vitro and in vivo in tumor cells. Systemic delivery PFP nanoemulsions carrying paclitaxel combined with 1 MHz US led to the positive breast, ovarian and pancreatic regression of a tumor.60 The studies by Rapoport et al. suggested that the droplet-to-bubble movement and the oscillation of bubbles lead to the release of the loaded therapeutic agents and improve their intracellular absorption. The proposed approach for transferring US-mediated drugs by polymer-linked perfluorocarbon NBs is based on the transition from droplet to bubble, as seen in Fig. 3.82 This is supposed to support the delivery of the capsulated medication, where under US activity, the medication will be that will ‘ripped off’ from the surface of the droplet.
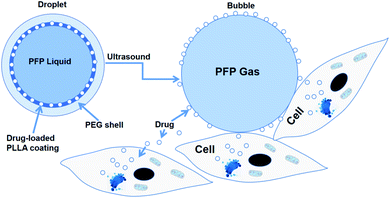 |
| Fig. 3 Diagram of drug being transferred from a nanodroplet to a cell under the action of ultrasound. | |
In another experiment, Du et al.83 established NBs using PLGA-mPEG, that is, poly(D,L-lactide-co-glycolide)-methoxy-poly(ethylene glycol). The micelles were turned into NBs after being filled with perfluoropentane, sealed by a PEG layer, and processed at 37 °C in NBs. In a mouse tumor model, DOX-loaded NBs demonstrated improved anticancer activity and decreased toxicity. Deng et al.84 prepared NBs for the transmission of drugs to cancer cells by condensing DOX hydrochloride. NBs charged with doxorubicin in HeLa cells were internalized in a time-dependent and concentration-dependent manner and demonstrated greater inhibition of cell growth compared to free DOX. PLGA NBs were also applied by Zhang et al.85 as a targeted chemotherapeutic agent delivery device, as an effective ultrasound contrast agent, and as a synergist for choriocarcinoma therapy by HIFU. Methotrexate-packed NBs (MTX) were successfully equipped and loaded by perfluorocarbon, instead of utilizing the dual emulsion evaporation technique. Monoclonal anti-HLAG antibodies (mAbHLA-G) produced by active tumor cells were conjugated to the NB surface. The mAbHLA-G/MTX/PLGA-loaded NBs demonstrated the controlled death of cancer cells, and correspondingly enhanced ultrasound imaging of the tumor.85 Multifunctional NBs dependent on perfluoropentane stabilized by amphiphilic anti-copolymer (polyethylene glycol anti-polycaprolactone) were created to transmit curcumin upon US irradiation.86 The primed curcumin was firmly held in vivo by NBs and under controlled release, the medication directly attacked the tumor. A new drug targeting method was developed by Lin et al.,87 which focuses on the complex formation of ultrasonic-sensitive nanobubbles and cell-permeable peptides (CPPs). The CPP–DOX compound (CPP–DOX) was enveloped in a NB-adjusted asparagine–glycine–arginine (NGR) peptide, which integrates the special targeting activity of NGR, capacity of CPP to penetrate cells, and NB properties to unlock ultrasonic drugs. Local ultrasonic activation may trigger CPP–DOX to be released from NBs and allow its penetration. This approach shows significant inhibitory effects on tumors, and US stimulation improves the inhibitory efficacy. Xie et al.40 also utilized this technique, where NB structures with the cell penetration property of CPP were successfully applied for targeting camptothecin (CPT). NBs carrying CPPs in the form of a CPP–CPT complex ensured the transmission of CPT into the tumor tissues and improved its delivery capacity. The CPP–CPT NBs showed good cell uptake and significant antitumor activity. Consequently, CPP–CPT NBs with US following systemic administration in mice gave a significant cancer eradication impact in the HeLa cell line and body protection compared to the usual CPT administered group.40
NBs can be used in complexes with liposomes guided by US for drug delivery (Fig. 4).88 NBs can also be utilized in US-targeted liposome complexes for drug distribution. Complexes of nanobubble-paclitaxel liposomes (NB-PTXLp) have been effectively developed and promoted the distribution of medication in tumor cells activated by US irradiation. Liposome linking to the surface of NBs does not affect the imaging capability of the theranostic device NB-PTXLp. Moreover, ultrasound irradiation leads to the cavitation of NBs, resulting in tumor cell sonoporation, which increases the permeability of the cells. This allowed cellular absorption of PTX-loaded liposomes to be promoted and led to the maximum antitumor potency of NB-PTXLps relative to the generic Abraxane and Paclitax formulations.88
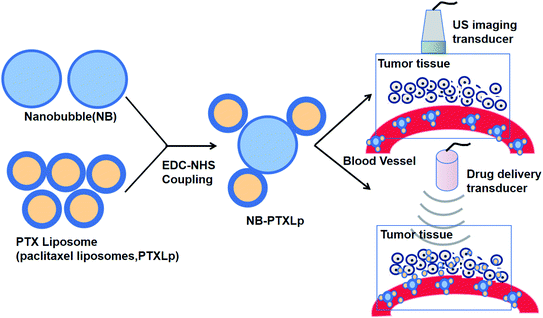 |
| Fig. 4 Complexes of nanobubble-paclitaxel liposomes (NB-PTXLp) were effectively developed and promoted the medication distribution in tumor cells activated by US irradiation. | |
Similarly, another study reported that the injection of liposomal compounds and NBs with low-frequency US may be an effective tumor treatment. They revealed that these conditions activate the anti-tumor immune response needed to reverse tumor development. This study concluded that liposomal nanobubbles combined with ultrasound irradiation can regulate and control the anti-tumor cellular immunologic function, and thus may be an important means for tumor therapy.89
Gao et al. established lipid NBs as an effective delivery mechanism for imaging-guided, cancer-targeted chemotherapy caused by ultrasound. They developed a drug delivery device enabled by US for selective therapeutic anti-tumor applications. The FA-ALNBs collected had strong drug EE with great physiological stability and standardized spheroidal structure. The FA-ALNBs demonstrated ultrasound-controllable capacity for drug release and illustrated substantial in vitro and in vivo anticancer efficacy under US irradiation. Also, the FA-ALNBs did not exhibit any significant toxic effect.90
PFB-NBs were co-loaded onto liposomes of less than 300 nm in diameter with a model compound. The subsequent nested-NBs demonstrated strong ultrasonic echo at conspicuous ultrasonic imaging frequencies between 3 MHz and 8 MHz. Nested-NBs were developed as a compound of both packaged NBs and droplets PFB. The temperature of the sample was also observed to increase more than the expected threshold, and thus the PFB droplets underwent a phase transition from liquid to gas (Fig. 5).91 This led to a three-fold increase in their diameter and promoted the breakdown of the liposome and release of the drugs.91
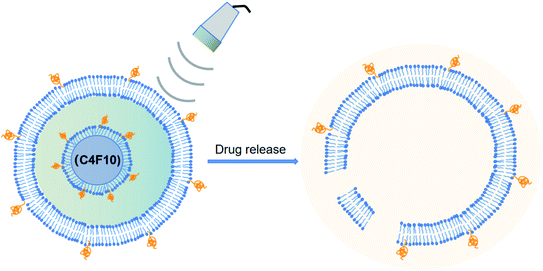 |
| Fig. 5 NBs contain a mixed population of both gaseous and liquid core particles, which upon continuous wave high-intensity focused ultrasound (CW HIFU) undergo rapid phase conversion, triggering liposomal drug release. | |
Herceptin-conjugated, PTX-loaded, PEGylated PLGA-based magnetic NBs were prepared by Song et al. using updated carbodiimide technology and an evaporation procedure of double emulsification. The PTX–USPIO–HER-loaded NBs exhibited a prominent size distribution, superparamagnetism, capacity selection, and USPIO/PTX setting. The quick, guided release of PTX in vitro with US irradiation from the NBs signaled the great potential of this material as a managed release mechanism for anticancer drugs. In particular, PTX–USPIO–HER-NBs could selectively bind with the HER2 antigen existing in SK-BR-3 cells and exhibited greater cytotoxic effects on SK-BR-3 cells than MDA-MB-231 cells, which provides a basis for targeted therapy and imaging. The use of these smart magnetic NBs in breast cancer has the potential for imaging-guided therapy and multimodal imaging.92 The successful DDS can be attributed to the capacity of the FH peptide to attack cancer-associated fibroblasts, and thus the ultrasonic NBs change with FH peptide. Thus, we introduced a novel updated FH peptide and DOX-loaded ultrasonic-sensitive NBs with the potential to attack CAFs by conjugating with tenascin C. These CAF-targeted NBs were called FH-NBs-DOX and not only acted as an ultrasonic contrast agent but also supplied more DOX to CAFs and played a stronger role in ultrasonic irradiation.93
In conclusion, NBs with US may provide some features that are helpful in the distribution of anticancer medication, enhancing the penetration of a chemotherapeutic product into the cancer cell line. This method will provide a positive technique for the site-specific supply of anti-cancer medication, decreasing the sensitivity to non-target tissues to the product, removing systemic side effects, and enhancing its treatment.
4.2 Ultrasound combined with NBs for nucleic acid delivery
4.2.1 Ultrasound combined with NBs for DNA delivery.
Regardless of the sonoporation phenomenon, US interaction with nucleic acid-charged bubbles is suggested to improve the transmission efficiency. This strategy may also contribute to the site-specific release of genetic materials, which reduce non-resonated tissue transfection. Several techniques have been employed for the transfer of genes through NBs, ranging from the concurrent administration of genes to their connotation inside nanobubble systems. There is a variety of methods including utilization of cationic lipids to compose the shell of nanobubbles for electrostatic attachment of DNA, direct physical assembly of DNA in the shells during preparation, application of cationic polymer layers on the shell for electrostatic interaction of DNA, covalent binding of DNA-loaded NBs vectors and the utilization of compatible DNA strands to establish NBs.94 The analysis found that in vitro, lipid-based NBs caused several transfections of the gene than albumin-based NBs. Additionally, major gene transfer with lipid-based NBs was also observed in the liver of mice. Submicron-sized bubbles in conjunction with traditional hand-held ultrasonic testing instruments have proven to be an efficient gene transfer reagent.95 Submicron-scale bubbles were developed and suggested as a prospective gene transmission method. Suzuki et al.96 accomplished the synthesis of novel bubbles (varying from 140 to 190 nm in size) combined with US to improve the efficiency of cancer-specific gene delivery. These researchers effectively revealed, following injection into mice, the gene delivery was restricted into the region accessible by US in a controlled manner, revealing that the device could be applied to increase the transfection of DNA. The report by Takahashi et al.97 displayed that the use of nanosized PEG-modified liposomes filled with gas is an efficient method for the dissemination of pDNA. Using cationic lipids, pDNA-loaded liposome NBs have been equipped to improve liposomal membrane transfection efficiency, stability, and image impact in the USA. Horie et al.98 verified the efficacy of NBs together with US for the transfer of TNF-α genes into tumor cells. TNF-α encoding plasmid DNA, loaded with the lipid-containing NBs, can be spatially and momentarily transfected to tumor cells under ultrasound irradiation. This increases the local production of TNF-α, which induces anticancer properties, increases p53-related apoptosis, decreases the tumor vessel volume, reduces the tumor size, and also minimizes the toxic effects. Watanabe et al.99 measured the potency of gene expression by mixing NBs with US. Their reported stated that the US-NBs system was used to transfect or cotransfect two forms of genes (luciferase and hNIS) into the muscles of the skeletal system. The gene expression kinetics was effectively discovered in vivo with the use of PET (positron emission tomography) and bioluminescence imaging.99
Bisazza et al.41 shaped an odd type of perfluoropentane-core NBs, consisting of a diethylaminoethyl-dextran shell encapsulated with complex DNA, where the diethylaminoethyl-dextran NBs were capable of defending their cargo from transfecting plasmid DNA and protease action. Another polymer NB type composed of a perfluoropentane core for DNA and chitosan shell was further developed by the same group.21 The composition was strengthened by introducing tetradecylphosphoric acid to achieve smaller structures. This can find an amphiphilic molecule in the perfluoropentane–water interface, hence increasing the surface tension.
Considering that chitosan is a biocompatible and low-toxic polycationic polymer, it was selected as the ideal NB shell. These NBs can complex and shield DNA. The DNA-laden NBs demonstrated promise for transfecting COS7 cells without affecting the cell conditions. US triggered their in vitro potential to transfect DNA. In the study by Tayier et al.,100 surface modification with polyethylenimine (PEI) polymer dramatically enhanced the amount of NBs to load DNA, resulting in substantially enhanced transfection performance of genes when paired with ultrasound (Fig. 6).
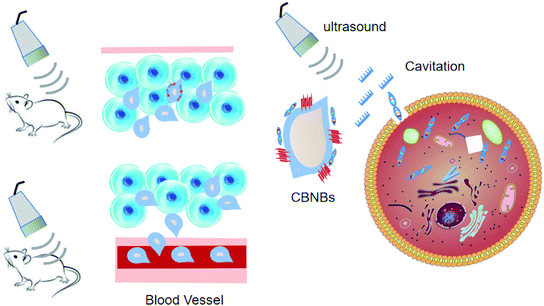 |
| Fig. 6 Ultrasound-assisted gene delivery into tumor tissue. Gene transfection efficiency was improved significantly by cationic biosynthetic nanobubbles (CBNBs) combined with ultrasound. | |
4.2.2 Ultrasound combined with NBs for RNA delivery.
Yin T. et al.101 prepared NBs carrying siRNA for cancer using a hetero-assembly approach to target the SIRT2 gene for anti-apoptosis with ultrasound irradiation. The formulation improved the silencing impact of siRNA-NBs on the genome, resulting in a significant improvement in apoptosis of cancer cells. Consequently, a substantially enhanced therapeutic outcome was observed in a glioma variant of the nude rodent. A further study by Yin T. et al.102 established US-sensitive NBs, which concurrently carried the anti-cancer siRNA and paclitaxel (PTX), aiming at the BCL-2 gene for the therapy of hepatic tumors, based on their promising findings. The delivery of siRNA and PTX was effectively achieved through the injection of the NBs into the blood circulation of nude mice with human HepG2 xenografts applying active low-frequency (below 1 MHz) ultrasound exposure directed to the position of the tumor cells. Tumor development was suppressed utilizing low-dose PTX in animal experiments, owing to the combined antitumor activity of both agents. To treat prostate cancer, Wang et al.103 designed NBs bearing the androgen receptor through the electrostatic approach. Combined with ultrasonic irradiation, siRNA-loaded NBs greatly restrained cell growth and brought about the repression of protein and AR mRNA production. Based on ultrasonic NBs and CPPs, novel siRNA-loaded NBs were created and targeted to treat EphA2-positive cancer. Local activation of US irradiation resulted in the release of CPP-siRNA from the NBs and allowed their penetration into the tumor cells. This played a therapeutic role in cancer after the systemic administration of CPP-siRNA NBs in mice, decreasing c-Myc silencing and impeding cancer proliferation.104–107 Wu et al.108 tested novel NB targeting (TNB) combined with small interfering RNA (siRNA)–cyanine 5 (Cy5) and confirmed its in vivo theranostic ability. NET-1 siRNA-conjugated TNB control in mice was reported to result in a substantial decrease in tumor growth and the maximum survival rate. Li et al.109 studied a non-viral shRNA gene delivery method, which was developed using a mixture of PEI, US, and NBs to target survivors of liver cancer. PEI-shRNA-NBs can act as a useful method for shRNA transmission. The US-sensitive siRNA NBs can be a perfect transmission plasmid for the treatment of tumors to mediate extremely efficient RNA interference.
5. Potential safety concerns
Safety is among the key concepts for designing drug/gene delivery systems. The safety concerns of NBs with ultrasound to achieve enhanced permeability and retention, such as the negative effect of ultrasonic irradiation and NB toxicity, are require to be further thoroughly explored as a concern for their potential clinical applications.
The advantage of NBs over MBs lies in the properties of targeted drug and gene delivery, while NBs need high ultrasonic energy to effectively induce cavitation to allow drug/gene release in target tissues or cells.7,110 This is conducive to the sonoporation of biological membranes for drug delivery. The bubble implosions can be used to induce cell permeability to therapeutic agents by penetrating the cell membrane, but they can also damage biological tissues. Tissue damage induced by ultrasound-mediated transfection is a concern for the safe therapeutic application of this transfection system. Also, high-intensity ultrasound can also cause the rapid collapse of bubbles and rapid release of drugs/genes loaded into the foam, which may not be able to meet the need for the continuous release of some drugs.
Ultrasound irradiation can induce ultrasonic heating and damage biological tissues, and if the pores on the cell membrane produced by ultrasonic cavitation are too large, this will affect the resealing of the pores, and therefore, the ultrasonic parameters are still an obvious problem. Conversely, different intensities of ultrasound therapy can be used for different purposes. Thus, further research is needed to investigate the intensity of ultrasound for different therapeutic purposes.111
Besides these safety concerns, the toxicity of NBs needs to be considered and will be the subject of future research. Zhang et al.112 found that nanobubbles are secure using the CCK-8 assay and histopathology analysis after the injection of nanobubbles. The phospholipids that made up nanobubbles are known to be low-toxicity materials and C3 F8 is also nontoxic; therefore, nanobubbles are safe to use in vitro and in vivo. Some other recent studies have also shown that NBs have no systemic toxicity in vivo, which is ideal for their future clinical application in anticancer therapy.113,114
Although the comprehensive safety assessment remains inadequate, this will be the subject of future research.115 Furthermore, if the use of the nanobubbles is beneficial for treatment, then the effort to translate them into clinical applications will be worth it.
6. Perspectives
Although NBs combined with US for tumor therapy is still in its infancy, they have tremendous appeal. Their unique structure, multifunctional characteristics, high degree of curative effect and the widespread variety of diseases give NBs broad development prospect. It should be noted that some challenges and vital issues will need to be discussed and dealt with when they are involved in biomedical applications and future clinical transformations. The most significant issues, from our point-of-view, are summarized below.
6.1 Ensuring sufficient amount of drug/gene is delivered and released in tumor tissues
The prerequisite for treating diseases is the delivery and release of a sufficient amount of drugs/genes in diseased tissues. Most ultrasonic-response materials require an ultrasonic response core (gaseous, PFC, or gas-generation). These sonogenic response cores take up a large amount of space in sonogenic-response materials (microbubbles, nanobubbles, and droplets), thereby reducing the drug/gene content and the number of tissues to which the drugs/genes are delivered to treat the disease, ultimately leading to limited therapeutic effects.116 Although the opening of the biological membrane using nanobubbles at different concentrations has been demonstrated, the amount of agent transported through the biological membrane at different bubble concentrations has not been quantified. Secondly, besides bubble concentration, there are several additional parameters (e.g., treatment duration, times, and frequency) that should be investigated to further optimize the opening of biological membranes with nanobubbles.
6.2 Targeting of nanobubbles
Nanobubbles have attracted extensive research attention due to their importance in ultrasonic molecular imaging and potential therapy function, such as passive targeting mechanisms known as the enhanced permeability and retention effects (EPR).117 The small size of nanobubbles overcomes the limitations of microbubbles and can penetrate the vascular wall into tumor tissues, which can be used in tumor-targeted imaging and therapy because the vascular endothelial gap of the tumor is about 380–780 nm, much wider than that of normal tissue (less than 7 nm).118
In addition to the role of EPR, the targeting of active tumor cells is also a prospect of enhanced chemotherapy drug therapy using ultrasound nanobubbles. Targeted nanobubbles have attracted extensive interest in the field of targeted tumor therapy and controlled drug delivery because they can carry and deliver drugs under ultrasound monitoring. The surface of nanobubbles can be modified to achieve targeting bubbles,119 where the most common strategy is to attach disease-specific ligands, such as antibodies, aptamers, and peptides.120
6.3 Increasing the stability of NBs
The stability of NBs is still a controversial topic. Nanoscale bubbles are considered to be unstable because of the extremely high inner pressure at the solid–water interface. According to the Epstein–Plesset theory,19 the lifetime of bubbles smaller than 1000 nm should be less than 0.02 s in water. However, significant research has confirmed that NBs can exist for hours or even days.121
Bubbles undergo expansion and suffer unstable and uncontrolled growth at a specific pressure or temperature threshold (pc and Tc, respectively). Borkent et al. reported that cavitation does not happen when the pressure is as low as −6 MPa.122 However, Zhang et al. observed that when the temperature increases up to a value close to the boiling point of water, the NBs also remain stable.123
Thus, due to the importance of the stability of NBs, further experimental data is needed to gain a comprehensive understanding of the stability of NBs.
6.4 Improvement of ultrasonic probes
At present, the instruments and probes for ultrasound imaging and ultrasound therapy are independent, and thus researchers have tried to integrate the probes for ultrasound imaging and ultrasound therapy, but the results have not been very satisfactory. Thus, the development of ultrasonic composite probes that can be used for both ultrasonic imaging and ultrasonic irradiation will provide a great convenience for ultrasound combined with NBs to mediate tumor-targeted therapy.
6.5 Optimization of ultrasonic parameters
Acoustic cavitation is the formation and collapse of vapor bubbles in a liquid due to an acoustic pressure field. Cavitation is generally classified as two types, stable cavitation and inertial cavitation. Inertial cavitation can generate broadband noise emissions when bubbles undergo large radial oscillations and collapse violently, which can damage tissue.124 The use of ultrasound to fragment drug-loaded echogenic liposomes (ELIP) near the target tissue has the potential to produce a large temporal peak in drug or therapeutic effect rather than relying on more gradual passive release, and thus optimization of the ultrasonic parameters is important.
Vascular leakage and apoptosis occur in vascular cells exposed to 1 MHz to 1.5 MHz pulsed ultrasound at peak-to-peak pressure amplitudes between 2 MPa and 36 MPa,125 but Kathryn et al. tested the hypothesis that low-intensity continuous wave (CW) ultrasound (0.49 MPa peak-to-peak pressure amplitude) enhances the delivery of liposome nanobubbles in an ex vivo mouse aorta. Their research showed that 1 MHz CW ultrasound increased the transport of liposome nanobubbles across the endothelium by nucleating stable cavitation.126 Thus, more studies are needed to explore the range of ultrasonic parameters for safety and effectiveness.
7. Conclusions
NBs combined with US is the focus of much work due to their possible application as innovative therapeutic devices in specific clinical environments. They offer a range of advantages appropriate for sophisticated and targeted distribution strategies for anticancer agents. This in turn enhances the therapeutic efficacy of anticancer drugs while minimizing their off-target side effects. Besides, US is a very well-established, non-invasive, and cheap diagnostic imaging procedure, and thus the application of US-mediated drug/gene delivery possesses tremendous potential as a method for future tumor treatment. US combined with NBs may improve the effectiveness of therapeutic agents and promote their distribution on-demand in the appropriate tissues. Therefore, it is the most promising targeted therapy method at present and is expected to be widely used in cancer therapy. Although there are many problems in the current research of nano-drug delivery systems, with the continuous exploration by domestic and foreign researchers, nano-drug delivery systems will have a broader application prospect.
Conflicts of interest
The authors declare that there are no conflicts of interest.
Acknowledgements
This work is financially supported by the National Natural Science Foundation of China (81873898 and 81960316) and the Gansu Province Key Talent Program (201939).
References
- J. Zugazagoitia,
et al., Current challenges in cancer treatment, Clin. Ther., 2016, 38(7), 1551–1566 CrossRef PubMed
.
- R. L. Siegel, K. D. Miller and A. Jemal, Cancer statistics, Ca-Cancer J. Clin., 2017, 67(1), 7–30 CrossRef PubMed
.
- M. J. Thun,
et al., The global burden of cancer: priorities for prevention, Carcinogenesis, 2009, 31(1), 100–110 CrossRef PubMed
.
- D. Peer,
et al., Nanocarriers as an emerging platform for cancer therapy, Nat. Nanotechnol., 2007, 2(12), 751–760 CrossRef CAS PubMed
.
-
X.-J. Liang, et al., Circumventing tumor resistance to chemotherapy by nanotechnology, Multi-Drug Resistance in Cancer, 2010, pp. 467–488 Search PubMed
.
- H. Ünal, N. Öztürk and E. Bilensoy, Formulation development, stability and anticancer efficacy of core-shell cyclodextrin nanocapsules for oral chemotherapy with camptothecin, Beilstein J. Org. Chem., 2015, 11, 204 CrossRef PubMed
.
- A. L. Klibanov,
et al., Ultrasound-triggered release of materials entrapped in microbubble-liposome constructs: a tool for targeted drug delivery, J. Controlled Release, 2010, 148(1), 13–17 CrossRef CAS PubMed
.
-
E. D. Michailidi, et al., Chapter 4 - Fundamentals and applications of nanobubbles, in Interface Science and Technology, ed. G. Z. Kyzas and A. C. Mitropoulos, 2019, Elsevier, pp. 69–99 Search PubMed
.
- F. Bosca,
et al., Porphyrin-Loaded Pluronic Nanobubbles: A New US-Activated Agent for Future Theranostic Applications, Bioconjugate Chem., 2018, 29(2), 234–240 CrossRef CAS PubMed
.
- H. Wu,
et al., Time-intensity-curve Analysis and Tumor Extravasation of Nanobubble Ultrasound Contrast Agents, Ultrasound Med. Biol., 2019, 45(9), 2502–2514 CrossRef PubMed
.
- P. Nittayacharn,
et al., Enhancing Tumor Drug Distribution With Ultrasound-Triggered Nanobubbles, J. Pharm. Sci., 2019, 108(9), 3091–3098 CrossRef CAS PubMed
.
- S. A. Peyman,
et al., On-chip preparation of nanoscale contrast agents towards high-resolution ultrasound imaging, Lab Chip, 2016, 16(4), 679–687 RSC
.
- M. Wu,
et al., Ultrasound-mediated nanobubble destruction (UMND) facilitates the delivery of A10-3.2 aptamer targeted and siRNA-loaded cationic nanobubbles for therapy of prostate cancer, Drug Delivery, 2018, 25(1), 226–240 CrossRef CAS PubMed
.
- Z. Xing,
et al., The fabrication of novel nanobubble ultrasound contrast agent for potential tumor imaging, Nanotechnology, 2010, 21(14), 145607 CrossRef PubMed
.
- R. S. S. Azevedo,
et al., In situ immune response and mechanisms of cell damage in central nervous system of fatal cases microcephaly by Zika virus, Sci. Rep., 2018, 8(1), 1 CrossRef CAS PubMed
.
- R. H. Perera,
et al., Improving performance of nanoscale ultrasound contrast agents using N,N-diethylacrylamide stabilization, Nanomedicine, 2017, 13(1), 59–67 CrossRef CAS PubMed
.
- C. Hernandez,
et al., Sink or float? Characterization of shell-stabilized bulk nanobubbles using a resonant mass measurement technique, Nanoscale, 2019, 11(3), 851–855 RSC
.
- B. H. Tan, H. An and C. D. Ohl, How Bulk Nanobubbles Might Survive, Phys. Rev. Lett., 2020, 124(13), 134503 CrossRef CAS PubMed
.
- M. Alheshibri,
et al., A History of Nanobubbles, Langmuir, 2016, 32(43), 11086–11100 CrossRef CAS PubMed
.
- C. Pellow,
et al., Threshold-dependent nonlinear scattering from porphyrin nanobubbles for vascular and extravascular applications, Phys. Med. Biol., 2018, 63(21), 215001 CrossRef PubMed
.
- R. Cavalli,
et al., New chitosan nanobubbles for ultrasound-mediated gene delivery: preparation and in vitro characterization, Int. J. Nanomed., 2012, 7, 3309–3318 CrossRef CAS PubMed
.
- E. E. J. Marxer,
et al., Development and characterization of new nanoscaled ultrasound active lipid dispersions as contrast agents, Eur. J. Pharm. Biopharm., 2011, 77(3), 430–437 CrossRef CAS PubMed
.
- B. E. O'Neill and N. Rapoport, Phase-shift, stimuli-responsive drug carriers for targeted delivery, Ther. Deliv., 2011, 2(9), 1165–1187 CrossRef PubMed
.
- R. Cavalli, A. Bisazza and D. Lembo, Micro- and nanobubbles: a versatile non-viral platform for gene delivery, Int. J. Pharm., 2013, 456(2), 437–445 CrossRef CAS PubMed
.
- S. Fokong,
et al., Image-guided, targeted and triggered drug delivery to tumors using polymer-based microbubbles, J. Controlled Release, 2012, 163(1), 75–81 CrossRef CAS PubMed
.
- C.-B. Zhang,
et al., Enhancement Effect of Ultrasound-Induced Microbubble Cavitation on Branched Polyethylenimine-Mediated VEGF165 Transfection With Varied N/P Ratio, Ultrasound Med. Biol., 2013, 39(1), 161–171 CrossRef PubMed
.
- X. Cai,
et al., Ultrasound-Responsive Materials for Drug/Gene Delivery, Front. Pharmacol., 2020, 10, 1650 CrossRef PubMed
.
- C. Kim,
et al., Multifunctional microbubbles and nanobubbles for photoacoustic and ultrasound imaging, J. Biomed. Opt., 2010, 15(1), 010510 CrossRef PubMed
.
- H. Li,
et al., Characteristics of micro-nano bubbles and potential application in groundwater bioremediation, Water Environ. Res., 2014, 86(9), 844–851 CrossRef CAS PubMed
.
- M. S. Khan,
et al., Oxygen-Carrying Micro/Nanobubbles: Composition, Synthesis Techniques and Potential Prospects in Photo-Triggered Theranostics, Molecules, 2018, 23(9), 2210 CrossRef PubMed
.
- L. C. du Toit,
et al., Investigating the effect of polymeric approaches on circulation time and physical properties of nanobubbles, Pharm. Res., 2011, 28(3), 494–504 CrossRef CAS PubMed
.
- A. Delalande,
et al., Ultrasound and microbubble-assisted gene delivery: recent advances and ongoing challenges, Ther. Deliv., 2012, 3(10), 1199–1215 CrossRef CAS PubMed
.
- S. V. V. N. Kothapalli,
et al., Assessment of the Viscoelastic and Oscillation Properties of a Nano-engineered Multimodality Contrast Agent, Ultrasound Med. Biol., 2014, 40(10), 2476–2487 CrossRef PubMed
.
- K. Hettiarachchi,
et al., On-chip generation of microbubbles as a practical technology for manufacturing contrast agents for ultrasonic imaging, Lab Chip, 2007, 7(4), 463–468 RSC
.
- G. Korpanty,
et al., Targeting vascular endothelium with avidin microbubbles, Ultrasound Med. Biol., 2005, 31(9), 1279–1283 CrossRef PubMed
.
- N. Matsuki,
et al., Oxygen supersaturated fluid using fine micro/nanobubbles, Int. J. Nanomed., 2014, 9, 4495 CrossRef PubMed
.
- E. J. Swanson,
et al., Injectable oxygen delivery based on protein-shelled microbubbles, Nano LIFE, 2010, 1, 215–218 CrossRef CAS
.
- F. Cavalieri,
et al., Ultrasonic Synthesis of Stable, Functional Lysozyme Microbubbles, Langmuir, 2008, 24(18), 10078–10083 CrossRef CAS PubMed
.
- M. Prato,
et al., 2H,3H-Decafluoropentane-based nanodroplets: new perspectives for oxygen delivery to hypoxic cutaneous tissues, PLoS One, 2015, 10(3), e0119769 CrossRef PubMed
.
- X. Xie,
et al., Ultrasound-responsive nanobubbles contained with peptide–camptothecin conjugates for targeted drug delivery, Drug Delivery, 2016, 23(8), 2756–2764 CrossRef CAS PubMed
.
- A. Bisazza,
et al., The in vitro characterization of dextran-based nanobubbles as possible DNA transfection agents, Soft Matter, 2011, 7(22), 10590–10593 RSC
.
- R. Cavalli,
et al., Preparation and characterization of dextran nanobubbles for oxygen delivery, Int. J. Pharm., 2009, 381, 160–165 CrossRef CAS PubMed
.
- T. M. Krupka,
et al., Formulation and characterization of echogenic lipid-Pluronic nanobubbles, Mol. Pharmaceutics, 2010, 7(1), 49–59 CrossRef CAS PubMed
.
- P. Bhandari, X. Wang and J. Irudayaraj, Oxygen nanobubble tracking by light scattering in single cells and tissues, ACS Nano, 2017, 11, 2682–2688 CrossRef CAS PubMed
.
- N. Rapoport, Z. Gao and A. Kennedy, Multifunctional nanoparticles for combining ultrasonic tumor imaging and targeted chemotherapy, J. Natl. Cancer Inst., 2007, 99(14), 1095–1106 CrossRef CAS PubMed
.
- N. Rapoport,
et al., Ultrasound-mediated tumor imaging and nanotherapy using drug loaded, block copolymer stabilized perfluorocarbon nanoemulsions, J. Controlled Release, 2011, 153(1), 4–15 CrossRef CAS PubMed
.
- M. P. Krafft, V. B. Fainerman and R. Miller, Modeling of the effect of fluorocarbon gases on the properties of phospholipid monolayers and the adsorption dynamics of their aqueous solutions or dispersions, Colloid Polym. Sci., 2015, 293(11), 3091–3097 CrossRef CAS
.
- N. Basilico,
et al., Dextran-shelled oxygen-loaded nanodroplets reestablish a normoxia-like pro-angiogenic phenotype and behavior in hypoxic human dermal microvascular endothelium, Toxicol. Appl. Pharmacol., 2015, 288(3), 330–338 CrossRef CAS PubMed
.
- L. Wang, M. Zhang, K. Tan, Y. Guo and H. Tong,
et al., Preparation of Nanobubbles Carrying Androgen Receptor siRNA and Their Inhibitory Effects on Androgen-Independent Prostate Cancer when Combined with Ultrasonic Irradiation, PLoS One, 2014, 9(5), 1–12 CAS
.
- R. H. Abou-Saleh, S. A. Peyman and B. R. G. Johnson, The influence of intercalating perfluorohexane into lipid shells on nano and microbubble stability, Soft Matter, 2016, 12(34), 7223–7230 RSC
.
- S. Capece,
et al., A general strategy for obtaining biodegradable polymer shelled microbubbles as theranostic devices, Chem. Commun., 2013, 49(51), 5763–5765 RSC
.
- R. Cavalli, M. Soster and M. Argenziano, Nanobubbles: a promising efficient tool for therapeutic delivery, Ther. Deliv., 2016, 7(2), 117–138 CrossRef CAS PubMed
.
- T. Yin,
et al., Nanobubbles for enhanced ultrasound imaging of tumors, Int. J. Nanomed., 2012, 7, 895–904 CAS
.
- J. Zhang,
et al., The Optimized Fabrication of a Novel Nanobubble for Tumor Imaging, Front. Pharmacol., 2019, 10, 610 CrossRef CAS PubMed
.
- E. Stride and M. Edirisinghe, Novel microbubble preparation technologies, Soft Matter, 2008, 4(12), 2350–2359 RSC
.
- Z. Xing,
et al., Novel ultrasound contrast agent based on microbubbles generated from surfactant mixtures of Span 60 and polyoxyethylene 40 stearate, Acta Biomater., 2010, 6(9), 3542–3549 CrossRef CAS PubMed
.
- A. H. Dhanaliwala,
et al., In vivo imaging of microfluidic-produced microbubbles, Biomed. Microdevices, 2015, 17(1), 23 CrossRef PubMed
.
- S. Wang,
et al., Production rate and diameter analysis of spherical monodisperse microbubbles from two-dimensional, expanding-nozzle flow-focusing microfluidic devices, Biomicrofluidics, 2013, 7(1), 14103 CrossRef PubMed
.
- P. S. Sheeran,
et al., Formulation and acoustic studies of a new phase-shift agent for diagnostic and therapeutic ultrasound, Langmuir, 2011, 27(17), 10412–10420 CrossRef CAS PubMed
.
- N. Y. Rapoport,
et al., Controlled and targeted tumor chemotherapy by ultrasound-activated nanoemulsions/microbubbles, J. Controlled Release, 2009, 138(3), 268–276 CrossRef CAS PubMed
.
- R. Cavalli, M. Soster and M. Argenziano, Nanobubbles: a promising efficient tool for therapeutic delivery, Ther. Delivery, 2016, 7(2), 117–138 CrossRef CAS PubMed
.
- J. Zhang, Y. Chen and C. Deng, The Optimized Fabrication of a Novel Nanobubble for Tumor Imaging, Front. Pharmacol., 2019, 10(610), 1–15 CrossRef PubMed
.
- B. E. Oeffinger and M. A. Wheatley, Development and characterization of a nano-scale contrast agent, Ultrasonics, 2004, 42(1–9), 343–347 CrossRef CAS PubMed
.
- V. Torchilin, Tumor delivery of macromolecular drugs based on the EPR effect, Adv. Drug Deliv. Rev., 2011, 63(3), 131–135 CrossRef CAS PubMed
.
- H. Maeda, H. Nakamura and J. Fang, The EPR effect for macromolecular drug delivery to solid tumors: Improvement of tumor uptake, lowering of systemic toxicity, and distinct tumor imaging in vivo, Adv. Drug Deliv. Rev., 2013, 65(1), 71–79 CrossRef CAS PubMed
.
- V. P. Chauhan,
et al., Normalization of tumour blood vessels improves the delivery of nanomedicines in a size-dependent manner, Nat. Nanotechnol., 2012, 7(6), 383–388 CrossRef CAS PubMed
.
- L. Duan,
et al., Micro/nano-bubble-assisted ultrasound to enhance the EPR effect and potential theranostic applications, Theranostics, 2020, 10(2), 462–483 CrossRef CAS PubMed
.
- P. Bhandari,
et al., Ultrasound beam steering of oxygen nanobubbles for enhanced bladder cancer therapy, Sci. Rep., 2018, 8(1), 3112 CrossRef PubMed
.
- H. A. Hancock, L. H. Smith and J. Cuesta,
et al., Investigations into pulsed high-intensity focused ultrasound-enhanced delivery: preliminary evidence for a novel mechanism, Ultrasound Med. Biol., 2009, 35(10), 1722–1736 CrossRef PubMed
.
- R. Williams, Production and transmission of ultrasound, Physiotherapy, 1987, 73(3), 113–116 Search PubMed
.
- W. L. Nyborg, Biological effects of ultrasound: development of safety guidelines. Part II: general review, Ultrasound Med. Biol., 2001, 27(3), 301–333 CrossRef CAS PubMed
.
- S. A. A. Rizvi and A. M. Saleh, Applications of nanoparticle systems in drug delivery technology, Saudi Pharmaceut. J., 2018, 26(1), 64–70 CrossRef PubMed
.
- A. Cruz,
et al., Influence of a Fluorescent Probe on the Nanostructure of Phospholipid Membranes: Dipalmitoylphosphatidylcholine Interfacial Monolayers, Langmuir, 2005, 21(12), 5349–5355 CrossRef CAS PubMed
.
- H. Yang,
et al., Nanobubble-Affibody: Novel ultrasound contrast agents for targeted molecular ultrasound imaging of tumor, Biomaterials, 2015, 37, 279–288 CrossRef CAS PubMed
.
- M. Eeman,
et al., Penetration of Surfactin into Phospholipid Monolayers: Nanoscale Interfacial Organization, Langmuir, 2006, 22(26), 11337–11345 CrossRef CAS PubMed
.
- X. Fan,
et al., Ultrasonic Nanobubbles Carrying Anti-PSMA Nanobody: Construction and Application in Prostate Cancer-Targeted Imaging, PLoS One, 2015, 10(6), e0127419 CrossRef PubMed
.
- M. Suzuki,
et al., Nanobubbles enhanced drug susceptibility of cancer cells using ultrasound, Int. Congr. Ser., 2005, 1284, 338–339 CrossRef CAS
.
- P. Bhandari,
et al., Ultrasound beam steering of oxygen nanobubbles for enhanced bladder cancer therapy, Sci. Rep., 2018, 8(1), 3112 CrossRef PubMed
.
- Y. Tian,
et al., Apatinib-loaded lipid nanobubbles combined with ultrasound-targeted nanobubble destruction for synergistic treatment of HepG2 cells in vitro, OncoTargets Ther., 2018, 11, 4785–4795 CrossRef PubMed
.
- X. Zhou,
et al., Biocompatible Chitosan Nanobubbles for Ultrasound-Mediated Targeted Delivery of Doxorubicin, Nanoscale Res. Lett., 2019, 14(1), 24 CrossRef PubMed
.
- Z. Gao,
et al., Drug-loaded nano/microbubbles for combining ultrasonography and targeted chemotherapy, Ultrasonics, 2008, 48(4), 260–270 CrossRef CAS PubMed
.
- N. Y. Rapoport,
et al., Controlled and targeted tumor chemotherapy by ultrasound-activated nanoemulsions/microbubbles, J. Controlled Release, 2009, 138(3), 268–276 CrossRef CAS PubMed
.
- L. Du,
et al., Ultrasound-Triggered Drug Release and Enhanced Anticancer Effect of Doxorubicin-Loaded Poly(D,L-Lactide-Co-Glycolide)-Methoxy-Poly(Ethylene Glycol) Nanodroplets, Ultrasound Med. Biol., 2011, 37(8), 1252–1258 CrossRef PubMed
.
- L. Deng,
et al., Development and optimization of doxorubicin loaded poly(lactic-co-glycolic acid) nanobubbles for drug delivery into HeLa cells, J. Nanosci. Nanotechnol., 2014, 14(4), 2947–2954 CrossRef CAS PubMed
.
- X. Zhang,
et al., Methotrexate-loaded PLGA nanobubbles for ultrasound imaging and Synergistic Targeted therapy of residual tumor during HIFU ablation, Biomaterials, 2014, 35(19), 5148–5161 CrossRef CAS PubMed
.
- G. Ji, J. Yang and J. Chen, Preparation of novel curcumin-loaded multifunctional nanodroplets for combining ultrasonic development and targeted chemotherapy, Int. J. Pharm., 2014, 466(1–2), 314–320 CrossRef CAS PubMed
.
- W. Lin,
et al., Cell-penetrating peptide-doxorubicin conjugate loaded NGR-modified nanobubbles for ultrasound triggered drug delivery, J. Drug Target., 2016, 24(2), 134–146 CrossRef CAS PubMed
.
- A. Prabhakar and R. Banerjee, Nanobubble Liposome Complexes for Diagnostic Imaging and Ultrasound-Triggered Drug Delivery in Cancers: A Theranostic Approach, ACS Omega, 2019, 4(13), 15567–15580 CrossRef CAS PubMed
.
- R. Suzuki,
et al., Tumor growth suppression by the combination of nanobubbles and ultrasound, Canc. Sci., 2016, 107(3), 217–223 CrossRef CAS PubMed
.
- S. Gao, X. Cheng and J. Li, Lipid nanobubbles as an ultrasound-triggered artesunate delivery system for imaging-guided, tumor-targeted chemotherapy, OncoTargets Ther., 2019, 12, 1841–1850 CrossRef CAS PubMed
.
- D. V. B. Batchelor,
et al., Nested Nanobubbles for Ultrasound-Triggered Drug Release, ACS Appl. Mater. Interfaces, 2020, 12(26), 29085–29093 CAS
.
- W. Song,
et al., Magnetic nanobubbles with potential for targeted drug delivery and trimodal imaging in breast cancer: an in vitro study, Nanomedicine, 2017, 12(9), 991–1009 CrossRef CAS PubMed
.
- L. Guo,
et al., New FH peptide-modified ultrasonic nanobubbles for delivery of doxorubicin to cancer-associated fibroblasts, Nanomedicine, 2019, 14(22), 2957–2971 CrossRef CAS PubMed
.
- R. Cavalli, A. Bisazza and D. Lembo, Micro- and nanobubbles: A versatile non-viral platform for gene delivery, Int. J. Pharm., 2013, 456(2), 437–445 CrossRef CAS PubMed
.
- H. Kida, Nanobubble Mediated Gene Delivery in Conjunction With a Hand-Held Ultrasound Scanner, Front. Pharmacol., 2020, 11(363), 1–10 Search PubMed
.
- R. Suzuki,
et al., Tumor specific ultrasound enhanced gene transfer in vivo with novel liposomal bubbles, J. Controlled Release, 2008, 125(2), 137–144 CrossRef CAS PubMed
.
- Y. Endo-Takahashi,
et al., pDNA-loaded Bubble liposomes as potential ultrasound imaging and gene delivery agents, Biomaterials, 2013, 34(11), 2807–2813 CrossRef CAS PubMed
.
- S. Horie,
et al., Evaluation of antitumor effects following tumor necrosis factor-α gene delivery using nanobubbles and ultrasound, Cancer Sci., 2011, 102(11), 2082–2089 CrossRef CAS PubMed
.
- Y. Watanabe,
et al., Delivery of Na/I symporter gene into skeletal muscle using nanobubbles and ultrasound: visualization of gene expression by PET, J. Nucl. Med., 2010, 51(6), 951–958 CrossRef CAS PubMed
.
- B. Tayier,
et al., Biosynthetic nanobubbles for targeted gene delivery by focused ultrasound, Nanoscale, 2019, 11(31), 14757–14768 RSC
.
- T. Yin,
et al., Ultrasound-sensitive siRNA-loaded nanobubbles formed by hetero-assembly of polymeric micelles and liposomes and their therapeutic effect in gliomas, Biomaterials, 2013, 34(18), 4532–4543 CrossRef CAS PubMed
.
- T. Yin,
et al., Tumor-penetrating codelivery of siRNA and paclitaxel with ultrasound-responsive nanobubbles hetero-assembled from polymeric micelles and liposomes, Biomaterials, 2014, 35(22), 5932–5943 CrossRef CAS PubMed
.
- L. Wang,
et al., Preparation of nanobubbles carrying androgen receptor siRNA and their inhibitory effects on androgen-independent prostate cancer when combined with ultrasonic irradiation, PLoS One, 2014, 9(5), e96586 CrossRef PubMed
.
- X. Xie,
et al., Cell-penetrating peptide-siRNA conjugate loaded YSA-modified nanobubbles for ultrasound triggered siRNA delivery, Colloids Surf., B, 2015, 136, 641–650 CrossRef CAS PubMed
.
- Z. Zhou,
et al., Synergistic effects of ultrasound-targeted microbubble destruction and TAT peptide on gene transfection: An experimental study in vitro and in vivo, J. Controlled Release, 2013, 170(3), 437–444 CrossRef CAS PubMed
.
- F. Zhu,
et al., Effectiveness of localized ultrasound-targeted microbubble destruction with doxorubicin liposomes in H22 mouse hepatocellular carcinoma model, J. Drug Target., 2015, 23(4), 323–334 CrossRef CAS PubMed
.
- E. Stride, Physical principles of microbubbles for ultrasound imaging and therapy, Cerebrovasc. Dis., 2009, 27(2), 1–13 CrossRef PubMed
.
- B. Wu,
et al., Preparation of novel targeting nanobubbles conjugated with small interfering RNA for concurrent molecular imaging and gene therapy in vivo, FASEB J., 2019, 33(12), 14129–14136 CrossRef CAS PubMed
.
- H. Li,
et al., Enhanced shRNA delivery by the combination of polyethylenimine, ultrasound, and nanobubbles in liver cancer, Technol. Health Care, 2019, 27, 263–272 Search PubMed
.
- S. R. Sirsi and M. A. Borden, State-of-the-art materials for ultrasound-triggered drug delivery, Adv. Drug Delivery Rev., 2014, 72, 3–14 CrossRef CAS PubMed
.
- U. Adhikari, A. Goliaei and M. L. Berkowitz, Nanobubbles, cavitation, shock waves and traumatic brain injury, Phys. Chem. Chem. Phys., 2016, 18(48), 32638–32652 RSC
.
- J. Zhang,
et al., The Optimized Fabrication of a Novel Nanobubble for Tumor Imaging, Front. Pharmacol., 2019, 31(10), 610 CrossRef PubMed
.
- Hu. Cong,
et al., Ultrasound-mediated nanobubble destruction (UMND) facilitates the delivery of VEGFR2-targeted CD-TK-loaded cationic nanobubbles in the treatment of bladder cancer, J. Cancer Res. Clin. Oncol., 2020, 146(6), 1415–1426 CrossRef PubMed
.
- S. Gao,
et al., Lipid nanobubbles as an ultrasound-triggered artesunate delivery system for imaging-guided, tumor-targeted chemotherapy, OncoTargets Ther., 2019, 12, 1841–1850 CrossRef CAS PubMed
.
- B. Cheng,
et al., Influence of Nanobubble Concentration on Blood-Brain Barrier Opening Using Focused Ultrasound Under Real-Time Acoustic Feedback Control, Ultrasound Med. Biol., 2019, 45(8), 2174–2187 CrossRef PubMed
.
- M. L. Fabiilli,
et al., Delivery of chlorambucil using an acoustically-triggered perfluoropentane emulsion, Ultrasound Med. Biol., 2010, 36(8), 1364–1375 CrossRef PubMed
.
- H. Maeda, T. Sawa and T. Konno, Mechanism of tumor-targeted delivery of macromolecular drugs, including the EPR effect in solid tumor and clinical overview of the prototype polymeric drug SMANCS, J. Controlled Release, 2001, 74(1–3), 47–61 CrossRef CAS PubMed
.
- W. B. Cai,
et al., The Optimized Fabrication of Nanobubbles as Ultrasound Contrast Agents for Tumor Imaging, Sci. Rep., 2015, 5, 13725 CrossRef PubMed
.
- B. A. Kaufmann,
et al., Detection of recent myocardial ischaemia by molecular imaging of P-selectin with targeted contrast echocardiography, Eur. Heart J., 2007, 28(16), 2011–2017 CrossRef PubMed
.
- A. L. Klibanov, Ultrasound molecular imaging with targeted microbubble contrast agents, J. Nucl. Cardiol., 2007, 14(6), 876–884 CrossRef PubMed
.
- S. Karpitschka,
et al., Nonintrusive optical visualization of surface nanobubbles, Phys. Rev. Lett., 2012, 109(6), 066102 CrossRef PubMed
.
- B. M. Borkent,
et
al., Superstability of surface nanobubbles, Phys. Rev. Lett., 2007, 98(20), 204502 CrossRef PubMed
.
- X. Zhang,
et al., Surface nanobubbles nucleate microdroplets, Phys. Rev. Lett., 2014, 112(14), 144503 CrossRef PubMed
.
- C. K. Holland,
et al., Direct evidence of cavitation in vivo from diagnostic ultrasound, Ultrasound Med. Biol., 1996, 22(7), 917–925 CrossRef CAS PubMed
.
- J. H. Hwang,
et al., Vascular effects induced by combined 1-MHz ultrasound and microbubble contrast agent treatments in vivo, Ultrasound Med. Biol., 2005, 31(4), 553–564 CrossRef PubMed
.
- K. E. Hitchcock,
et al., Ultrasound-enhanced delivery of targeted echogenic liposomes in a novel ex vivo mouse aorta model, J. Controlled Release, 2010, 144(3), 288–295 CrossRef CAS PubMed
.
|
This journal is © The Royal Society of Chemistry 2021 |