DOI:
10.1039/D1RA01486B
(Paper)
RSC Adv., 2021,
11, 15204-15212
Enzymatic preparation of phytosterol esters with fatty acids from high-oleic sunflower seed oil using response surface methodology†
Received
24th February 2021
, Accepted 5th April 2021
First published on 22nd April 2021
Abstract
Phytosterol esters are functional compounds that can effectively reduce plasma cholesterol concentration, and have wide applications in the food industry. In this study, a simple and efficient enzymatic method was successfully applied to synthesize phytosterol oleic acid esters with fatty acids from high-oleic sunflower seed oil. Among the tested lipases, Candida rugosa lipase (CRL) exhibited higher catalytic activity in the esterification of phytosterols with fatty acids (oleic acid 84%) from high-oleic sunflower seed oil. Box–Behnken design and response surface methodology were used to investigate the influence of reaction factors on the conversion of phytosterols. The maximum conversion of phytosterols (96.8%) and yield of phytosterol esters (92%) could be obtained under optimal conditions: reaction temperature 50 °C, a molar ratio of phytosterols to fatty acids at 1
:
2.3, enzyme loading of 5.8%, isooctane volume of 2 mL and reaction time of 2 h. It was noteworthy that this enzymatic esterification method indeed expended a much shorter reaction time (2 h) than that observed in previous reports. In general, the enzymatic preparation of phytosterol oleic acid esters with fatty acids from high-oleic sunflower seed oil will be a simple and rapid method for producing unsaturated fatty acid esters of phytosterol with both higher oil solubility and oxidative stability, which is beneficial as functional food ingredients.
1. Introduction
Phytosterols have attracted increasing attention due to their excellent cholesterol-lowering, anti-inflammatory, antioxidant and cardiovascular-protective activity.1–5 A study showed that a daily intake of 1.5–3 g phytosterols could cause a decrease of 8%–15% in low-density lipoprotein cholesterol (LDL-C).6 Despite their potential applications, the bioavailability of phytosterols was greatly limited by their high melting points (>135 °C), weak oil solubility and water solubility.7 Esterification of phytosterols with fatty acids is one of the most efficient methods to overcome these limitations.1,8,9 The solubility of phytosterols was greatly improved after esterification, whereas the melting point was lowered. Many reports have identified that the fatty acid esters of phytosterols (so-called phytosterol esters) could effectively decrease LDL-C levels in a similar manner as free phytosterols.10
The esterification of phytosterols and fatty acids can be catalyzed by chemical catalysts or enzymatic catalysts (e.g., lipase).1 Conventional chemical catalysts (acid catalysts), including H2SO4 and p-toluenesulfonic acid, have been studied extensively in the esterification of phytosterols with fatty acids.11–13 However, the acid-catalyzed synthesis of phytosterol esters generally required harsh reaction temperatures (>130 °C), which easily led to many side-reactions (e.g., dehydration of phytosterols, isomerization of unsaturated fatty acids).12,14 Moreover, the traditional acid catalyst-promoted esterification of phytosterols was more suitable for saturated fatty acids (e.g., lauric acid, palmitic acid) as substrates due to their better stability at high heating temperature. However, unsaturated fatty acids, especially polyunsaturated fatty acids (e.g., linoleic acid, linolenic acid), would be oxidized at high reaction temperature and had lower esterification activity.1 In recent years, the enzyme-catalyzed synthesis of phytosterol unsaturated fatty acid esters has attracted increasing attention as these methods have the advantages of mild and environmentally friendly reaction conditions and fewer side-products.1,15–22 Thus, many efforts have been focused on promoting the efficiency of enzymatic catalysis.
Based on the reaction participants, the enzymatic preparation of unsaturated fatty acid esters of phytosterol can be divided into two types: (1) esterification of phytosterols with unsaturated fatty acids;1,15–17 (2) transesterification of phytosterols with fatty acid esters (e.g., fatty acid ethyl esters, edible oils).8,21,22 In most cases, the synthesis of phytosterol fatty acid esters using fatty acids as an acyl donor was more convenient than that using fatty acids esters (e.g., fatty acid glyceride) due to the ease of separation of excess free fatty acids from the reaction mixtures. Many lipases, such as Carica papaya, Ricinus communis, Rhizomucor miehei, Candida antarctica B and Candida rugosa, have been investigated as bio-catalysts for the esterification of phytosterols with unsaturated fatty acids.1 Among these lipases, Candida rugosa lipase (CRL) has been proven to be efficient for various fatty acids, such as lauric acid,17 oleic acid,15 linoleic acid22 and linolenic acid.19 Compared with phytosterol saturated fatty acid esters, phytosterol unsaturated fatty acid esters exhibited much higher solubility in edible oils (32–36 g/100 mL).19,23 Since oleic acid (C18:1) showed more oxidative stability than polyunsaturated fatty acids (e.g., linoleic acid), phytosterol esters from oleic acid were shown to be more stable than other phytosterol polyunsaturated fatty acid esters (e.g., linoleic acid and linolenic acid),24,25 which will broaden the applications of phytosterol esters in the food industry.
High-oleic sunflower seed oil contains a higher content of oleic acid (∼85%) than other commercial vegetable oils, including olive oil (oleic acid ∼ 78%), camellia seed oil (oleic acid ∼ 79%) and high-oleic peanut oil (oleic acid > 75%).26 Meanwhile, as a good oleic acid resource, the price of high-oleic sunflower seed oil is cheaper than the price of olive oil, camellia seed oil and high-oleic peanut oil. Aiming to develop an efficient method for the preparation of phytosterol esters with higher solubility in edible oils and excellent oxidative stability, the enzymatic preparation of phytosterol oleic acid esters with fatty acids from high-oleic sunflower seed oil has been investigated in this work. Considering the longer esterification reaction time (17–72 h) using Candida rugosa lipase (CRL) as a bio-catalyst in previous studies,15–18 Box–Behnken design (BBD) and response surface methodology were used to optimize the reaction factors in the conversion of phytosterols, and the reaction mechanism was discussed.
2. Materials and methods
2.1 Materials and instruments
Phytosterols (purity 95%), composed of 25% stigmasterol, 45% β-sitosterol and 30% campesterol, were purchased from Wuhan Yuancheng Creation Technology Co., Ltd. (Wuhan, China). High-oleic sunflower seed oil was purchased from a local supermarket. Oleic acid (85%) was purchased from Aladdin Reagents Co., Ltd. (Shanghai, China). C. rugosa lipase (CRL) was purchased from Sigma-Aldrich Chemical Co. Ltd. (Shanghai, China). Stearic acid (99%) and palmitic acid (99%) were obtained from Sinopharm Chemical Reagent Co. Ltd. (Shanghai, China). Lipozyme RMIM, TMIM, CALA and Novozym 435 were purchased from Novozym Biotechnology Co., Ltd. (Bagsvaerd, Denmark). N-Hexane (HPLC grade) was purchased from Thermo Fisher Scientific Co. Ltd. (Shanghai, China). Isooctane, cyclohexane, toluene, acetone and other reagents were of analytical grade, and purchased from Kemiou Chemical Reagent Co. Ltd. (Tianjin, China).
2.2 Preparation of fatty acid from high-oleic sunflower seed oil
Sodium hydroxide (NaOH, 20 g) and ethanol (95%, 200 mL) were added to a 1000 mL three-neck flask (reactor), and stirred at 80 °C under the condition of condensation reflux until NaOH was dissolved. Then, high-oleic sunflower seed oil (100 g) was added to the solution and reacted for 4 h. After the reaction, the unreacted oil was washed off by adding petroleum ether, and the soap layer was obtained after centrifugation. Then, a solution of sulfuric acid (3 mol L−1) was added to the soap and stirred for 30 min at a speed of 300 rpm at room temperature to form two phases. The upper phase was reserved, washed and dried to obtain the pure fatty acids.
2.3 Esterification of phytosterols with fatty acid
The esterification of phytosterols with fatty acid was carried out in isooctane with CRL as the catalyst. First, phytosterols (246 mg), fatty acid (253 mg), CRL (40 mg) and a magnetic stirrer were successively added into a 25 mL reaction tube. Isooctane was added to the reaction tube with a syringe. After the resulting mixture was stirred for a certain time at 900 rpm, it was heated to the specified temperature (20–60 °C) under a nitrogen atmosphere. After the reaction, the mixture was diluted with n-hexane in a 25 mL volumetric flask, then 1 mL of the mixture was transferred to a 10 mL volumetric flask and shaken evenly to determine the conversion of phytosterols, which was expressed as follows:15
Conversion of phytosterols = (A − B)/A × 100% |
where A is the molar amount of phytosterols at the beginning of the reaction, and B is the molar amount of phytosterols at the end of reaction.
2.4 Experimental design by response surface methodology
A three-factor-three-level design was carried out based on the Box–Behnken design (BBD) in this study. The three most important factors were examined as follows: reaction temperature (30, 40, and 50 °C), molar ratio of the substrates (1
:
1, 1
:
2, and 1
:
3), and enzyme loading (4%, 6%, and 8%). A total of 17 experimental tests with 5 center points were performed. In order to investigate the relationships and interaction of the variables, Design-Expert® software version 10 was used to analyze the data, and the quadratic polynomial equation was obtained as follows:
where Y is the predicted response variable, Xi and Xj are code-independent variables, and β0, βi, βii, and βij are the intercept, linear, quadratic, and interaction terms, respectively.
2.5 Analysis of fatty acid composition by GC
An Agilent 6890N gas chromatograph (Agilent Technologies Co., Ltd., USA), equipped with a flame ionization detector (FID) and a BPX-70 capillary column (30.0 m × 0.25 mm × 0.25 μm) (Agilent Technologies Co., Ltd., USA) was used. Nitrogen was used as the carrier gas with a flow of 1.0 mL min−1. The oven was programmed from the set temperature of 170 °C to 210 °C at 2 °C min−1. The injection volume and split ratio were 1 μL and 50
:
1, respectively. The GC injection and detector temperature were 250 °C and 300 °C, respectively.
2.6 Analysis of conversion of phytosterols by HPLC
A Waters e2695 high performance liquid chromatography (HPLC) system equipped with a Waters 2489 UV detector was used for the products analysis (Waters Technology Co. Ltd., USA). Chromatographic separation was carried out on a silica gel column (4.6 × 250 mm × 5 μm) (Waters Technology Co. Ltd., USA). The mobile phases were n-hexane and 2-propanol (20
:
1, v/v) with a flow rate of 0.8 mL min−1. The injection volume was 10 μL, and the column temperature and detection wavelength were set at 30 °C and 280 nm, respectively.
2.7 Yield of phytosterol esters
The phytosterol esters crude products obtained by esterification were purified by a mixed solution of n-hexane and anhydrous ethanol (1
:
1.5, v/v) at 50 °C. Then, hot NaHCO3 solution (0.5 mol L−1, 80 °C) was added to the mixture under agitation until it became neutral. Then, the mixture was centrifuged at 10
000 rpm. The supernatant was retained and dried, and the high purity phytosterol esters were obtained. The isolated yield of the phytosterol esters was calculated as follows:
Yield of phytosterol esters = C/D × 100% |
where C is the mass of the phytosterol esters obtained after purification, and D is the mass of the theoretical phytosterol esters under optimum conditions.
2.8 Statistical analysis
All data were the average of at least three independent replicates, and the mean values and standard deviations were calculated. Origin 8.5 was used to process and draw the test data. Analysis of variance (ANOVA) was performed by SPSS 20.0, and the differences were statistically significant by Duncan's test (p < 0.05).
3. Results and discussion
3.1 Fatty acid composition of high-oleic sunflower seed oil
The fatty acid composition of high-oleic sunflower seed oil was determined by GC method, and the results are shown in Table 1. The oleic acid content, unsaturated fatty acid content (oleic acid plus linoleic acid) and saturated fatty acid content (palmitic acid plus stearic acid) of high-oleic acid sunflower oil was 83.6%, 92.2% and 7.8%, respectively. Oleic acid (C18:1) is a monounsaturated fatty acid, which has far better oxidation stability than that of linoleic acid (C18:2), linolenic acid (C18:3) and other polyunsaturated fatty acids.27 In addition, oleic acid has the physiological function of reducing the blood lipid level and preventing cardiovascular disease in patients with hyper lipidemia.28 Thus, high-oleic sunflower seed oil could provide desirable oleic acid for the preparation of phytosterol esters.
Table 1 Fatty acid composition of high-oleic sunflower seed oil
Fatty acid |
Palmitic acid (16:0) |
Stearic acid (18:0) |
Oleic acid (18:1) |
Linoleic acid (18:2) |
Content (%) |
4.4 |
3.4 |
83.6 |
8.6 |
3.2 Catalytic performance of the enzyme
As the bio-catalyst of the reaction, the enzyme plays a very important role in the synthesis of phytosterol esters. The specificity of different lipases or the same kind of lipases with different sources to the catalytic substrates is also different, so it is critical to select suitable lipases. In this study, five kinds of common lipases, including Lipozyme RM IM, Lipozyme TL IM, CALA, Novozym 435 and CRL, were selected for the synthesis of phytosterol oleic esters under the same reaction conditions (30 °C, 8 wt% enzyme loading, 1
:
1.5 molar ratio of phytosterols to fatty acids, 2 mL isooctane).
The effect of different lipases on the conversion of phytosterols is shown in Fig. 1. The five lipases displayed significant differences in the catalytic efficiency under the same reaction conditions. Among these bio-catalysts, only CRL exhibited high catalytic activity on the esterification of phytosterols with fatty acids from high-oleic sunflower seed oil, while Lipozyme RM IM, Lipozyme TL IM, Novozym 435 and CALA showed relatively low catalytic activity. Specifically, the conversion of phytosterols was up to 92.2% when employing CRL as the catalyst, whereas only 8.8%, 12.4%, 10.8% and 6.3% conversions were obtained with Lipozyme RM IM, Lipozyme TL IM, Novozym 435 and CALA, respectively. The difference may be attributed to the stronger substrate specific selection of CRL.1 The results indicated that the esterification of phytosterols with fatty acids catalyzed by CRL was a substrate-dependent enzyme reaction. Based on the above results, CRL was selected as the optimal bio-catalyst in the following experiments.
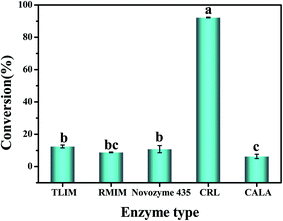 |
| Fig. 1 Conversion of phytosterols catalyzed by different enzymes. Reaction conditions: 30 °C, 120 min, 8% enzyme loading, 1 : 1.5 molar ratio of phytosterols to fatty acids, 2 mL isooctane. | |
3.3 Effect of solvents
The reaction solvent is one of the most important factors of esterification or transesterification catalyzed by lipase in non-aqueous medium. On the one hand, the properties of the solvents can affect the mass transfer in the reaction system by changing the solubility of the substrates.29 On the other hand, organic solvents have great influence on the structure of the enzymes, thus affecting the activity and stability of lipase.30,31 Therefore, five common non-polar organic solvents, n-hexane, isooctane, cyclohexane, toluene and acetone were evaluated. As shown in Fig. 2(a), CRL had better catalytic activity in n-hexane, cyclohexane and isooctane, while exhibiting relatively low catalytic activity in toluene and acetone, which might be related to the hydrophobicity of the solvents. The hydrophobicity of a solvent can be represented by its log
P value, which is defined as the logarithm of the partition coefficient of a given compound in the standard two-phase system of octanol/water. The larger the log
P value is, the more hydrophobic the solvent. The log
P values of the five solvents are shown in Table 2. Isooctane, with a log
P value of 4.7, had the strongest hydrophobicity and afforded the highest (92.2%) conversion of phytosterols (Fig. 2(a)). The high conversion was obtained in a solvent with strong hydrophobicity because the enzyme structure and active center were not destroyed, and still retained high activity. During the reaction, hydrophilic solvents (e.g., acetone) would take away the necessary water on the surface of lipase, resulting in the decrease or even loss of enzyme activity. Therefore, the catalytic activity of CRL was poor in solvents with large polarity (such as toluene and acetone), and the conversion of phytosterols was only 30.3% and 20%, respectively. Although the log
P value of n-hexane (3.5) was higher than that of cyclohexane (3.2), the conversion of phytosterols was slightly lower than that of cyclohexane, which might be because the solubility of phytosterols in n-hexane was lower than that of cyclohexane.21 Therefore, isooctane was selected as the solvent for the subsequent reactions.
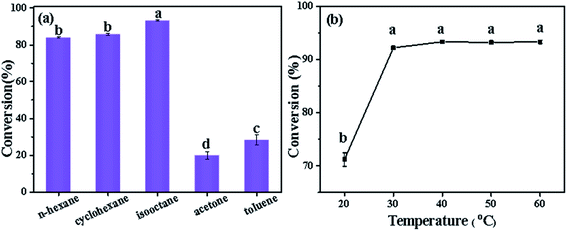 |
| Fig. 2 (a) Effect of solvents on the esterification of phytosterols. Reaction conditions: 30 °C, 120 min, 8% enzyme loading, 1 : 1.5 molar ratio of phytosterols to fatty acid, 2 mL solvent. (b) Effect of the reaction temperature on the esterification of phytosterols. Reaction conditions: 120 min, 8% enzyme loading, 1 : 1.5 molar ratio of phytosterols to fatty acid, 2 mL isooctane. | |
Table 2 The hydrophobicity index of different organic solvents
Solvents |
Isooctane |
n-Hexane |
Cyclohexane |
Toluene |
Acetone |
log P |
4.7 |
3.5 |
3.2 |
2.39 |
−0.23 |
3.4 Effect of the reaction temperature
Temperature is a crucial factor that not only affects the reaction rate, but also lipase activity. In general, much higher temperature will lead to the loss of enzyme biological activity during enzyme-catalyzed reactions, including esterification and transesterification. Therefore, the effect of the reaction temperature from 20 °C to 60 °C on the esterification of phytosterols was investigated using CRL as the catalyst (Fig. 2(b)). When the reaction temperature was 20 °C (room temperature), the conversion of phytosterols was 71.2%. With the increase of the reaction temperature, the conversion of phytosterols was increased sharply and reached its maximum at 40 °C, with an excellent conversion of 93.3%. Further increasing the reaction temperature from 40 °C to 60 °C, there was no significant difference in their conversions at equilibrium. Thus, 40 °C was selected as the suitable reaction temperature for subsequent studies.
3.5 Effect of the molar ratio of substrates
The influence of the molar ratio of the substrates (phytosterols/fatty acids) on the esterification was investigated from 1
:
1 to 1
:
4, and the results are shown in Fig. 3(a). Theoretically, an equimolar ratio of both substrates should be ideal, considering the economic cost and subsequent purification. However, direct esterification is a reversible reaction, implying that increasing the amount of one reactant can promote the reaction to move towards esterification. Currently, the price of high-oleic sunflower seed oil is lower than that for olive oil and high-oleic peanut oil. Thus, oleic acid made from high-oleic sunflower seed oil was more economic and suitable for industrialization.
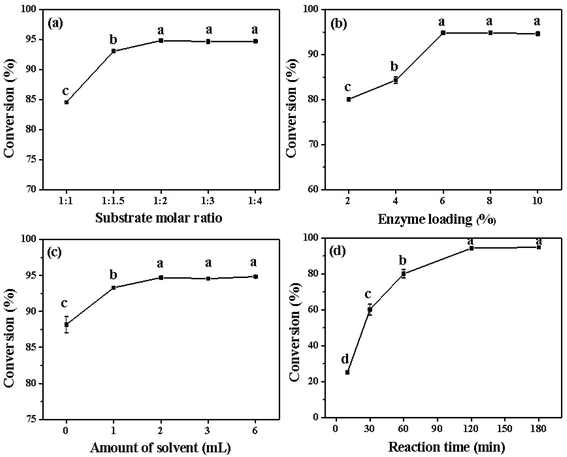 |
| Fig. 3 (a) Effect of the molar ratio of substrates (phytosterols/fatty acid) on the esterification of phytosterols. Reaction conditions: 40 °C, 120 min, 8% enzyme loading, 2 mL isooctane. (b) Effect of enzyme loading on the esterification of phytosterols. Reaction conditions: 40 °C, 120 min, 1 : 2 molar ratio of phytosterols to fatty acids, 2 mL isooctane. (c) Effect of the amount of isooctane on the esterification of phytosterols. Reaction conditions: 40 °C, 120 min, 6% enzyme loading, 1 : 2 molar ratio of phytosterols to fatty acids. (d) Effect of the reaction time on the esterification of phytosterols. Reaction conditions: 40 °C, 6% enzyme loading, 1 : 2 molar ratio of phytosterols to fatty acids, 2 mL isooctane. | |
Since fatty acids made from high-oleic sunflower seed oil were cheap and easy to remove after the reaction, excessive fatty acids were used in the esterification of phytosterols. As shown in Fig. 3(a), the conversion of phytosterols reached 84.6% when the molar ratio of phytosterols to fatty acids was 1
:
1. The conversion of phytosterols was further improved to 94.8% when the molar ratio of phytosterols to fatty acids was 1
:
2. With continued increasing of the molar ratio to 1
:
3 or 1
:
4, the conversions of phytosterols exhibited no noteworthy variation (94.7–94.8%). Therefore, a 1
:
2 molar ratio of phytosterols to fatty acids was selected as the suitable molar ratio for the following experiments.
3.6 Effect of enzyme loading
The amount of enzyme will directly affect the esterification reaction rate and equilibrium time. However, increasing the enzyme loading will greatly increase the cost due to the high cost of enzyme, which is undesirable from an economic perspective. Therefore, the effect of enzyme loading on the esterification of phytosterols was evaluated by varying the enzyme loading from 2% to 10% (Fig. 3(b)). Obviously, to some extent, the higher amount of CRL led to better conversion. The conversion of phytosterols was only 80.1% when the enzyme loading was 2%. When the enzyme loading was increased to 6%, the conversion of phytosterols approached 94.8%. Upon further increasing the amount of CRL (8–10%), 94.7–94.8% conversion of phytosterols was observed, suggesting that 6% of enzyme loading was sufficient to ensure the substrates fully reacted. Based on the above results, 6% was the optimal enzyme loading for the synthesis of phytosterol esters.
3.7 Effect of the amount of solvent
In the lipase-catalyzed reactions, the use of organic solvents as a reaction medium possesses several advantages, including increased solubility of substrates, better lipase stability, and inhibition of side reactions such as hydrolysis. The influence of the amount of solvent (isooctane) on the esterification reaction was investigated under the above optimal conditions, and the results are presented in Fig. 3(c). The conversion of phytosterols was only 88.1% without any organic solvents, which might be due to the insufficient substrate contact. Using isooctane as an organic solvent, the conversion of phytosterols was clearly increased. The conversion of phytosterols was 93.3% when 1 mL of isooctane was added to the esterification. The conversion of phytosterols reached 94.8% when the amount of organic solvent (isooctane) was 2 mL. Subsequently, no noteworthy variation (94.6%, 94.8%) was observed when the amount of isooctane was increased from 3 mL to 6 mL. Therefore, 2 mL was selected as the optimal amount of isooctane for subsequent studies.
3.8 Effect of the reaction time
The effect of the reaction time on the esterification of phytosterols with fatty acids from high-oleic sunflower seed oil is shown in Fig. 3(c). In the early stage of the reaction, the conversions of phytosterols presented a rapid increase with the extension of reaction time (0–60 min). In detail, the conversion of phytosterols reached 25.1% for 10 min, and then approached 80.1% for 60 min. Subsequently, the conversion of phytosterols was increased slowly with the prolonging of the reaction time, and reached 94.8% for 120 min. Furthermore, there was no significant difference on the conversion of phytosterols when further prolonging the reaction time (180 min), indicating that the esterification reaction of the phytosterols reached equilibrium. Indeed, the CRL-catalyzed synthesis of the phytosterol esters with fatty acids from high-oleic sunflower seed oil required much less reaction time (2 h) than that in previous reports (17–72 h).15,16,18 Thus, the optimal reaction time was 120 min.
3.9 Model fitting
In the response surface experiment, the effects of the reaction temperature, substrate molar ratio and enzyme loading on the conversion of phytosterols were studied. The design scheme and corresponding experimental data are shown in Table S1 in ESI.† The model of the phytosterol conversion was analyzed by multiple regression analysis (Table 3). The coefficient of the regression models (R2) was 0.9987, indicating that there was a high correlation between the predicted value and the actual value. The significance of each factor on the response value can be judged by the P value. The quadratic polynomial regression model of phytosterol conversion was very significant (p < 0.0001), and lack of fit was not significant (p > 0.05), suggesting that the regression equation was well fitted. These results also illustrated that the mathematical model was suitable and reliable for the analysis and prediction of phytosterol conversion.
Table 3 ANOVA for the quadratic models
Source |
Sum of squares |
Degrees of freedom |
Mean square |
F value |
P > F |
Model |
827.19 |
9 |
91.91 |
174.95 |
<0.0001 |
X1 |
186.05 |
1 |
186.05 |
354.14 |
<0.0001 |
X2 |
322.83 |
1 |
322.83 |
614.50 |
<0.0001 |
X3 |
64.07 |
1 |
64.07 |
121.96 |
<0.0001 |
X1X2 |
52.20 |
1 |
52.20 |
99.36 |
<0.0001 |
X1X3 |
38.25 |
1 |
38.25 |
72.82 |
<0.0001 |
X2X3 |
7.98 |
1 |
7.98 |
15.19 |
0.0059 |
X12 |
4.21 |
1 |
4.21 |
8.01 |
0.0254 |
X22 |
117.37 |
1 |
117.37 |
223.41 |
<0.0001 |
X32 |
23.85 |
1 |
23.85 |
45.39 |
0.0003 |
Residual |
3.68 |
7 |
0.53 |
|
|
Lack of fit |
2.93 |
3 |
0.98 |
5.26 |
0.0712 |
Pure error |
0.74 |
4 |
0.19 |
|
|
Total |
830.87 |
16 |
|
|
|
R2 = 0.9987 |
|
|
|
|
|
The regression equations for the models of phytosterol conversion were successfully obtained as follows:
Phytosterol conversion (%) = −79.05 + 2.93A + 46.16B + 16.15C − 0.36AB − 0.15AC − 0.71BC − 0.01A2 − 5.28B2 − 0.59C2 |
Three-dimension response surfaces were made to study the relationship between different variables and the response. Fig. 4(A–C) showed the interaction influence of the reaction temperature, substrate molar ratio and enzyme loading on the conversion of phytosterols. The linear terms of A (temperature), B (substrate molar ratio) and C (enzyme loading) were all extremely significant to the conversion of phytosterols. The quadratic term A2 had a significant influence (0.01 ≤ P < 0.05), and B2 and C2 had extremely significant impacts on the conversion (P < 0.01). The interaction terms AB, AC and BC all had a significant effect on the conversion (P < 0.01). The influence of the three factors on esterification was in the order of substrate molar ratio > temperature > enzyme loading. The maximum phytosterol conversion was obtained in the range of reaction temperature 45–50 °C, substrate molar ratio (phytosterols/fatty acids) of 1
:
2.1–1
:
2.5 and enzyme loading of 5%–7%.
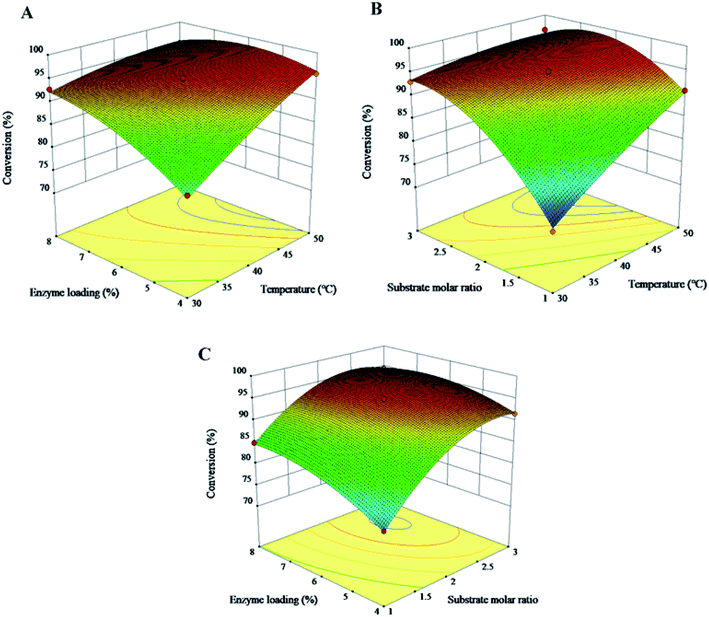 |
| Fig. 4 Response surfaces for phytosterol conversion using CRL as a catalyst: (A) varying temperature and enzyme loading; (B) varying temperature and substrate molar ratio; (C) substrate molar ratio and enzyme loading. | |
3.10 Optimization of reaction conditions and model verification
According to the response surface methodology (RSM) analysis, the optimum conditions were as follows: temperature 50 °C, substrate molar ratio (phytosterols
:
fatty acids) 1
:
2.3, enzyme loading of 5.8%, isooctane volume of 2 mL, and reaction time of 120 min. Under these conditions, the maximum conversion of phytosterols (96.8%) was obtained, which was in agreement with the predicted value (98.7%), indicating the exact fitting effect of the model. These results suggested that the predicted model was reliable.
3.11 HPLC analysis of phytosterol esters
Under the optimal reaction conditions, 96.8% conversion of phytosterols and 92% isolated yield of phytosterol esters were obtained, indicating that a few by-products were generated during the esterification of the phytosterols using CRL as a bio-catalyst. HPLC analysis was employed to calculate the conversion of phytosterols, and the HPLC-UV chromatogram of a typical phytosterol ester sample is shown in Fig. 5. Reaction substrates (phytosterols) and esterification products (phytosterol esters) could be clearly distinguished by HPLC method with retention times of 4.0 min and 8.2 min, respectively. Under the optimal reaction conditions, the peak of the phytosterols almost disappeared, while a new group of phytosterol ester peaks appeared.
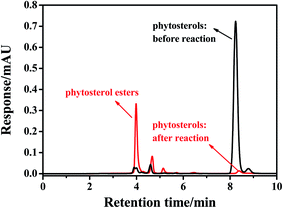 |
| Fig. 5 The HPLC-UV chromatogram of the esterification products. | |
3.12 Comparison with enzymatic method reported in the literature
Since the CRL-catalyzed preparation of the phytosterol esters with fatty acids from high-oleic sunflower seed oil required much less reaction time (2 h) than that in previous reports (17–72 h), Table 5 compared the reaction conditions for the esterification of phytosterols with different fatty acids (e.g., oleic acid) catalyzed by CRL. Indeed, the esterification involving fatty acids from saturated fatty acid (e.g., caprylic acid, lauric acid) to unsaturated fatty acids (e.g., oleic acid) required a much longer esterification reaction time to reach a satisfactory degree of conversion (>80%). In most cases, a longer reaction time (>24 h) is one of the limitations for enzymatic esterification for large-scale preparation. Therefore, developing an enzymatic preparation of phytosterol esters with less reaction time (2–6 h) is highly desirable, which will extend the applications in the food industry.
Were the differences of our work with reported examples (Table 4) only using isooctane as a solvent in the presence of CRL? Under the optimized reaction conditions (50 °C, 1
:
2.3 molar ratio of phytosterols/fatty acids, 5.8% of enzyme loading, 2 mL isooctane, 120 min), the catalytic effect of CRL on the esterification of phytosterols with other fatty acids was also investigated (Table 5). The esterification of phytosterols with saturated fatty acids, such as palmitic acid (C16:0) and stearic acid (C18:0), could afford the corresponding phytosterol esters in higher conversion of 99.7% and 99.6%, respectively (Table 5, entries 1 and 2). Moreover, oleic acid obtained from different resources had been also evaluated. Commercially available oleic acid (85% purity, industrial grade) could afford a similar conversion of 95.3% (Table 5, entry 4). Furthermore, the much higher purity of oleic acid (>95%) may have some positive effect on the esterification. Thus, oleic acid (96% purity) was obtained through urea-inclusion method using fatty acids from the hydrolysis of high-oleic sunflower seed oil in this work. Then, the oleic acid with 96% purity was used as a substrate, and a conversion of 97.5% was detected (Table 5, entry 5).
Table 4 Comparison of CRL-catalyzed esterification of phytosterols with fatty acids (FA)
Substrate (FA) |
Reaction parametersa |
Conversion |
Ref. |
A/S: molar ratio of acids to phytosterols. |
Oleic acid |
30 °C, 5 : 1 A/S, 2000 U mL−1, 24 h |
80% |
16 |
Oleic acid |
n-Hexane, 51.3 °C, 2.1 : 1 A/S, 7.2% lipase, 17 h |
97% |
15 |
Oleic acid |
n-Hexane, 35 °C, 3 : 1 A/S, 5% lipase, 72 h |
85% |
18 |
Caprylic acid |
n-Hexane, 45 °C, 2.15 : 1 A/S, 7.9% lipase, 9.2 h |
98% |
32 |
Cotton seed oil deodorizer distillate |
n-Heptane, 44.2 °C, 3 : 1 A/S, 0.84% lipase, 12 h |
90.8% |
20 |
Lauric acid |
Water-in-[Bmim]PF6, 50 °C, 2 : 1 A/S, 10% lipase, pH 7.4, 48 h |
95.1% |
17 |
Fatty acids from high-oleic sunflower seed oil |
Isooctane, 50 °C, 2.3 : 1 A/S, 5.8% lipase, 2 h |
96.8% |
This work |
Table 5 CRL-catalyzed esterification of phytosterols with different fatty acids
Entry |
Fatty acid |
Conversion (%) |
Oleic acid (84%) resulting from the hydrolysis of high-oleic sunflower seed oil. Oleic acid (85%) was industrial grade. Oleic acid (96.2%) was obtained through urea-inclusion method using fatty acids from the hydrolysis of high oleic sunflower seed oil. The substrate contained 96.2% oleic acid and 3.8% linoleic acid. Oleic acid (99%) was GC grade. |
1 |
Palmitic acid (C16:0) |
99.7 ± 0.1 |
2 |
Stearic acid (C18:0) |
99.6 ± 0.1 |
3 |
Oleic acid (C18:1)a |
96.8 ± 0.1 |
4 |
Oleic acid (C18:1)b |
95.4 ± 0.9 |
5 |
Oleic acid (C18:1)c |
97.5 ± 0.7 |
6 |
Oleic acid (C18:1)d |
25.0 ± 2.0 |
It is worth noting that the oleic acid with 99% purity (commercially available) as a substrate afforded a lower conversion of 25.0% (Table 5, entry 6). In this study, the efficiency of the synthesis of phytosterol oleic ester catalyzed by CRL was much higher than reported results (Table 4).15,16,18 Based on the above control experiments (Table 5, entries 3–6), it was possible due to the fatty acids used in this experiments (Table 5, entries 3–5) containing trace components of lipid compounds, which when used as substrates, could improve the enzyme activity of CRL and thus greatly accelerate the esterification reaction. Endo et al. reported a method in a patent that mixed phospholipids, fat-soluble vitamins and lipases, and could improve the lipases stability.33 Another patent showed that using triglycerides (edible oils) to wash Lipozyme TL IM could recover its activity, and the lipase could be recycled and used for a number of times.34 In general, the above results still suggested that the CRL-catalyzed esterification of phytosterols with fatty acids from high-oleic sunflower seed oil could provide phytosterol oleic esters with excellent conversion and shorter reaction time.
4. Conclusions
In this study, phytosterol oleic acid esters was successfully synthesized by CRL-catalyzed esterification of phytosterols with fatty acids made from high-oleic sunflower seed oil (oleic acid 84%) in isooctane. A maximum phytosterol conversion of 96.8% and phytosterol ester yield of 92% were obtained under the following optimal reaction conditions: 50 °C for the reaction temperature, 120 min for the reaction time, 1
:
2.3 molar ratio of phytosterols to fatty acids, 5.8% enzyme loading and 2 mL isooctane. This CRL-catalyzed preparation of phytosterol esters with fatty acids from high-oleic sunflower seed oil required much less reaction time (2 h) than that in the literature. This work provides a simple and efficient production method for the preparation of unsaturated fatty acid phytosterol esters with good oxidative stability.
Conflicts of interest
There are no conflicts to declare.
Acknowledgements
This work was supported by the Key Scientific and Technological project in Henan Province (No. 192102110211) and China Postdoctoral Science Foundation funded project (No. 2018T110730).
References
- W. S. He, H. Zhu and Z. Y. Chen, J. Agric. Food Chem., 2018, 66, 3047–3062 CrossRef CAS PubMed.
- R. Sobhy, F. C. Zhan, E. Mekawi, I. Khalifa, H. S. Liang and B. Li, Bioorg. Chem., 2020, 94, 103478 CrossRef CAS PubMed.
- R. Sobhy, I. Khalifa, H. S. Liang and B. Li, Bioorg. Chem., 2020, 101, 104047 CrossRef CAS PubMed.
- R. Sobhy, Q. Shen, A. A. Abd-Elrahman, I. Khalifa, H. S. Liang and B. Li, Steroids, 2020, 161, 108678 CrossRef CAS PubMed.
- J. C. Carles Escola-Gil, Curr. Med. Chem., 2019, 26, 6688–6690 CrossRef PubMed.
- R. Chawla, S. Sivakumar and N. Goel, Asian Journal of Dairy and Food Research, 2016, 35, 217–226 CrossRef.
- H. Vaikousi, A. Lazaridou, C. G. Biliaderis and J. Zawistowski, J. Agric. Food Chem., 2007, 55, 1790–1798 CrossRef CAS PubMed.
- N. Weber, P. Weitkamp and K. D. Mukherjee, J. Agric. Food Chem., 2001, 49, 67–71 CrossRef CAS PubMed.
- W. Liu, B. Xiao, X. P. Wang, J. N. Chen and G. L. Yang, RSC Adv., 2021, 11, 10738–10746 RSC.
- Z. Tan, K. Le, M. Moghadasian and F. Shahidi, Food Chem., 2012, 134, 2097–2104 CrossRef CAS PubMed.
- Y. Pouilloux, G. Courtois, M. Boisseau, A. Piccirilli and J. Barrault, Green Chem., 2003, 5, 89–91 RSC.
- X. H. Meng, Q. Y. Pan and T. K. Yang, J. Am. Oil Chem. Soc., 2011, 88, 143–149 CrossRef CAS.
- Q. C. Deng, P. Zhang, Q. D. Huang, F. H. Huang, F. Wei, M. M. Zheng, X. Yu, Q. Zhou and C. Zheng, Eur. J. Lipid Sci. Technol., 2011, 113, 441–449 CrossRef CAS.
- Y. Yang, W. He, C. Jia, Y. Ma, X. Zhang and B. Feng, J. Mol. Catal. A: Chem., 2012, 357, 39–43 CrossRef CAS.
- B. H. Kim and C. C. Akoh, Food Chem., 2007, 102, 336–342 CrossRef CAS.
- T. Kobayashi, A. Ogino, Y. Miyake, H. Mori, A. Hosoda, M. Fujita, T. Tsuno and S. Adachi, J. Am. Oil Chem. Soc., 2014, 91, 1885–1890 CrossRef CAS.
- C. X. Zeng, S. J. Qi, Z. G. Li, R. M. Luo, B. Yang and Y. H. Wang, Bioprocess Biosyst. Eng., 2015, 38, 939–946 CrossRef CAS PubMed.
- P. Villeneuve, F. Turon, Y. Caro, R. Escoffier, B. Baréa, B. Barouh, R. Lago, G. Piombo and M. Pina, Enzyme Microb. Technol., 2005, 37, 150–155 CrossRef CAS.
- M. M. Zheng, Y. Lu, F. H. Huang, L. Wang, P. M. Guo, Y. Q. Feng and Q. C. Deng, J. Agric. Food Chem., 2013, 61, 231–237 CrossRef CAS PubMed.
- X. Y. Zhang, J. Yu and A. W. Zeng, Biotechnol. Appl. Biochem., 2017, 64, 270–278 CrossRef CAS PubMed.
- W. S. He, D. D. Cui, Y. L. Zhang, Y. Liu, J. Yin, G. Chen, C. S. Jia and B. Feng, Food Sci. Technol. Res., 2017, 23, 525–533 CrossRef CAS.
- M. M. Zheng, L. J. Mao, F. H. Huang, X. Xiang, Q. C. Deng and Y. Q. Feng, RSC Adv., 2015, 5, 43074–43080 RSC.
- C. Y. Shang, W. X. Li and R. F. Zhang, Mater. Res. Bull., 2015, 68, 336–342 CrossRef CAS.
- M. Raczyk, D. Kmiecik, R. Przybylski and M. Rudzińska, J. Am. Oil Chem. Soc., 2017, 94, 701–711 CrossRef CAS PubMed.
- J. N. Chen, G. Y. Tang, J. F. Zhou, W. Liu and Y. L. Bi, RSC Adv., 2019, 9, 40109–40117 RSC.
- E. Séverac, O. Galy, F. Turon, P. Monsan and A. Marty, Bioresour. Technol., 2011, 102, 4954–4961 CrossRef PubMed.
- M. Kasprzak, M. Rudzińska, D. Kmiecik, R. Przybylski and A. Olejnik, Food Chem. Toxicol., 2019, 136, 111074 CrossRef PubMed.
- S. B. Smith, D. K. Lunt, D. R. Smith and R. L. Walzem, Meat Sci., 2020, 163, 108076 CrossRef CAS PubMed.
- M. Miao, H. Liu, B. Jiang, C. Yang, X. L. Xia and T. Zhang, J. Funct. Foods, 2014, 7, 452–461 CrossRef CAS.
- X. X. Pan, B. Q. Chen, J. Wang, X. Z. Zhang, B. Y. Zhul and T. W. Tan, Appl. Biochem. Biotechnol., 2012, 168, 68–77 CrossRef CAS PubMed.
- D. Herbst, S. Peper and B. Niemeyer, J. Biotechnol., 2012, 162, 398–403 CrossRef CAS PubMed.
- Z. Tan and F. Shahidi, J. Am. Oil Chem. Soc., 2012, 89, 657–666 CrossRef CAS.
- Y. Endo and R. Minoshima, Thermally stable enzyme and production thereof, JP2000106873A, 2000.
- S. Negishi, J. Suzuki and Y. Yamauchi, Method for recovery of lipase activity, EP2019135A1, 2013.
Footnote |
† Electronic supplementary information (ESI) available. See DOI: 10.1039/d1ra01486b |
|
This journal is © The Royal Society of Chemistry 2021 |
Click here to see how this site uses Cookies. View our privacy policy here.