DOI:
10.1039/D1RA01408K
(Paper)
RSC Adv., 2021,
11, 23597-23606
A novel coumarin-based colorimetric and fluorescent probe for detecting increasing concentrations of Hg2+ in vitro and in vivo†
Received
21st February 2021
, Accepted 25th June 2021
First published on 5th July 2021
Abstract
Mercury has complex biological toxicity and can cause a variety of physiological diseases and even death, so it is of great importance to develop novel strategies for detecting trace mercury in environmental and biological samples. In this work, we designed a new coumarin-based colorimetric and fluorescent probe CNS, which could be obtained from inexpensive starting materials with high overall yield in three steps. Probe CNS could selectively respond to Hg2+ with obvious color and fluorescence changes, and the presence of other metal ions had no effect on the fluorescence changes. Probe CNS also exhibited high sensitivity against Hg2+, with a detection limit as low as 2.78 × 10−8 M. More importantly, the behavioral tracks of zebrafish had no obvious changes upon treatment with 10 μM probe CNS, thus indicating its low toxicity. The probe showed potential application value and was successfully used for detecting Hg2+ in a test strip, HeLa cells and living zebrafish larvae.
1. Introduction
Mercury (Hg) is one of the most toxic heavy-metal ions in the environment and has attracted increasing attention due to its accumulation in natural ecosystems.2–4 Generally, mercury exists as elemental mercury, oxidized mercury (Hg2+) and mercury particles in air, soil and water.5–7 All these mercury species are not biodegradable and can be concentrated in the human body, thereby causing irreversible damage to the liver, kidneys, brain, and endocrine and central nervous systems.8–11 In particular, Hg2+ can be easily converted into the lipophilic methyl mercury, which exhibits good membrane penetrating ability and can be easily absorbed by aquatic organisms.12–14 Recently, mercury chloride and methyl mercury have been classified as potentially carcinogenic agents by the Environmental Protection Agency.9 Therefore, development of novel strategies for selectively and sensitively detecting trace mercury in environmental and biological samples is of great importance.
Several methods have been developed for detecting mercury species, such as atomic absorption–emission spectrometry,15,16 inductively coupled plasma-atomic emission spectrometry,17 and anodic stripping voltammetry.18 However, these methods always suffer from complicated manipulation procedure and expensive instrumentation.1,14 In contrast, fluorescent probes have attracted increasing attention because of their low cost, high sensitivity and selectivity, noninvasiveness, and experimental convenience.19–22 In the past several years, great efforts have been made on the development of fluorescent probes for mercury ions based on the heteroatom-based coordination reaction, and Hg2+-promoted elimination and desulfurization reactions.23–33 A portion of them exhibit excellent selectivity and sensitivity, fast response time, and low detection limits. However, these reported fluorescent probes still have limitations including complex synthesis routine, using expensive chemical regents, high toxicity, and poor biocompatibility, thus being not applicable for detecting Hg2+ in biological systems and environmental samples.
Coumarin is a well-known secondary metabolite found in different parts of plants.34 Structural modification of coumarin can afford various derivatives with various pharmacological activities and low toxicity to human body.35 Some of these coumarin derivatives exhibit strong fluorescent emission and can be used as fluorescent probes for imaging different metal ions and metabolites in living biological systems.35,36 Coumarin derivatives can also be used as fluorescent tags or imaging agents for discriminating tumor lesions and investigating the subcellular localization of drugs.37–46 In our research, a novel intramolecular charge transfer (ICT)-based fluorescent probe CNS (Scheme 1), employing coumarin as the fluorophore and thioacetals as the reacting site for Hg2+, was constructed by a facile and highly efficient synthesis strategy. The probe should be highly selective and sensitive to Hg2+, because the electron-rich dithioacetal group can be specifically and rapidly cleaved in the presence of Hg2+. The optical properties and probing behaviors of CNS against Hg2+, and its applications in living cells and zebrafish were investigated.
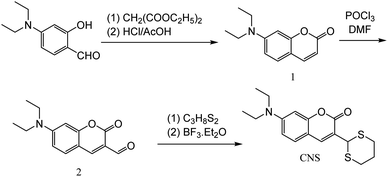 |
| Scheme 1 Synthesis step of probe CNS. | |
2. Experimental
2.1 Materials and measurements
All chemical reagents were obtained commercially and used without further purification. 1H NMR and 13C NMR spectra were recorded on a Bruker AV-400 spectrometer using TMS as internal standard. Electrospray ionization (ESI) mass spectra were recorded by an LC-MS 2010A (Shimadzu) instrument. The high-resolution mass spectrum (HRMS) was measured using a Q-TOF6510 spectrograph (Agilent). The UV-vis absorption spectra were analyzed by a UV-2600 PC spectrophotometer (Shimadzu, Japan). The fluorescence spectra were recorded on a F-7000 Fluorescence Spectrophotometer (Hitachi, Japan).
2.2 Synthesis of probe CNS
2.2.1 Synthesis of compound 1. To a solution of 4-diethylaminosalicylaldehyde (3.86 g, 20 mmol) in ethyl alcohol (120 mL) was added diethyl malonate (7 mL, 25 mmol), piperidine (2.0 mL, 0.02 mmol) and two drops of acetic acid. The reaction was heated to reflux and stirred for 6 h. Then all volatiles were evaporated under reduced pressure, and then concentrated. HCl (40 mL) and acetic acid (40 mL) were added and the reaction was continued at 110 °C temperature for 24 h. This solution was cooled to room temperature and poured into ice water (150 mL). NaOH solution (30%) was added dropwise to adjust the pH to 6, and a brown precipitate formed immediately. After stirring for 1 h, the mixture was filtered, washed with water, purified through a flash silica gel column by the using DCM as eluent to afford compound 1. 1H NMR (400 MHz, DMSO-d6) δ 7.69 (d, J = 9.3 Hz, 1H), 7.29 (d, J = 8.8 Hz, 1H), 6.55 (dd, J = 8.8, 2.5 Hz, 1H), 6.38 (d, J = 2.4 Hz, 1H), 5.85 (d, J = 9.3 Hz, 1H), 3.29 (q, J = 7.0 Hz, 4H), 0.98 (t, J = 7.0 Hz, 6H) (Fig. S1†).
2.2.2 Synthesis of compound 2. Dry DMF (3 mL) was added dropwise to POCl3 (3 mL) 0 °C, the mixture was stirred for 10 min and slowly added to a solution of 1 (2.17 g, 10 mmol) in dry DMF (20 mL). The resulting mixture was stirred at 60 °C for 24 h and then poured into ice water (150 mL). NaOH solution (30%) was added to adjust the pH to 7, and a large amount of precipitate was formed. The mixture was filtered and thoroughly washed with water, purified through a flash silica gel column to give compound 2 as an orange solid. 1H NMR (400 MHz, DMSO-d6) δ 9.77 (s, 1H), 8.29 (s, 1H), 7.56 (d, J = 9.0 Hz, 1H), 6.70 (dd, J = 9.0, 2.4 Hz, 1H), 6.48 (d, J = 2.4 Hz, 1H), 3.38 (q, J = 7.1 Hz, 4H), 1.02 (t, J = 7.0 Hz, 6H), 0.13 (s, 3H) (Fig. S2†).
2.2.3 Synthesis of CNS. Compound 2 (0.245 g, 1 mmol) was added to a 100 mL three-necked flask and dissolved in 20 mL ethyl alcohol. Then, to the mixture was added propane-1,3-dithiol (0.1 mL, 0.99 mmol) followed by a catalytic amount of BF3·Et2O. The reaction was heated to reflux and stirred for 2 h. The resulting mixture was cooled to room temperature and refrigerated for 30 minutes. The solvent was evaporated and the crude product was recrystallized by ethyl alcohol to afford CNS as a pale yellow solid (0.311 g. 75.05% for three steps). 1H NMR (400 MHz, DMSO-d6) δ 7.94 (s, 1H), 7.55 (d, J = 8.9 Hz, 1H), 6.71 (dd, J = 8.9, 2.5 Hz, 1H), 6.52 (d, J = 2.4 Hz, 1H), 5.35 (s, 1H), 3.44 (q, J = 7.0 Hz, 4H), 3.11 (ddd, J = 14.6, 12.3, 2.3 Hz, 2H), 2.89 (dt, J = 14.0, 3.7 Hz, 2H), 2.12 (ddd, J = 14.0, 4.5, 2.3 Hz, 1H), 1.79–1.52 (m, 1H), 1.12 (t, J = 7.0 Hz, 6H) (Fig. S3†). 13C NMR (101 MHz, DMSO-d6) δ 156.11, 151.31, 142.59, 130.16, 118.40, 109.69, 107.96, 96.71, 44.57, 43.31, 31.52, 25.23, 12.74 (Fig. S4†). HRMS (ESI): calcd for C17H21NO2S2: 335.1014, found: 336.1157 for [M + H]+(Fig. S5†).
2.3 Preparation of stock solutions
The CNS (3.51 mg, 1.0 mmol) was dissolved in THF and the volume was set to 100 mL to give the probe stock solution (1.0 × 10−3 M). The stock solutions of the metal ions with the concentration of 1.0 × 10−3 M were prepared by dissolving 1.0 mmol of each inorganic salt (AgNO3, Ba(NO3)2, Cd(NO3)2·4H2O, Co(NO3)2·6H2O, Cu(NO3)2·3H2O, FeSO4·7H2O, Fe(NO3)3·9H2O, Hg(NO3)2·H2O, KNO3, NaNO3, Ni(NO3)2·6H2O,Pb(NO3)2, Sr(NO3)2, Zn(NO3)2·6H2O) in water and the volume was set to 100 mL.
2.4 The UV-vis absorption spectra of CNS in the presence of different metal ions
The solution of CNS (1 mL) was placed in a 100 mL volumetric flask, and added 1.0 equiv. metal ions (Ag+, Ba2+, Cu2+, Co2+, Cd2+, Fe3+, Fe2+, K+, Na+, Ni2+, Pb2+, Sr2+, Zn2+ and Hg2+), constant to 100 mL with THF/H2O (1
:
1, v/v) solution, then get the 1.0 × 10−5 M fluid to be tested. The fluid to be tested were added to the quartz cell, and the corresponding ultraviolet spectrum was measured.
2.5 The fluorescence spectra of CNS in the presence of different metal ions
The solution of CNS (1 mL) was placed in a 100 mL volumetric flask, and added 1.0 equiv. metal ions (Ag+, Ba2+, Cu2+, Co2+, Cd2+, Fe3+, Fe2+, K+, Na+, Ni2+, Pb2+, Sr2+, Zn2+ and Hg2+), constant to 100 mL with THF/H2O (1
:
1, v/v) solution, then get the 1.0 × 10−5 M fluid to be tested. The fluid to be tested were added to the quartz cell, and the corresponding fluorescence spectrum was measured.
2.6 Competition experiments
The solution of CNS (1 mL) was placed in a 100 mL volumetric flask, 1 mL Hg2+ was added to the volumetric flask, and then added 1.0 equiv. other metal ions (Ag+, Ba2+, Cu2+, Co2+, Cd2+, Fe3+, Fe2+, K+, Na+, Ni2+, Pb2+, Sr2+, Zn2+), constant to 100 mL with THF/H2O (1
:
1, v/v) solution. The fluid to be tested were added to the quartz cell, and the fluorescence spectrum was measured.
2.7 Cell culture and fluorescence imaging
HeLa cells were grown in Dulbecco's Modified Eagle Medium (DMEM) containing 10% fetal bovine serum (FBS) and 1% penicillin–streptomycin. After incubating at 37 °C in a humidified atmosphere with 5% CO2 for 24 h, cells were washed with PBS buffer (phosphate buffered saline, pH = 7.2) and incubated with fresh medium containing 10 μM probe CNS for 30 min. Then, cells were treated with different concentrations of Hg2+ (0, 10, 20, and 50 μM) for 10 min and the fluorescence spectra were measured on a laser confocal microscopy (Olympus, Japan). For the control group, HeLa cells were grown in DMEM for 30 min without any treatment.
2.8 Zebrafish maintenance and fluorescence imaging
The wild type zebrafish eggs were grown in 24-well plate with E3 water containing 0.2 mM 2-phenylthiourea. After incubating in a light incubator at 28 ± 0.5 °C for 5 days, the zebrafish larvae were treated with new medium containing 10 μM probe CNS for 30 min. Then, different concentrations of Hg2+ (0, 5, 10, and 20 μM) were added to the medium, and the fluorescence spectra were measured on a laser confocal microscopy after 10 min of incubation. For the control group, the zebrafish larvae were grown in E3 water for 30 min without any treatment. The toxicity of probe CNS was measured using 5 days zebrafish larvae. Zebrafish larvae were treated with E3 water containing different concentrations of CNS (0, 5, and 10 μM) for 24 h, and the behavioral tracks of zebrafish were analyzed on an automated computerized video-tracking system (Viewpoint, Lyon, France).
3. Results and discussion
3.1 Selective recognition of Hg2+
The absorption spectra of probe CNS under the influence of various metal ions (Ag+, Ba2+, Cu2+, Co2+, Cd2+, Fe3+, Fe2+, K+, Na+, Ni2+, Pb2+, Sr2+, Zn2+ and Hg2+) were investigated in THF/H2O (1
:
1, V/V) solution (1.0 × 10−5 M), and the results were depicted in Fig. 1. The free probe CNS exhibited a strong absorption peak centered at 395 nm, which was shifted to 459 nm after adding 2.0 × 10−5 M Hg2+ ions. In contrast, addition of other metal ions (Ag+, Ba2+, Cu2+, Co2+, Cd2+, Fe3+, Fe2+, K+, Na+, Ni2+, Pb2+, Sr2+ and Zn2+) showed no effect on the absorption spectrum of CNS at the same concentration and under analogous test conditions. In addition, the probe solution turns yellow after adding Hg2+, while adding other metal ions induced no obvious color changes.
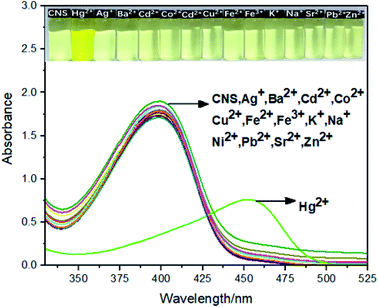 |
| Fig. 1 The absorption spectra of CNS upon addition of various metal ions in THF/H2O (1 : 1, V/V) solution (1.0 × 10−5 M). Inset: The photograph of CNS solution (1.0 × 10−5 M) in the presence of 1.0 × 10−5 M of Hg2+. | |
The fluorescence spectra of probe CNS upon treatment of various metal ions including Ag+, Ba2+, Cu2+, Co2+, Cd2+, Fe3+, Fe2+, K+, Na+, Ni2+, Pb2+, Sr2+, Zn2+ and Hg2+ were tested tin THF/H2O (1
:
1, V/V) solution (1.0 × 10−5 M). As shown in Fig. 2A, addition of Hg2+ ion to the solution of CNS in THF/H2O resulted in obvious fluorescence improvement and the maximum emission wavelength was 505 nm, whereas adding other metal ions resulted in negligible fluorescence improvement Fig. 2B. These results indicated the high selectivity of CNS toward Hg2+ over other tested metal ions.
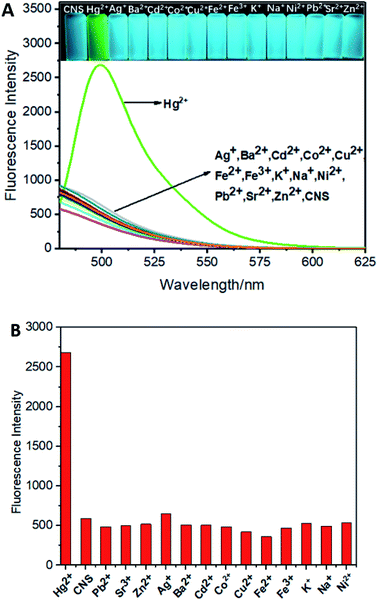 |
| Fig. 2 (A) The fluorescence spectra of CNS upon addition of various metal ions in THF/H2O (1 : 1, V/V) solution (1.0 × 10−5 M). (B) Comparison of Hg2+ and other metal ions detected by probe CNS. Inset: The photograph of CNS solution (1.0 × 10−5 M) in the presence of 1.0 × 10−5 M Hg2+ under the UV light. Excitation wavelength = 460 nm. | |
3.2 Sensitivity studies
The fluorescence titration experiments of CNS in THF/H2O (1
:
1, V/V) solution (1.0 × 10−5 M) against increasing concentrations of Hg2+ was carried out to investigate the fluorescence change of CNS under the influence of Hg2+. As depicted in Fig. 3A, probe CNS showed almost no fluorescence in THF/H2O (1
:
1, V/V) solution (1.0 × 10−5 M). After adding increasing concentrations of Hg2+ (0–14 μM), a significant fluorescence improvement could be observed around 505 nm, and the fluorescence intensity become stable when the concentration of Hg2+ was above 4 μM. These results proved the 1
:
1 response of probe CNS to Hg2+. The Job's plot of probe CNS with Hg2+ was further investigated in THF/H2O (1
:
1, V/V) solution (1.0 × 10−5 M), and the result was shown in Fig. 3B. The maximum fluorescence intensity was detected to be 0.5 (molar fraction of [Hg2+]/[CNS + Hg2+]), which proved a 1
:
1 stoichiometry for the reaction of probe CNS with Hg2+.
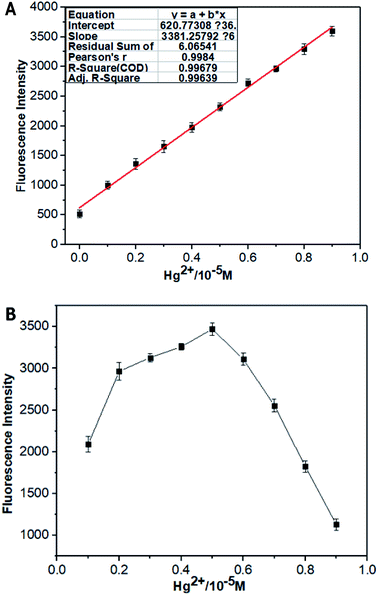 |
| Fig. 3 (A) The relative fluorescence intensity of CNS THF/H2O solution (10−5 M, 1 : 1, V/V) as a function of Hg2+ concentration in the range of 1 × 10−6 to 1 × 10−5 M. (B) Job's plot of CNS with Hg2+ ([CNS] + [Hg2+] = 10 M), THF/H2O (1 : 1, V/V) solution (1.0 × 10−5 M). Excitation wavelength = 460 nm. | |
3.3 Competition experiments
It is very necessary to study the anti-interference of probe CNS, as various metal ions are generally presented in environmental and biological samples. Consequently, the competition experiment was carried out to examine the binding ability of CNS toward other metal ions in THF/H2O (1
:
1, V/V) solution (1.0 × 10−5 M). As shown in Fig. 4, the fluorescence intensity increased significantly after the addition of Hg2+. In contrast, there were no obvious changes on the fluorescence intensity of CNS after adding various metal ions, which proved again the high selectivity of probe CNS toward Hg2+.
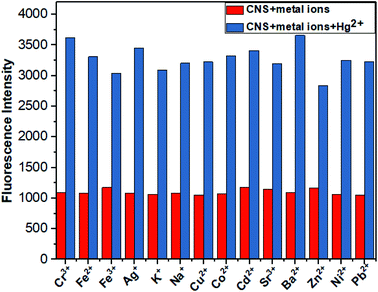 |
| Fig. 4 Competitive tests for Hg detection by probe CNS. Red indicates probe detection of other metal ions, blue indicates probe detection of CNS + other metal ions + Hg2+, THF/H2O solution (10−5 M, 1 : 1, V/V). Excitation wavelength = 460 nm. | |
3.4 pH effects
Next, we studied the fluorescent intensity of probe CNS in the presence and absence of Hg2+ under different pH conditions (Fig. 5). In the absence of Hg2+, the solution of probe CNS exhibited negligible fluorescence changes under a wide pH range,2–11 which indicated its high stability under acidic and basic conditions. After addition of Hg2+, the fluorescence intensity of probe CNS was significantly changed. However, the solution of probe CNS showed the maximum fluorescence intensity in the pH range from 3 to 8. This information revealed that probe CNS showed high stability and applicable to the biological scope.
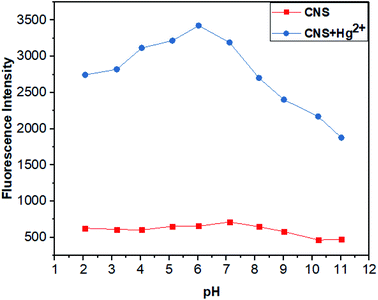 |
| Fig. 5 Fluorescence intensity of probe CNS (10−5 M) and CNS + Hg2+ (10−5 M) at different pH,2–11 THF/H2O solution (1 : 1, V/V). Excitation wavelength = 460 nm. | |
3.5 Response time of CNS
The response time of CNS to Hg2+ in THF/H2O solution (10−5 M, 1
:
1, V/V) was investigated and the results were depicted in Fig. 6. Clearly, the fluorescence intensity of probe CNS reached its maximum in 1 minute and remained stable for 5 minutes after adding Hg2+, demonstrating the fast responding time of CNS against Hg2+.
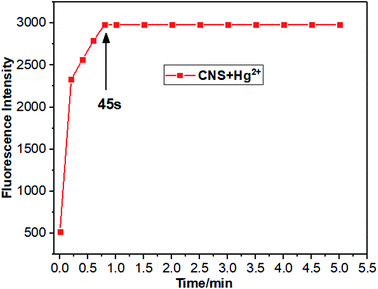 |
| Fig. 6 Response time and stability of probe CNS. Fluorescence intensity of CNS + Hg2+ (10−5 M), THF/H2O solution (1 : 1, V/V). Excitation wavelength = 460 nm. | |
Compared with other probes (Table 1), our probe has the fastest response time, and has a lower detection limit and a wider pH range. In terms of application, we have done cell experiments and zebrafish experiments, which fully demonstrates that our probe has a good application in biological detection.
Table 1 Comparison of CNS with other related fluorescent probes
Probe |
LOD (μM) |
pH range |
Response time |
Cell imaging |
Zebrafish imaging |
Ref. |
 |
2.1 |
No data |
No data |
No |
No |
47 |
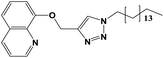 |
4.42 |
4–8 |
No data |
No |
No |
48 |
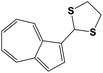 |
1.65 |
No data |
No data |
No |
No |
49 |
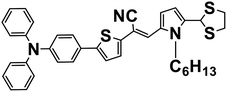 |
8 |
No data |
6 min |
Yes |
No |
50 |
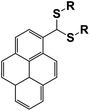 |
1.74/1.53 |
3–7.4 |
30 min |
Yes |
No |
51 |
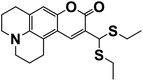 |
9 |
No data |
No data |
Yes |
No |
39 |
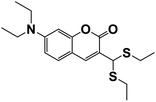 |
2.2 |
4–10 |
2 min |
Yes |
No |
52 |
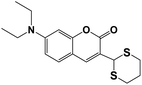 |
2.78 |
3–8 |
45 s |
Yes |
Yes |
This work |
3.6 Detection mechanism
To investigate the proposed sensing mechanism of probe CNS for Hg2+, we measured the 1H NMR spectra of probe CNS before and after reacting with Hg2+. As shown in Fig. 7, after adding Hg2+ to the solution of CNS in DMSO-d6, the original peaks at 3.48–3.27 and 3.23–3.12 ppm ascribed to the methylene protons of the thioacetal group disappeared, and a new peak at 9.65 ppm assigned to the aldehyde proton was observed. These results proved that the thioacetal group had been successfully eliminated under the promotion of Hg2+, which gave the corresponding aldehyde group and induced the intramolecular charge transfer (ICT) process, as shown in Scheme 2.
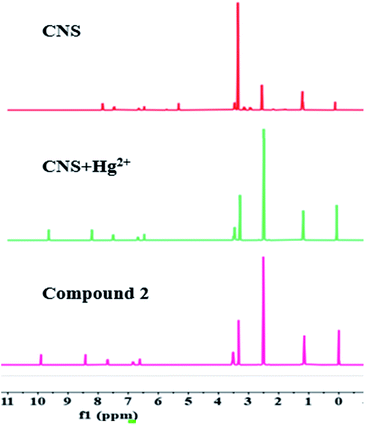 |
| Fig. 7 The 1H NMR of CNS, CNS + Hg2+ and compound 2. | |
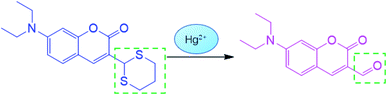 |
| Scheme 2 Recognition mechanism of CNS for Hg2+. | |
3.7 Paper test of probe CNS
A filter paper strip test was performed to study the convenience of probe CNS in practical application. Firstly, the filter paper was immersed in a probe solution (1.0 mM) and dried in air. Then the filter paper was immersed in a metal ion solution (1.0 mM). As shown in Fig. 8, the filter paper showed obvious color change only under the induction of Hg2+. The color change could be distinguished both under sunlight and 365 nm UV lamps. Therefore, the test bar can be used to test Hg2+ ion selectivity at any time.
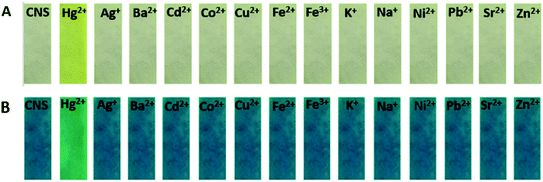 |
| Fig. 8 The selectivity of the metal ions was tested with a filter paper, (A) in sunlight and (B) in UV light at 365 nm. | |
3.8 Analytical applications in living cells
To investigate the biological applications of probe CNS, fluorescent imaging experiments were carried out in HeLa cells in the presence of 10 μM probe CNS and different concentrations of Hg2+ (0, 10, 20, and 50 μM). As depicted in Fig. 9, HeLa cells treated with 10 μM probe CNS for 30 min gave very weak fluorescence, which could be ascribed to the auto-fluorescence of probe CNS. To the medium was added 10 μM Hg2+ and the cells were incubated for 10 min, the fluorescence in HeLa cells were obviously improved, thereby indicating the chemical reaction between probe and Hg2+. With the increasing of Hg2+ concentration (20 μM and 50 μM), the fluorescence intensity in HeLa cells were significantly enhanced (Fig. 10). These results proved that CNS was a promising probe used for detecting Hg2+ in living cells, it could penetrate into HeLa cells in 30 min and rapidly respond to low concentrations of Hg2+.
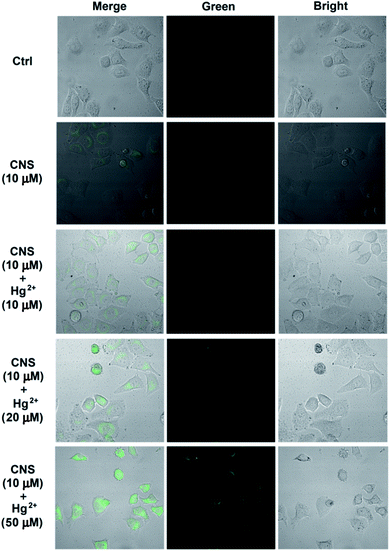 |
| Fig. 9 Confocal fluorescence images of Hg2+ in living cells: cells incubated with CNS (10 μM) for 30 min; probe-loaded cells incubated with different concentrations of Hg2+ (10 μM, 20 μM, 50 μM) for 10 min. Then the fluorescence spectra were measured on a laser confocal microscopy. Green channel images: 490 nm–550 nm. | |
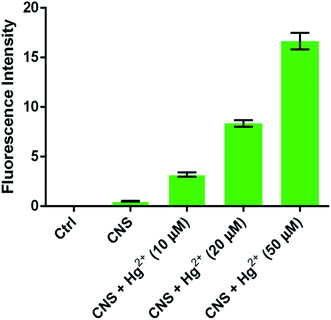 |
| Fig. 10 The improvement of fluorescence intensities from HeLa cells (fluorescence intensity was analyzed by Image J software). | |
3.9 Toxicity of probe CNS in zebrafish
The toxicity of probe CNS was investigated in zebrafish larvae by measuring their behavioral tracks on an automated computerized video-tracking system (Fig. 11). Generally, high toxicity compound can dramatically affect the behavioral tracks of zebrafish larvae, including swimming duration, movement distance, and swimming speed. In our experiments, the zebrafish larvae treated with 5 μM and 10 μM probe CNS for 24 h, and it seemed that probe CNS had no obvious effect on behavioral tracks of zebrafish larvae (Fig. 11A). There were no obvious decrease on the swimming duration (Fig. 11B), movement distance (Fig. 11C), and swimming speed (Fig. 11D). These data demonstrated that probe CNS exhibited very low toxicity at low concentrations.
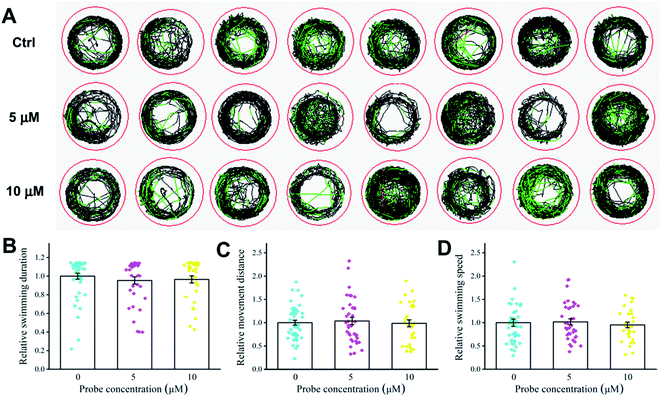 |
| Fig. 11 Effect of different concentrations of CNS (0, 5, and 10 μM) on behavioral tracks of zebrafish. (A) Behavioral tracks. Red, green, and black lines depict fast, medium, and slow movement, respectively. (B) Swimming duration, (C) movement distance, and (D) swimming speed of zebrafish larvae. | |
3.10 Analytical applications in zebrafish
We further investigated the biological applications of probe CNS in zebrafish before and after addition of difference concentrations of Hg2+. The 5 days zebrafish larvae were incubated in E3 water containing 10 μM probe CNS and different concentrations of Hg2+ (0, 5, 10, and 20 μM), then the fluorescence spectra were measured on a laser confocal microscopy. As shown in Fig. 12, the 5 days zebrafish larvae treated with E3 water containing 10 μM probe CNS for 30 min emitted weak fluorescence generated by the probe itself. After incubation with 5 μM Hg2+ for 10 min, the zebrafish larvae gave strong green fluorescence improvement, and the fluorescence intensity was significantly enhanced with the increasing of Hg2+ concentration (Fig. 13). All these results indicated that probe CNS could be used for monitoring low concentrations of Hg2+ in living zebrafish model.
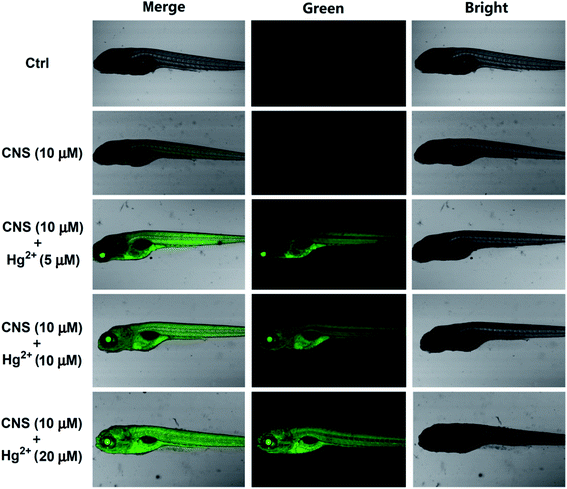 |
| Fig. 12 Confocal fluorescence images of Hg2+ in zebrafish: zebrafish incubated with CNS (10 μM) for 30 min; probe-loaded zebrafish incubated with different concentrations of Hg2+ (5 μM, 10 μM, 20 μM) for 10 min. Then the fluorescence spectra were measured on a laser confocal microscopy. Green channel images: 490 nm–550 nm. | |
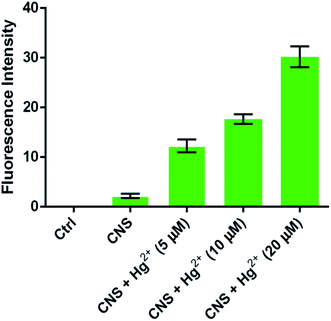 |
| Fig. 13 The improvement of fluorescence intensities from zebrafish larvae (fluorescence intensity was analyzed by Image J software). | |
4. Conclusions
In summary, we have obtained a new coumarin-based colorimetric and fluorescent probe CNS by a low cost and high yield synthesis route. Probe CNS exhibited excellent selectivity and sensitivity, fast response time, as well as good stability under physiological pH condition. In filter paper strip test, an obvious color change could be observed upon addition of Hg2+. The color change could be distinguished both under sunlight and 365 nm UV lamps, and the presence of other competitive metal ions had no obvious interference on the background. The probe showed low toxicity to zebrafish larvae, and was successfully used for detecting increasing concentrations of Hg2+ in HeLa cells and zebrafish model. All these results indicated that probe CNS should be a promising fluorescent agent for detecting Hg2+ in environmental samples and living biological systems.
Ethical statement
Animal procedures were conducted in compliance with the NIH Guide for the Care and Use of Laboratory Animals (No.8023, amended in 1996), and approved by the Animal Care and Use Committee of Qilu University of Technology that followed the guideline for the Care and Use of Laboratory Animals of China.
Conflicts of interest
The authors declare no competing financial interests.
Acknowledgements
This work was supported by the Key Research and Development Program of Shandong Province of China (2018 CXGC1106), and Young Science Foundation of Shandong Academy of Sciences (No. 2020QN0018).
References
- B. Zhou, S. Qin, B. Chen and Y. Han, A new BODIPY-based fluorescent “turn-on” probe for highly selective and rapid detection of mercury ions, Tetrahedron Lett., 2018, 59(49), 4359–4363 CrossRef CAS.
- S. Koenig, M. Solé, C. Fernández-Gómez and S. Díez, New insights into mercury bioaccumulation in deep-sea organisms from the NW Mediterranean and their human health implications, Sci. Total Environ., 2013, 442, 329–335 CrossRef CAS PubMed.
- H. Li, J. Zhai, J. Tian, Y. Luo and X. Sun, Carbon nanoparticle for highly sensitive and selective fluorescent detection of mercury(II) ion in aqueous solution, Biosens. Bioelectron., 2011, 26(12), 4656–4660 CrossRef CAS PubMed.
- H. Xiao, J. Li, K. Wu, G. Yin, Y. Quan and R. Wang, A turn-on BODIPY-based fluorescent probe for Hg(II) and its biological applications, Sens. Actuators, B, 2015, 213, 343–350 CrossRef CAS.
- R. Zou, X. Zeng, G. Luo, Y. Qiu, B. Zhang and Y. Xu, et al., Mercury stability of byproducts from wet flue gas desulfurization devices, Fuel, 2016, 186, 215–221 CrossRef CAS.
- H. Xu, Y. Ma, W. Huang, J. Mei, S. Zhao and Z. Qu, et al., Stabilization of mercury over Mn-based oxides: speciation and reactivity by temperature programmed desorption analysis, J. Hazard. Mater., 2017, 321, 745–752 CrossRef CAS PubMed.
- Y. Zhou, X. He, H. Chen, Y. Wang, S. Xiao and N. Zhang, et al., An ESIPT/ICT modulation based ratiometric fluorescent probe for sensitive and selective sensing Hg2+, Sens. Actuators, B, 2017, 247, 626–631 CrossRef CAS.
- J. Vas and M. Monestier, Immunology of Mercury, Ann. N. Y. Acad. Sci., 2008, 1143(1), 240–267 CrossRef CAS.
- A. Masih, A. Taneja and R. Singhvi, Exposure profiles of mercury in human hair at a Terai belt of North India, Environ. Geochem. Health, 2016, 38(1), 145–156 CrossRef CAS.
- C. Song, B. Yang, Y. Zhu, Y. Yang and L. Wang, Ultrasensitive sliver nanorods array SERS sensor for mercury ions, Biosens. Bioelectron., 2017, 87, 59–65 CrossRef CAS.
- W. Crowe, P. J. Allsopp, G. E. Watson, P. J. Magee, J. J. Strain and D. J. Armstrong, et al., Mercury as an environmental stimulus in the development of autoimmunity – a systematic review, Autoimmun. Rev., 2017, 16(1), 72–80 CrossRef CAS.
- E. M. Nolan and S. J. Lippard, Tools and Tactics for the Optical Detection of Mercuric Ion, Chem. Rev., 2008, 108(9), 3443–3480 CrossRef CAS.
- D. W. Boening, Ecological effects, transport, and fate of mercury: a general review, Chemosphere, 2000, 40(12), 1335–1351 CrossRef CAS.
- C. Wu, J. Wang, J. Shen, C. Bi and H. Zhou, Coumarin-based Hg2+ fluorescent probe: synthesis and turn-on fluorescence detection in neat aqueous solution, Sens. Actuators, B, 2017, 243, 678–683 CrossRef CAS.
- J. V. Cizdziel and S. Gerstenberger, Determination of total mercury in human hair and animal fur by combustion atomic absorption spectrometry, Talanta, 2004, 64(4), 918–921 CrossRef CAS.
- A. Q. Shah, T. G. Kazi, J. A. Baig, H. I. Afridi and M. B. Arain, Simultaneously determination of methyl and inorganic mercury in fish species by cold vapour generation atomic absorption spectrometry, Food Chem., 2012, 134(4), 2345–2349 CrossRef CAS.
- Y. Zhao, J. Zheng, L. Fang, Q. Lin, Y. Wu and Z. Xue, et al., Speciation analysis of mercury in natural water and fish samples by using capillary electrophoresis-inductively coupled plasma mass spectrometry, Talanta, 2012, 89, 280–285 CrossRef CAS PubMed.
- O. Abollino, A. Giacomino, M. Malandrino, G. Piscionieri and E. Mentasti, Determination of Mercury by Anodic Stripping Voltammetry with a Gold Nanoparticle-Modified Glassy Carbon Electrode, Electroanalysis, 2008, 20(1), 75–83 CrossRef CAS.
- K. Ponnuvel, V. Padmini and R. Sribalan, A new tetrazole based turn-on fluorescence chemosensor for Zn2+ ions and its application in bioimaging, Sens. Actuators, B, 2016, 222, 605–611 CrossRef CAS.
- K. Ponnuvel, M. Kumar and V. Padmini, A new quinoline-based chemosensor for Zn2+ ions and their application in living cell imaging, Sens. Actuators, B, 2016, 227, 242–247 CrossRef CAS.
- B. Zhu, Z. Wang, Z. Zhao, W. Shu, M. Zhang and L. Wu, et al., A simple highly selective and sensitive hydroquinone-based two-photon fluorescent probe for imaging peroxynitrite in live cells, Sens. Actuators, B, 2018, 262, 380–385 CrossRef CAS.
- L. Wang, J. Zhang, X. An and H. Duan, Recent progress on the organic and metal complex-based fluorescent probes for monitoring nitric oxide in living biological systems, Org. Biomol. Chem., 2020, 18(8), 1522–1549 RSC.
- H. N. Kim, W. X. Ren, J. S. Kim and J. Yoon, Fluorescent and colorimetric sensors for detection of lead, cadmium, and mercury ions, Chem. Soc. Rev., 2012, 41(8), 3210–3244 RSC.
- J. F. Zhang, Y. Zhou, J. Yoon and J. S. Kim, Recent progress in fluorescent and colorimetric chemosensors for detection of precious metal ions (silver, gold and platinum ions), Chem. Soc. Rev., 2011, 40(7), 3416–3429 RSC.
- S.-L. Kao and S.-P. Wu, A fluorescent turn-on probe for Hg(II) based on an NTe2 chelating motif and its application in living cell imaging, Sens. Actuators, B, 2015, 212, 382–388 CrossRef CAS.
- S. Erdemir, O. Kocyigit and S. Karakurt, A new perylene bisimide-armed calix[4]-aza-crown as “turn on” fluorescent sensor for Hg2+ ion and its application to living cells, Sens. Actuators, B, 2015, 220, 381–388 CrossRef CAS.
- S.-Y. Yu and S.-P. Wu, A highly selective turn-on fluorescence chemosensor for Hg(II) and its application in living cell imaging, Sens. Actuators, B, 2014, 201, 25–30 CrossRef CAS.
- K. M. Vengaian, C. D. Britto, K. Sekar, G. Sivaraman and S. Singaravadivel, Fluorescence “on–off–on” chemosensor for selective detection of Hg2+ and S2−: application to bioimaging in living cells, RSC Adv., 2016, 6(9), 7668–7673 RSC.
- A. K. Jha, S. Umar, R. K. Arya, D. Datta and A. Goel, Pyrano[3,2-c]julolidin-2-ones: a novel class of fluorescent probes for ratiometric detection and imaging of Hg2+ in live cancer cells, J. Mater. Chem. B, 2016, 4(28), 4934–4940 RSC.
- K. M. Vengaian, C. D. Britto, G. Sivaraman, K. Sekar and S. Singaravadivel, Phenothiazine based sensor for naked-eye detection and bioimaging of Hg(II) and F− ions, RSC Adv., 2015, 5(115), 94903–94908 RSC.
- J. Ding, H. Li, C. Wang, J. Yang, Y. Xie and Q. Peng, et al., “Turn-On” Fluorescent Probe for Mercury(II): High Selectivity and Sensitivity and New Design Approach by the Adjustment of the π-Bridge, ACS Appl. Mater. Interfaces, 2015, 7(21), 11369–11376 CrossRef CAS PubMed.
- Y. Yan, Y. Zhang and H. Xu, A Selective “Turn-On” Fluorescent Probe for Recognition of Mercury(II) Ions in Aqueous Solution Based on a Desulfurization Reaction, ChemPlusChem, 2013, 78(7), 628–631 CrossRef CAS.
- M. Santra, B. Roy and K. H. Ahn, A “Reactive” Ratiometric Fluorescent Probe for Mercury Species, Org. Lett., 2011, 13(13), 3422–3425 CrossRef CAS PubMed.
- F. Borges, F. Roleira, N. Milhazes, L. Santana and E. Uriarte, Simple Coumarins and Analogues in Medicinal Chemistry: Occurrence, Synthesis and Biological Activity, Curr. Med. Chem., 2005, 12(8), 887–916 CrossRef CAS PubMed.
- X.-y. Sun, T. Liu, J. Sun and X.-j. Wang, Synthesis and application of coumarin fluorescence probes, RSC Adv., 2020, 10(18), 10826–10847 RSC.
- M. Xing, K. Wang, X. Wu, S. Ma, D. Cao and R. Guan, et al., A coumarin chalcone ratiometric fluorescent probe for hydrazine based on deprotection, addition and subsequent cyclization mechanism, Chem. Commun., 2019, 55(99), 14980–14983 RSC.
- M. E. Aliaga, M. Gazitua, A. Rojas-Bolanos, M. Fuentes-Estrada, D. Durango and O. Garcia-Beltran, A selective thioxothiazolidin-coumarin probe for Hg2+ based on its desulfurization reaction. Exploring its potential for live cell imaging, Spectrochim. Acta, Part A, 2020, 224, 7 CrossRef PubMed.
- C. G. Chen, N. Vijay, N. Thirumalaivasan, S. Velmathi and S. P. Wu, Coumarin-based Hg2+ fluorescent probe: fluorescence turn-on detection for Hg2+ bioimaging in living cells and zebrafish, Spectrochim. Acta, Part A, 2019, 219, 135–140 CrossRef CAS PubMed.
- X. H. Cheng, S. H. Qu, L. Xiao, W. N. Li and P. He, Thioacetalized coumarin-based fluorescent probe for mercury(II): ratiometric response, high selectivity and successful bioimaging application, J. Photochem. Photobiol., A, 2018, 364, 503–509 CrossRef CAS.
- Y. Ding, Y. M. Pan and Y. F. Han, A Coumarin-Based Fluorescent Probe for Ratiometric Monitoring of Hg2+ in Live Cells, Ind. Eng. Chem. Res., 2019, 58(19), 7786–7793 CrossRef CAS.
- M. Dong, J. Y. Tang, Y. F. Lv, Y. T. Liu, J. F. Wang and T. T. Wang, et al., A dual-function fluorescent probe for Hg(II) and Cu(II) ions with two mutually independent sensing pathways and its logic gate behavior, Spectrochim. Acta, Part A, 2020, 226, 7 Search PubMed.
- X. W. Li, Q. X. Duan, Y. M. Yu, K. Wang, H. C. Zhu and X. Zhang, et al., A coumarin-based fluorescent probe for Hg2+ and its application in living cells and zebrafish, Luminescence, 2020, 35(6), 941–946 CrossRef CAS PubMed.
- S. Mondal, N. Patra, H. P. Nayek, S. K. Hira, S. Chatterjee and S. Dey, Unusual absence of FRET in triazole bridged coumarin-hydroxyquinoline, an active sensor for Hg2+ detection, Photochem. Photobiol. Sci., 2020, 19(9), 1211–1221 CrossRef CAS PubMed.
- J. M. V. Ngororabanga, Z. R. Tshentu and N. Mama, A New Highly Selective Colorimetric and Fluorometric Coumarin-based Chemosensor for Hg2+, J. Fluoresc., 2020, 30(5), 985–997 CrossRef CAS PubMed.
- Z. X. Pan, Z. X. Xu, J. Chen, L. P. Hu, H. Q. Li and X. Zhang, et al., Coumarin Thiourea-Based Fluorescent Turn-on Hg2+ Probe That Can Be Utilized in a Broad pH Range 1–11, J. Fluoresc., 2020, 30(3), 505–514 CrossRef CAS PubMed.
- Y. M. Yu, C. Y. Liu, B. Tian, X. Y. Cai, H. C. Zhu and P. Jia, et al., A novel highly selective ratiometric fluorescent probe with large emission shift for detecting mercury ions in living cells and zebrafish, Dyes Pigm., 2020, 177, 7 CrossRef.
- H. E. Jiang, D. N. Tang, Z. J. Li, J. W. Li, H. B. Liu and Q. J. Meng, et al., A dual-channel chemosensor based on 8-hydroxyquinoline for fluorescent detection of Hg2+ and colorimetric recognition of Cu2+, Spectrochim. Acta, Part A, 2020, 243, 6 CrossRef PubMed.
- L. N. Liu, L. He, Y. Qu, N. Lu, Q. Y. Cao and Z. H. Yan, A hydroxyquinoline-base nanoprobe for fluorescent sensing of Hg2+ ion in aqueous solution, Inorg. Chim. Acta, 2018, 474, 128–133 CrossRef CAS.
- C. M. Lopez-Alled, L. C. Murfin, G. Kociok-Kohn, T. D. James, J. Wenk and S. E. Lewis, Colorimetric detection of Hg2+ with an azulene-containing chemodosimeter via dithioacetal hydrolysis, Analyst, 2020, 145(19), 6262–6269 RSC.
- J. Ding, H. Y. Li, C. Wang, J. Yang, Y. J. Xie and Q. Peng, et al., “Turn-On” Fluorescent Probe for Mercury(II): High Selectivity and Sensitivity and New Design Approach by the Adjustment of the pi-Bridge, ACS Appl. Mater. Interfaces, 2015, 7(21), 11369–11376 CrossRef CAS PubMed.
- Y. Y. Gao, T. T. Ma, Z. Z. Ou, W. J. Cai, G. Q. Yang and Y. Li, et al., Highly sensitive and selective turn-on fluorescent chemosensors for Hg2+ based on thioacetal modified pyrene, Talanta, 2018, 178, 663–669 CrossRef CAS PubMed.
- M. S. Xu, L. Wang, M. Li, Z. W. Ma, D. Zhang and J. H. Liu, A new highly sensitive and selective fluorescent probe for Hg2+ and its application in living cells, Phosphorus, Sulfur Silicon Relat. Elem., 2020, 196(1), 13–18 CrossRef.
Footnote |
† Electronic supplementary information (ESI) available. See DOI: 10.1039/d1ra01408k |
|
This journal is © The Royal Society of Chemistry 2021 |