DOI:
10.1039/D1RA01179K
(Paper)
RSC Adv., 2021,
11, 11244-11254
Incorporation of Keggin-based H3PW7Mo5O40 into bentonite: synthesis, characterization and catalytic applications†
Received
12th February 2021
, Accepted 26th February 2021
First published on
17th March 2021
Abstract
The Keggin-based molybdo-substituted tungstophosphoric acid, H3[PW7Mo5O40]·12H2O, were synthesized and incorporated with a bentonite clay by using a wetness impregnation method. The catalysts were characterized using several methods, such as inductively coupled plasma-atomic emission spectroscopy (ICP-AES), Fourier transform infrared spectroscopy (FT-IR), X-ray diffractometry (XRD), scanning electron microscopy energy-dispersive X-ray spectroscopy (SEM-EDS), transmission electron microscopy (TEM), and thermogravimetric and differential thermal analysis (TG-DTA). This extremely active catalytic system provides a green strategy for the synthesis of 1,8-dioxo-octahydroxanthene and 1,8-dioxo-decahydroacridine derivatives under solvent free conditions at 80 °C with a good reaction mass efficiency, effective mass yield, and excellent atom economy. Both the surface acidity and catalytic activity sharply increased after H3[PW7Mo5O40]·12H2O was impregnated with bentonite clay. In addition, the PW7Mo5/bentonite catalyst can be conveniently recovered and reused numerous times without demonstrating a significant loss in activity.
1. Introduction
Polyoxometallates (POMs) or heteropoly acid (HPAs) are a large family of distinct d-block transitional metal-oxide, anionic cluster structures of metals, they have inspired several recent research activities in comprehensive fields of science.1–6 POMs can, in some instances, be assembled with precise control regarding the placement of different heteroatoms such as W, Mo, or V within their molecular frameworks.7–9 Predominantly, the Keggin-type POMs are also characteristically templated by a central tetrahedral template {XO4} (X = P, Si, Nb, B, Ge, etc.) that can have a prominent impact on the properties of the anion, these are mostly associated with a change in the charge and polarization of the metal-oxo shell.10–15 Furthermore, one or more of the W atoms can be substituted by different heteroatoms that comprise inner transition metals, such as Co, Ni, Mo, V and so on to yield transition-metal substituted POMs.8,15–17 This compositional modification tunes the reactivity and effects processes such as the acidity and stability of the POMs.18,19 However, the major problems with POMs are their low thermal stability, low surface area, high solubility in polar solvents, and their recovery and reusability.20 For the effective access the catalyst needs to be supported on a suitable support material.21–23
Bentonite, a clay mineral which is abundant on earth, consists of layers of two tetrahedral silica sheets sandwiching one octahedral alumina sheet.24,25 It is well-documented that bentonite clays can be used as an efficient support owing to their common fascinating features, such as their higher surface acidity, excellent thermal stability, and effortlessly controlled structure and morphology.26
Multicomponent reactions (MCRs) provide a convenient approach for the rapid synthesis of complex molecules from simple starting materials, without the isolation of intermediates.27 The MCRs have achieved significant importance in medicinal and organic chemistry,28 because these reactions minimize the consumption of catalysts and solvents, thus permitting the minimization of waste, time, effort and cost as compared to multistep synthesis.29,30 Xanthene derivatives have been classified as oxygen-containing molecular families, possessing a highly reactive inbuilt pyran ring.31 In addition, they have been used as synthetic precursors for several organic compounds and dyes,32–34 such as fluorescents and chiroptical molecular switches (examples include, rosamine, fluorone, fluorescein, rhodamine etc.).33,35 The core structure of the xanthene derivatives shows a multitude of physicochemical and pharmacological properties, such as anti-inflammatory,36 antimalarial,37 analgesic,38 anticancer,20 antiviral,39 antibacterial properties, and so on.38 Additionally, acridine derivatives, constituting a 1,4-dihydropyridine (DHP) ring skeleton, are very important compounds owing to their pharmacological properties.40 Various members of this family are currently used for cytostatic and antitumor activities (for example, nitracrine derivatives),41 platelet antiaggregatory activities, the treatment of Alzheimer's disease, strong anti-cancer treatments (for example, amsacrine derivatives), cardiovascular diseases including hypertension and diabetes, and so on.41,42 The structures of some xanthene and acridine derivatives are shown in Fig. 1.
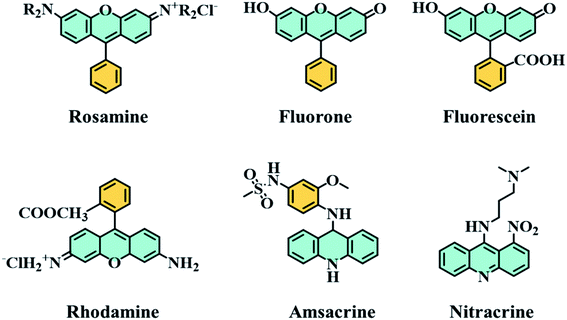 |
| Fig. 1 Structures of some known xanthenes and acridines. | |
As yet, several procedures have been employed for synthesizing many of these organic derivatives using various catalysts, such as Fe3+ montmorillonite,43 NiFeTi CLDH6,42 SmCl3,44 HClO4–SiO2,45 InCl3/ionic liquid,46,47 FSG-Hf(NPf2)4,48 p-dodecylbenzenesulfonic acid, Amberlyst-15,49 [MIMPS]3PW12O40 and [TEAPS]3PW12O40,47 SO42−/ZrO2,50 [Hbim]BF4,51 [Cmmim][BF4],52 SBSSA,53 [Hmim]TFA,54 CaCl2,55 SBNPSA,56 thiourea dioxide,57 SbCl3/SiO2,58 and 2,4,6-trichloro-1,3,5-triazine (TCT).59 However, these types of catalyst possesses some disadvantages, such as an inability to recycle the catalyst, the use of expensive reagents, use of reflux conditions, prolonged work up, hazardous organic solvents and low reaction yields. Therefore, it has become important to find an alternative route for the synthesis of xanthene and acridine derivatives. Hence, the development of a novel, contemporary reaction that is easy to perform, cost effective, and offers the reusability of the catalyst, and perhaps offers a greener method, is highly attractive.
In this regard, and in a continuation of our interest in the development of modified novel Keggin-type POMs for different acid catalysed organic transformations,60,61 herein, we report a series of molybdo-substituted tungstophosphoric acid H3[PW7Mo5O40]·12H2O catalysts that were prepared and impregnated with bentonite clay (Scheme 1). Their effectiveness was explored for the preparation of 1,8-dioxo-octahydroxanthene and 1,8-dioxo-decahydroacridine derivatives in excellent yields and with a short reaction time.
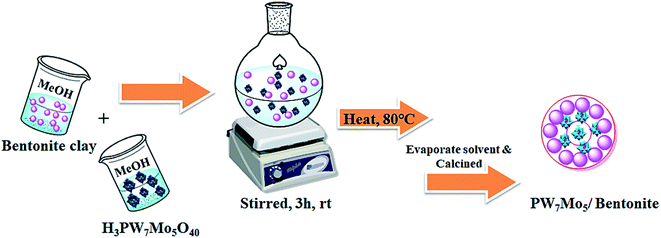 |
| Scheme 1 Schematic illustration showing the synthetic procedure for the fabrication of the PW7Mo5/bentonite catalyst. | |
2. Results and discussion
2.1 Catalytic characterization
2.1.1 FT-IR analysis.
The Fourier transform infrared spectroscopy (FT-IR) spectra of the bulk PW7Mo5, bentonite clay and PW7Mo5/bentonite composites with PW7Mo5 loading from 10–25% are depicted in Fig. 2. For the bulk PW7Mo5, the characteristic bands exhibited at 1070 (P–Oa in central tetrahedral), 958 (terminal M
Od), 873 (M–Ob–M), and 762 cm−1 (M–Oc–M) coincide with the asymmetric vibrations in the Keggin unit.17 The strong characteristic bands of the Si–O stretching vibrations were detected at 993 cm−1 and the bending vibration of Al–Al–OH was determined at 913 cm−1 for the parent bentonite. Additionally, the other two bands at 790 and 691 cm−1 correspond to the Si–O–Al and Si–O–Si bending vibrations, respectively.25 However, the characteristics peaks of the 10%, 15%, 20% and 25% PW7Mo5 supported bentonite clays have a similar pattern to that of the parent bentonite, as only small shifts in frequency appeared owing to the electrostatic interactions between the Keggin structure of PW7Mo5 and the parent bentonite.22,62
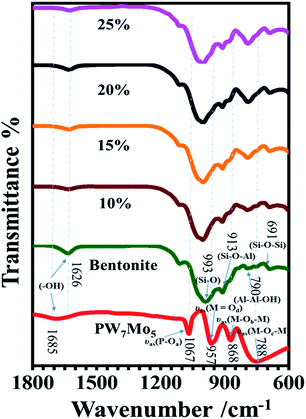 |
| Fig. 2 FT-IR analysis of bulk PW7Mo5, bentonite and the PW7Mo5/bentonite catalyst. | |
2.1.2 XRD analysis.
The X-ray diffractometry (XRD) analysis of the bulk PW7Mo5, plain bentonite and PW7Mo5/bentonite composites (with PW7Mo5 loading from 10, 15, 20 and 25%) in 3° < 2θ < 80° are depicted in Fig. 3. The bulk PW7Mo5 catalyst exhibited major diffraction peaks, for example 2θ, at 7–10°, 14.5°, 20.7°, 25.44° and 34.4°, demonstrating the characteristic features of the Keggin structure and the crystalline state.17 Sharp reflections exhibited at 2θ = 7° and 20° are characteristic of the amorphous material of plain bentonite, and the one at 2θ = 27° corresponds to α-quartz. For the XRD patterns of the different loading values (10, 15, 20 and 25%) of PW7Mo5 on bentonite, the peaks were observed to be less intense, demonstrating that during the impregnation of PW7Mo5 on bentonite, the clay lost some of its crystallinity compared to the parent bentonite. Most of the diffractograms of PW7Mo5/bentonite indicate that no separate crystal phases were detected and the 2θ value of quartz was unaffected, even after exchanging the bentonite clay with PW7Mo5.26,63 This indicates that PW7Mo5 was well distributed over the surface of bentonite.
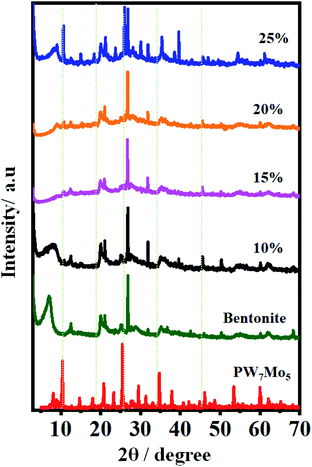 |
| Fig. 3 XRD analysis of bulk PW7Mo5, bentonite and the PW7Mo5/bentonite catalyst. | |
2.1.3 SEM and TEM analysis.
The surface morphology and texture of the prepared samples were examined using scanning electron microscopy (SEM) and transmission electron microscopy (TEM) analysis (Fig. 4a–d). Fig. 4a shows the agglomerated irregular-shaped crystalline structure with smooth particles for bulk PW7Mo5 at 2 µm magnification. The SEM images of bentonite and 20% PW7Mo5/bentonite are depicted in Fig. 4b and c respectively. The result clearly reveals that the surface morphology of the 20% PW7Mo5/bentonite catalyst is quite dissimilar from that of the parent bentonite clay. Subsequently, it was confirmed that PMo7W5 are homogeneously dispersed over the surface of bentonite.
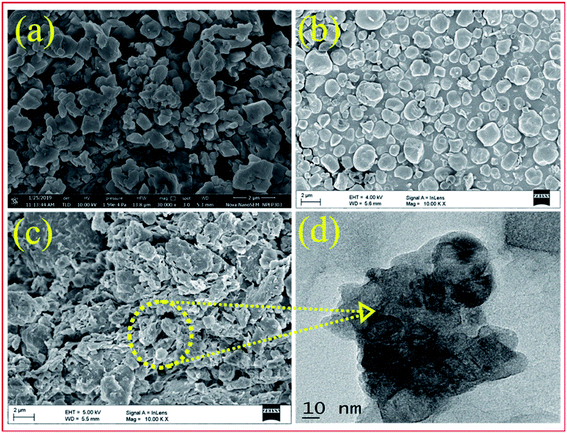 |
| Fig. 4 FE-SEM images of bulk PW7Mo5 (a), bentonite clay (b), 20% PW7Mo5/bentonite (c) and the TEM images of 20% PW7Mo5/bentonite (d). | |
In addition, the morphology of the 20% PW7Mo5/bentonite samples was investigated using TEM. The smaller cloudy-like features and dark coloured spot in Fig. 4 indicate that the functional components of PW7Mo5 were successfully immobilized and aggregate with the bentonite clay support.
2.1.4 EDX analysis and elemental mapping images.
The chemical compositions of the bulk PW7Mo5 and 20% PW7Mo5/bentonite were confirmed using energy-dispersive X-ray spectroscopy (EDS) analysis as shown in Fig. S1.† The results obtained for the fresh PW7Mo5 catalyst clearly show the occurrence of P, W and Mo (Fig. S1a†). Furthermore, the EDX analysis of 20% PW7Mo5/bentonite (Fig. S1b†) showed the presence of P, W, Mo, Na, Ca, Al and O elements, confirming the formation of 20% PW7Mo5/bentonite.
Additionally, the elemental mapping images (EDS) of the catalyst proved the uniform distribution of the elements such as W, Mo, P, Na, Ca, Al and O in the prepared bulk PW7Mo5 and the 20% PW7Mo5/bentonite catalyst (Fig. S2a and b†).
2.1.5 TG-DT analysis.
The thermal stability of bulk PW7Mo5 and 20% PW7Mo5/bentonite were studied using thermogravimetric and differential thermal analysis (TG-DT) (Fig. 5). The three types of crystallographic water molecule obtained in the POMs are hydration water, protonized water and structural water, as depicted in Fig. 7.17,60 The TG curve for the bulk PW7Mo5 shows the total weight loss of 8.78% below 520 °C, which demonstrates that 12-water molecules were lost. The first mass loss at 70 to 150 °C, was about 7.38% because of the loss of the absorbed water molecule. The second weight loss of 2.92% appeared as an endothermic peak at 350 °C for 2.18 molecules of protonized water. The third exothermic peak appeared at 520 °C, owing to the weight loss of 1.2% for the structural water molecule. The exothermic peak centred at 520 °C is attributed to the decay of the Keggin unit of PW7Mo5 to the individual oxides, suggesting that the POMs will persist up to 520 °C (Fig. 5a).
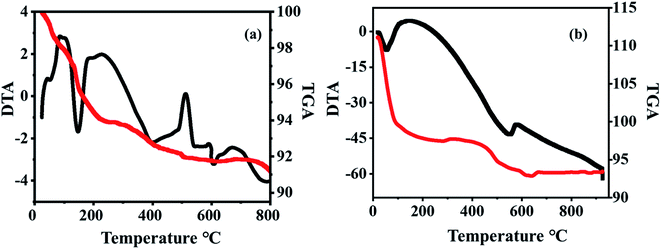 |
| Fig. 5 TG-DT analysis of (a) PW7Mo5 and (b) the 20% PW7Mo5/bentonite catalyst. | |
The thermogravimetric analysis (TGA) of 20% PW7Mo5/bentonite shows a weight loss at 80 to 175 °C, of about 9.8% owing to the weight loss of the adsorbed water molecules. A gradual mass loss of about 3% is observed up to 630 °C, which is due to the release of structural water. From this it can be seen that the thermal stability of the bulk PW7Mo5 materials has noticeably improved, which may be due to the strong interaction between PW7Mo5 and bentonite clay (Fig. 5b).14
2.2 Catalytic activity test
The catalytic activity of the newly prepared PW7Mo5/bentonite was explored for the synthesis of substituted xanthenes. Moreover, to accomplish the best reaction conditions for the xanthene synthesis, the effect of PW7Mo5/bentonite loading, the amount of PW7Mo5/bentonite, the results in various solvents, different temperatures and comparison of different catalysts were obtained. A model reaction between benzaldehyde and 5,5-dimethyl-1,3-cyclohexanedione was studied to optimize these parameters.
For a demonstration of the effect of the PW7Mo5 catalyst loading on bentonite for the synthesis of 3a, different catalyst loadings were employed, such as the parent bentonite, bulk PW7Mo5, 10%, 15%, 20%, and 25% w/w, as shown in Table 1. The parent bentonite gave a moderate yield of the product, but it took longer for the reaction to reach completion (Table 1, entry 1). Bulk PW7Mo5 gave a reasonable catalytic ability in terms of the reaction time and the yield of the desired product (Table 1, entry 2). It was observed that both the yield of the product and the reaction time improved upon increasing the catalyst loading up to 20% w/w (Table 1, entries 3–5). Additionally, an increase in the amount of catalyst does not affect the reaction time and the yield of the product as a result of the leaching of the catalyst from the support (Table 1, entry 6).
Table 1 Effect of PW7Mo5 loading on the support bentonite for the model reactiona
Entry |
Catalyst |
Timeb (min) |
Yieldc (%) |
Reaction conditions: 1 : 2 ratio of benzaldehyde, 5,5-dimethyl-1,3-cyclohexanedione and different catalysts (100 mg) at 80 °C.
Reaction progress monitored by TLC.
Isolated yields, bold values highlight the best result.
|
1 |
Pure bentonite |
85 |
65 |
2 |
Bulk H3PW7Mo5O40·12H2O |
45 |
70 |
3 |
10% PW7Mo5/bentonite |
35 |
70 |
4 |
15% PW7Mo5/bentonite |
15 |
85 |
5
|
20% PW
7
Mo
5
/bentonite
|
5
|
92
|
6 |
25% PW7Mo5/bentonite |
5 |
92 |
Next, we performed the model reaction using various amounts of 20% PW7Mo5/bentonite to assess the effect of the catalyst on the synthesis of xanthene derivatives at 80 °C. It was observed that in the absence of a catalyst, product 3a was only afforded in trace amounts (Table 2, entry 1), even after 3 h. By increasing the amount of the catalyst up to 100 mg, a notable alteration was observed in the percentage yield (Table 2, entries 2–4). There is no significant improvement in the yield observed upon increasing the amount of 20% PW7Mo5/bentonite catalyst up to 120 mg, because the additional catalyst does not increase the rate of the reaction (Table 2, entry 9).
Table 2 Optimization of the reaction conditions for the model reactiona
Entry |
Catalyst (mg) |
Temperature (°C) |
Timeb (min) |
Yieldc (%) |
Reaction conditions: 1 : 2 ratio of benzaldehyde, 5,5-dimethyl-1,3-cyclohexanedione and 20% PW7Mo5/bentonite.
Reaction progress monitored by TLC.
Isolated yields, bold values represent the best result.
|
1 |
— |
80 |
180 |
Trace |
2 |
20 |
80 |
120 |
55 |
3 |
50 |
80 |
75 |
60 |
4 |
80 |
80 |
65 |
75 |
5
|
100
|
80
|
5
|
92
|
6 |
100 |
50 |
55 |
80 |
7 |
100 |
70 |
50 |
83 |
8 |
100 |
100 |
5 |
92 |
9 |
120 |
80 |
10 |
92 |
Hereafter, the effects of the temperature and time were studied, which play an important role and affect the reaction kinetics to a large extent. Various temperatures (50–100 °C) were used to carry out the model reaction for different time periods (5–180 min). Hence, the best result was obtained in the presence of 100 mg of 20% PW7Mo5/bentonite at 80 °C (Table 2, entry 5). It was observed that no substantial change was detected after extending the reaction time and increasing the temperature.
To study the advantages of the solvent-free reaction conditions over various organic solvents, the model reaction was performed in dissimilar solvents and the results are represented in Table 3. If MeOH, EtOH, EtOH–H2O (1
:
1), and polyethylene glycol (PEG-400) are used as the solvents, moderate yields of the products are obtained (Table 3, entries 1–4), whereas, if CH3CN and PhMe are used, a poor yield of the product is obtained (Table 3, entries 5 and 6). However, when the reaction was carried out under thermal solvent-free conditions, a superior yield of the product was obtained within a short reaction time.
Table 3 Effect of different solvents on the model reactiona
Entry |
Solvent |
Temperature (°C) |
Timeb (min) |
Yieldc (%) |
Reaction conditions: 1 : 2 ratio of benzaldehyde, 5,5-dimethyl-1,3-cyclohexanedione and 20% PW7Mo5/bentonite (100 mg).
Reaction progress was monitored by TLC.
Isolated yields, bold values highlight the best result.
|
1 |
MeOH |
Reflux |
25 |
55 |
2 |
EtOH |
Reflux |
20 |
65 |
3 |
EtOH–H2O (1 : 1) |
Reflux |
10 |
68 |
4 |
PEG-400 |
110 |
35 |
75 |
5 |
CH3CN |
Reflux |
5 |
45 |
6 |
PhMe |
Reflux |
25 |
35 |
7
|
Solvent free
|
80
|
5
|
92
|
To further extend the reaction scope of the catalyst, the synthesis of 1,8-dioxo-octahydroacridines (Table 4) was carried out under the same reaction conditions as used for the synthesis of the corresponding 1,8-dioxo-octahydroxanthenes. We carried out the reaction in a 1
:
2
:
1 ratio of aldehydes, 5,5-dimethyl-1,3-cyclohexanedione and ammonium acetate (NH4OAc) with 20% PW7Mo5/bentonite at 80 °C, which afforded the 1,8-dioxo-decahydroacridine derivatives (4a–4n) in excellent yields (92–79%) within a short period of time (Table 5). In addition, the aromatic aldehydes containing both electron withdrawing and electron donating groups afforded the products with high yields. The work-up procedure was very simple and included the addition of hot ethanol at the end of the reaction, filtering the reaction mixture to separate off the catalyst, and finally recrystallizing the products from ethanol.
Table 4 Synthesis of 1,8-dioxo-octahydroxanthenes using the 20% PW7Mo5/bentonite catalysta,b,c
Table 5 Synthesis of 1,8-dioxo-decahydroacridines using the 20% PW7Mo5/bentonite catalysta,b,c
Quantification of the effectiveness of a reaction with the aim of waste reduction continues to drive the development of novel green chemistry metrics, such as the E-factor, atom economy, reaction mass efficiency, optimum efficiency, and practical mass yield.67,68 These metrics serve to quantify the efficiency or environmental performance of a chemical reaction.69
The E-factor is the mass ratio of waste to desired product and the atom efficiency.70 The lower the value of the E-factor, the more ecocompatible the reaction. An E-factor ranging from 0.1 to 0.6 highlights the greenness of the protocol, as shown in Fig. 6.
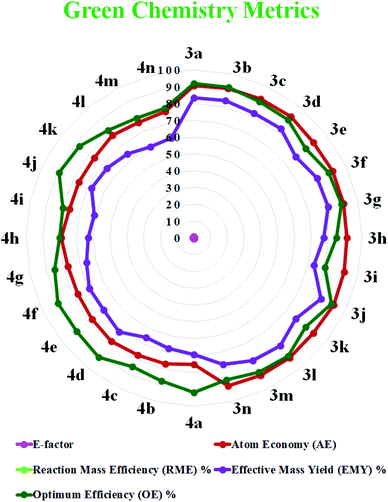 |
| Fig. 6 Radar chart of the measured green metrics for the synthesis of 1,8-dioxo-octahydroxanthene (3a–n) and the 1,8-dioxo-decahydroacridine derivatives (4a–n). | |
We measured the green chemistry metrics for both the 1,8-dioxo-octahydroxanthenes and 1,8-dioxo-octahydroacridines derivatives to afford 3a and 4a under the optimized reaction conditions and compared these with their ideal value, as demonstrated in Table 6. The result shows that the values of the green chemistry metrics such as the E-factor, atom economy (AE), reaction mass efficiency (RME), effective mass yield (EMY) and optimum efficiency (OE) are close to their ideal values, as demonstrated here (see the ESI† for detailed calculations).
Table 6 Quantitative evaluation of green chemistry metrics for 3a and 4a
Entry |
Green chemistry metrics |
Ideal value |
Product 3a |
Product 4a |
1 |
E-Factor |
0 |
0.19 |
0.44 |
2 |
Atom economy (AE) |
100% |
90.67 |
75.38 |
3 |
Reaction mass efficiency (RME) |
100% |
83.39 |
69.34 |
4 |
Effective mass yield (EMY) |
100% |
83.39 |
69.34 |
5 |
Optimum efficiency (OE) |
100% |
91.97 |
91.98 |
A comparison of the catalytic efficiency of the 20% PW7Mo5/bentonite catalyst was performed with other reported catalysts for the synthesis of the 1,8-dioxo-octahydroxanthene derivatives. The result shows that 20% PW7Mo5/bentonite is superior to some of the previous reported catalysts in terms of the excellent yield and short reaction time (Table 7, entry 12).
Table 7 Comparative study of 20% PW7Mo5/bentonite with previously reported catalysts for the model reaction
Entry |
Catalyst |
Reaction conditions |
Time |
Yield (%) Ref. |
1 |
Fe3+ montmorillonite |
EtOH, 100 °C |
6 h |
94 (ref. 43) |
2 |
SO42−/ZrO2 |
EtOH, 70 °C |
8 h |
95 (ref. 50) |
3 |
[Hbim]BF4 |
MeOH, rt |
45 min |
85 (ref. 51) |
4 |
[Cmmim][BF4] |
Solvent free, 80 °C |
150 min |
87 (ref. 52) |
5 |
SBSSA |
Reflux, EtOH |
10 h |
98 (ref. 53) |
6 |
[Hmim]TFA |
Solvent free, 80 °C |
3 h |
85 (ref. 54) |
7 |
CaCl2 |
DMSO/85–90 °C |
4 h |
85 (ref. 55) |
8 |
SBNPSA |
Reflux, EtOH |
2 h |
93 (ref. 56) |
9 |
Thiourea dioxide |
Reflux |
45 min |
96 (ref. 57) |
10 |
SbCl3/SiO2 |
Solvent-free, 120 °C |
50 min |
93 (ref. 58) |
11 |
2,4,6-Trichloro-1,3,5-triazine (TCT) |
Solvent-free, 120 °C |
50 min |
92 (ref. 59) |
12 |
20% PW7Mo5/bentonite |
Solvent free, 80 °C |
5 min |
92 (This work) |
2.3 Plausible mechanism for the reaction
The 20% PW7Mo5/bentonite catalyses the synthesis of the 1,8-dioxo-octahydroxanthene derivatives by stimulating the carbonyl group of the aromatic aldehydes (1), making it more liable to nucleophilic attack by dimedone (2), to form intermediate (a), followed by Michael addition of another molecule of dimedone to form the intermediate (b). Intramolecular cyclization occurs after the successive removal of water, which results in the desired product (3a–3n) and regenerates the 20% PW7Mo5/bentonite catalyst in the reaction mixture (Fig. 7).
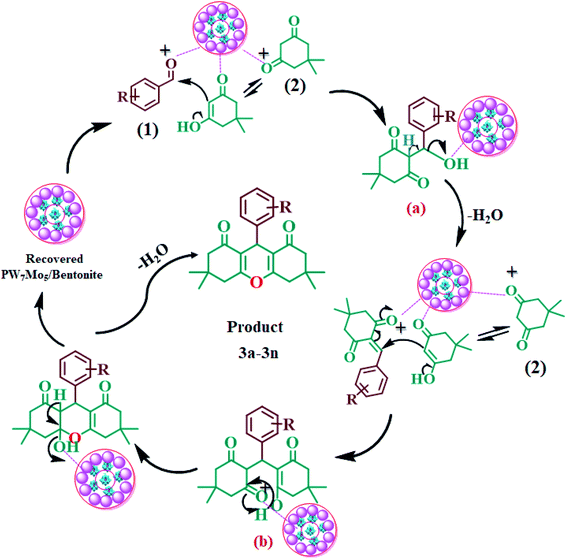 |
| Fig. 7 Probable mechanism for the synthesis of 1,8-dioxo-octahydroxanthenes using the 20% PW7Mo5/bentonite catalyst. | |
2.4 Recyclability of the 20% PW7Mo5/bentonite catalyst
To study the reusability of the catalyst (20% PW7Mo5/bentonite), recycling studies were performed using a 1
:
2 ratio of benzaldehyde, 5,5-dimethyl-1,3-cyclohexanedione and 20% PW7Mo5/bentonite catalyst (100 mg), which were observed to be the optimized reaction conditions for a specified time period. After the reaction reached completion (monitored by thin layer chromatography (TLC)), the reaction mixture was diluted using hot ethanol (10.0 mL) and filtered to separate the catalyst. The crude product was obtained by solvent evaporation under reduced pressure and recrystallized from ethanol. The recovered catalyst was washed with ethanol (10 mL) and dried overnight for further reuse. The results (Table 8) reveal that the catalyst stability was maintained for up to six cycles. After the sixth cycle, the recovered catalyst was characterized using FT-IR and powder XRD analysis. The characteristic peaks at 1623, 1005, 913, 787 and 687 cm−1 for the FT-IR spectra (Fig. S3a†) and 2θ = 20.70°, 24.88°, 26.89°, and 31.63° for the XRD pattern (Fig. S3b†) remained the same.
Table 8 Recycling study of the catalyst for the model reactiona
No. of runs |
Timeb (min) |
Yieldc (%) |
Reaction conditions: 1 : 2 ratio of benzaldehyde, 5,5-dimethyl-1,3-cyclohexanedione and 20% PW7Mo5/bentonite (100 mg).
Reaction progress monitored by TLC.
Isolated yield.
|
1 |
5 |
92 |
2 |
5 |
92 |
3 |
5 |
91 |
4 |
5 |
89 |
5 |
5 |
87 |
6 |
5 |
85 |
3. Experimental
3.1 Materials and general characterization
Sodium molybdate (Na2MoO4·2H2O), sodium tungstate (Na2WO4·2H2O), disodium phosphate (Na2HPO4), and bentonite clay powder were purchased from Molychem in India and used without further purification. All the chemicals and solvents used in the organic synthesis were purchased from Alfa Aesar, Merck and Sigma-Aldrich.
The elemental content was measured using inductively coupled plasma-atomic emission spectroscopy (ICP-AES), ARCOS, on a simultaneous ICP Spectrometer. The FT-IR spectra were obtained using a Bruker ALPHA (Eco-ATR) spectrophotometer. The XRD pattern was obtained using a Bruker AXS Company, D8 ADVANCE diffractometer (Germany). Scanning electron microscopy (SEM) images and elemental analysis of the catalyst were employed using a FEI Nova NanoSEM 450 combined with a Bruker XFlash 6I30 instrument for energy-dispersive X-ray spectroscopy (EDX), with a scanning electron electrode at 15 kV. TEM was performed on a HR-TEM: Jeol/JEM 2100 at 200 kV voltage. TG and DTA were performed on a SHIMADZU, DTG-60H simultaneous DTA-TG apparatus. TLC was used to monitor the reaction on Merck silica plates, and the imaging was accomplished using iodine/ultraviolet light. The melting points of all the synthesized compounds were determined in open capillary tubes and are uncorrected. The 1H NMR and 13C NMR spectra were recorded on a Bruker Avance DRX-400, in pure DMSO-d6 or CDCl3 solvents using TMS as an internal reference standard.
3.2 Catalyst preparation
Synthesis of the Keggin type POMs with the general formula H3[PW7Mo5O40]·12H2O was performed according to a previous report by Huixiong.8 Briefly, disodium phosphate (0.58 g, Na2HPO4) and the desired amount of sodium tungstate (9.46 g, Na2WO4·2H2O) were dissolved in deionised water (DI). The resulting mixture was stirred for 30 min at 90 °C. Then, an aqueous solution of sodium molybdate (4.95 g, Na2MoO4·2H2O) was added to the above heated solution, and the pH was maintained close to 1.5–2 using sulfuric acid (H2SO4). The mixture was heated at 90 °C for 6 h. Finally, the cooled solution was extracted with diethyl ether. The powder H3[PW7Mo5O40]·12H2O was obtained after the concentrated etherate solution was dried in a vacuum. The results of the ICP-AES elemental analysis revealed that the atomic ratio of P/W/Mo is almost maintained at 1.12/7.12/5.01 which corresponds to the formula H3[PW7Mo5O40]·12H2O (PW7Mo5).
In a typical synthesis of 10% PW7Mo5/bentonite, 0.5 g of PW7Mo5 was dissolved in dry methanol solution and added dropwise into the flask containing 4.5 g of bentonite clay and stirred for 3 h at room temperature. The resulting mixture was heated to 80 °C until complete evaporation of the liquid part had occurred. The solid residue was then calcined in an oven at 200 K for 5 h. A series of PW7Mo5/bentonite loadings (10, 15, 20, 25 wt%) were prepared using a similar method.
3.3 General procedure for synthesis of the 1,8-dioxo-octahydroxanthene derivatives
A mixture of aromatic aldehyde (3.0 mmol), 5,5-dimethylcyclohexane-1,3-dione (6.0 mmol), and 20% PW7Mo5/bentonite catalyst (100 mg) was heated at 80 °C under solvent-free conditions for a suitable time, as indicated by TLC. After the reaction was complete, the reaction mixture was diluted using hot ethanol (10.0 mL) and filtered for catalyst separation. The crude product was obtained by solvent evaporation under reduced pressure and recrystallized from ethanol. The recovered catalyst was washed with ethanol (10 mL) and dried overnight for further reuse. Recrystallized xanthene derivatives were characterized using FT-IR, 1H and 13C NMR spectroscopy. The NMR spectral data for product 3a, synthesized using the model reaction, are given below; the remaining spectroscopic data are provided in the ESI.†
3.3.1 3,3,6,6-Tetramethyl-9-phenyl-3,4,5,6,7,9-hexahydro-1H-xanthene-1,8(2H)-dione (3a).
IR (ATR, ν cm−1): 685, 835, 1035, 1242, 1364, 1581, 1664, 2958. 1H NMR (400 MHz, CDCl3) δH (ppm) = 1.09 (s, 6H, 2 × CH3), 1.23 (s, 6H, 2 × CH3), 2.37 (m, 8H, 4 × CH2), 5.54 (s, 1H, CH), 7.09 (d, J = 8.2 Hz, 2H, Ar-H), 7.22 (t, 1H, Ar-H), 7.26 (t, J = 8.4 Hz, 2H, Ar-H). 13C NMR (100 MHz, CDCl3) δC (ppm) = 27.5, 29.8, 31.5, 32.8, 46.5, 47.1, 115.7, 125.9, 126.8, 128.3, 138.1, 189.6, 190.6.
3.4 General procedure for synthesis of the 1,8-dioxo-decahydroacridine derivatives
A mixture of aromatic aldehyde (3.0 mmol), 5,5-dimethylcyclohexane-1,3-dione (6.0 mmol), ammonium acetate (NH4OAc, 3.0 mmol) and the 20% PW7Mo5/bentonite catalyst (100 mg) was heated at 80 °C under solvent-free conditions for a suitable time as indicated by TLC. After the reaction was complete, the reaction mixture was diluted using hot ethanol (10.0 mL) and filtered for catalyst separation. The crude product was obtained by solvent evaporation under reduced pressure and recrystallized from ethanol. The recovered catalyst was washed with ethanol (10 mL) and dried overnight for further reuse. The recrystallized xanthene derivatives were characterized using FT-IR, 1H and 13C NMR spectroscopy. The NMR spectral data for product 4a are given below; the remaining spectroscopic data are provided in the ESI.†
3.4.1 3,3,6,6-Tetramethyl-9-phenyl-3,4,6,7,9,10-hexahydroacridine-1,8(2H,5H)-dione (4a).
IR (ATR, ν cm−1): 661, 833, 1035, 1154, 1237, 1363, 1582, 1630, 2956, 3286. 1H NMR (400 MHz, CDCl3) δH (ppm) = 1.09 (s, 6H, 2 × CH3); 1.24 (s, 6H, 2 × CH3), 2.51–2.33 (m, 8H, 4 × CH2), 5.55 (s, 1H, CH), 7.11 (d, J = 8.2 Hz, 2H, Ar-H), 7.19 (d, J = 6.9 Hz, 2H, Ar-H), 7.26 (s, 1H, Ar-H), 7.27 (s, 1H, NH). 13C NMR (100 MHz, CDCl3) δC (ppm) = 27.5, 29.8, 31.5, 32.8, 47.1, 115.7, 125.9, 126.8, 128.1, 138.1, 189.5, 190.6.
4. Conclusion
In conclusion, we have successfully synthesized a novel Keggin-type molybdo-substituted tungstophosphoric acid (H3PW7Mo5O40·12H2O) catalyst, which has been impregnated with acidified bentonite clay. The catalytic activity of PW7Mo5/bentonite was probed using the one-pot synthesis of the 1,8-dioxo-octahydroxanthene and 1,8-dioxo-decahydroacridine derivatives. The 20% PW7Mo5/bentonite exhibited a higher catalytic activity than the bulk PW7Mo5 catalyst, as well as 10%, 15% and 25% PW7Mo5/bentonite. The effects of various parameters, such as the catalyst loading, amount of catalyst, effect of the solvents, influence of the temperature on the rate of reaction, comparison of different catalysts and green metrics, were discussed in detail. The PW7Mo5/bentonite at 20% was found to be the most active, stable, and reusable catalyst. The PW7Mo5/bentonite catalysts have great potential for application as profitable catalysts for encouraging acid-catalysed organic transformations under environmentally friendly conditions and processes.
Conflicts of interest
There are no conflicts to declare.
Acknowledgements
The author SGS is thankful for financial assistance in the form of a minor research project (STAT/VI/RG/DEPT/2019–20/337–38) and UGC-DST SAP from Dr B. A. Marathwada University, Aurangabad (MS), India. The author DSA gratefully acknowledges the University Grants Commission (UGC), New Delhi (India) for a senior research fellowship (SRF).
References
- X. Liu, Y. Zhang, G. Zhao, J. Zhang, S. Ren and G. Fang, ACS Omega, 2019, 4, 18487–18494 CrossRef CAS.
- A. Enferadi-Kerenkan, T.-O. Do and S. Kaliaguine, Catal. Sci. Technol., 2018, 8, 2257–2284 RSC.
- E. Rafiee and S. Eavani, RSC Adv., 2016, 6, 46433–46466 RSC.
- J. Lan, J. Lin, Z. Chen and G. Yin, ACS Catal., 2015, 5, 2035–2041 CrossRef CAS.
- Y. Zhang, Z. Shen, J. Tang, Y. Zhang, L. Kong and Y. Zhang, Org. Biomol. Chem., 2006, 4, 1478–1482 RSC.
- D. Borkin, E. Morzhina, S. Datta, A. Rudnitskaya, A. Sood, M. Torok and B. Torok, Org. Biomol. Chem., 2011, 9, 1394–1401 RSC.
- H. Aghayan, A. R. Khanchi, T. Yousefi and H. Ghasemi, J. Nucl. Mater., 2017, 496, 207–214 CrossRef CAS.
- H. Wu, M. Zhou, Y. Qu, H. Li and H. Yin, Chin. J. Chem. Eng., 2009, 17, 200–206 CrossRef CAS.
- A. O. Terent'Ev, I. A. Yaremenko, V. A. Vil', I. K. Moiseev, S. A. Kon'Kov, V. M. Dembitsky, D. O. Levitsky and G. I. Nikishin, Org. Biomol. Chem., 2013, 11, 2613–2623 RSC.
- C. Tagusagawa, A. Takagaki, K. Takanabe, K. Ebitani, S. Hayashi and K. Domen, J. Phys. Chem. C, 2009, 113, 17421–17427 CrossRef CAS.
- J. Xu, R. W. Gable and C. Ritchie, Acta Crystallogr., Sect. C: Struct. Chem., 2018, 74, 1384–1389 CrossRef CAS PubMed.
- E. Rezaei-Seresht, F. M. Zonoz, M. Estiri and R. Tayebee, Ind. Eng. Chem. Res., 2011, 50, 1837–1846 CrossRef CAS.
- L. R. V. da Conceicao, L. M. Carneiro, D. S. Giordani and H. F. de Castro, Renewable Energy, 2017, 113, 119–128 CrossRef CAS.
- L. D. Chavan and S. G. Shankarwar, Chin. J. Catal., 2015, 36, 1054–1059 CrossRef CAS.
- N. C. Coronel, M. J. da Silva, S. O. Ferreira, R. C. da Silva and R. Natalino, ChemistrySelect, 2019, 4, 302–310 CrossRef CAS.
- J. E. Molinari, L. Nakka, T. Kim and I. E. Wachs, ACS Catal., 2011, 1, 1536–1548 CrossRef CAS.
- H. Cai, X. Wu, Q. Wu and W. Yan, Dalton Trans., 2016, 45, 14238–14242 RSC.
- J. Li, X. Wang, W. Zhu and F. Cao, ChemSusChem, 2009, 2, 177–183 CrossRef CAS PubMed.
- Z. Xie, H. Wu, Q. Wu and L. Ai, RSC Adv., 2018, 8, 13984–13988 RSC.
- L. Rozic, B. Grbic, N. Radic, S. Petrovic, T. Novakovic, Z. Vukovic and Z. Nedic, Appl. Clay Sci., 2011, 53, 151–156 CrossRef CAS.
- V. V. Bokade and G. D. Yadav, Appl. Clay Sci., 2011, 53, 263–271 CrossRef CAS.
- G. Yadav, J. Catal., 2003, 217, 88–99 CAS.
- L. Y. Zhang, S. Y. Cai, J. H. Mo, G. T. Wei, Z. M. Li, R. C. Ye and X. M. Xie, Mater. Manuf. Processes, 2015, 30, 279–284 CrossRef CAS.
- A. d. N. de Oliveira, M. A. Barbosa de Lima, L. H. de Oliveira Pires, M. Rosas da Silva, P. T. Souza da Luz, R. S. Angelica, G. N. da Rocha Filho, C. E. F. da Costa, R. Luque and L. A. Santos do Nascimento, Materials, 2019, 12, 1431 CrossRef PubMed.
- D. S. Moraes, R. S. Angélica, C. E. F. Costa, G. N. Rocha Filho and J. R. Zamian, Appl. Clay Sci., 2010, 48, 475–480 CrossRef CAS.
- R. Liu, X. Xia, X. Niu, G. Zhang, Y. Lu, R. Jiang and S. He, Appl. Clay Sci., 2015, 105–106, 71–77 CrossRef CAS.
- R. A. Valiulin, L. M. Halliburton and A. G. Kutateladze, Org. Lett., 2007, 9, 4061–4063 CrossRef CAS PubMed.
- A. Domling, W. Wang and K. Wang, Chem. Rev., 2012, 112, 3083–3135 CrossRef CAS PubMed.
- Y. Liu, X. H. Zhang, J. Ren and G. Y. Jin, Synth. Commun., 2004, 34, 151–157 CrossRef CAS.
- S. Brauch, S. S. van Berkel and B. Westermann, Chem. Soc. Rev., 2013, 42, 4948 RSC.
- Subodh, N. K. Mogha, K. Chaudhary, G. Kumar and D. T. Masram, ACS Omega, 2018, 3, 16377–16385 CrossRef CAS PubMed.
- G. Shabir, A. Saeed and P. Ali Channar, Mini-Rev. Org. Chem., 2018, 15, 166–197 CrossRef CAS.
- S. A. Hilderbrand and R. Weissleder, Tetrahedron Lett., 2007, 48, 4383–4385 CrossRef CAS PubMed.
- B. B. Bhowmik and P. Ganguly, Spectrochim. Acta, Part A, 2005, 61, 1997–2003 CrossRef PubMed.
- R. Singh and G. Panda, Org. Biomol. Chem., 2010, 8, 1097 RSC.
- S. Girault, P. Grellier, A. Berecibar, L. Maes, E. Mouray, P. Lemière, M.-A. Debreu, E. Davioud-Charvet and C. Sergheraert, J. Med. Chem., 2000, 43, 2646–2654 CrossRef CAS PubMed.
- C. Teixeira, N. Vale, B. Perez, A. Gomes, J. R. B. Gomes and P. Gomes, Chem. Rev., 2014, 114, 11164–11220 CrossRef CAS PubMed.
- A. Kumar, L. Rout, L. S. K. Achary, R. S. Dhaka and P. Dash, Sci. Rep., 2017, 7, 42975 CrossRef PubMed.
- S. Hatakeyama, N. Ochi, H. Numata and S. Takano, J. Chem. Soc., Chem. Commun., 1988, 64, 1202–1204 RSC.
- A. Amoozadeh, S. Rahmani, M. Bitaraf, F. B. Abadi and E. Tabrizian, New J. Chem., 2016, 40, 770–780 RSC.
- M. Gensicka-Kowalewska, G. Cholewiński and K. Dzierzbicka, RSC Adv., 2017, 7, 15776–15804 RSC.
- G. Rathee, S. Kohli, N. Singh, A. Awasthi and R. Chandra, ACS Omega, 2020, 5, 15673–15680 CrossRef CAS PubMed.
- G. Song, B. Wang, H. Luo and L. Yang, Catal. Commun., 2007, 8, 673–676 CrossRef CAS.
- A. Ilangovan, S. Malayappasamy, S. Muralidharan and S. Maruthamuthu, Chem. Cent. J., 2011, 5, 81 CrossRef CAS PubMed.
- L. Q. Wu, Y. F. Wu, C. G. Yang, L. M. Yang and L. J. Yang, J. Braz. Chem. Soc., 2010, 21, 941–945 CrossRef CAS.
- X. Fan, X. Hu, X. Zhang and J. Wang, Can. J. Chem., 2005, 83, 16–20 CrossRef CAS.
- S. M. Vahdat, S. Khaksar, M. Akbari and S. Baghery, Arabian J. Chem., 2019, 12, 1515–1521 CrossRef CAS.
- M. Hong and G. Xiao, J. Fluorine Chem., 2012, 144, 7–9 CrossRef CAS.
- B. Das, P. Thirupathi, I. Mahender, V. S. Reddy and Y. K. Rao, J. Mol. Catal. A: Chem., 2006, 247, 233–239 CrossRef CAS.
- S. S. Kahandal, A. S. Burange, S. R. Kale, P. Prinsen, R. Luque and R. V. Jayaram, Catal. Commun., 2017, 97, 138–145 CrossRef CAS.
- K. Venkatesan, S. S. Pujari, R. J. Lahoti and K. V. Srinivasan, Ultrason. Sonochem., 2008, 15, 548–553 CrossRef CAS PubMed.
- A. N. Dadhania, V. K. Patel and D. K. Raval, J. Saudi Chem. Soc., 2017, 21, S163–S169 CrossRef CAS.
- K. Niknam, F. Panahi, D. Saberi and M. Mohagheghnejad, J. Heterocycl. Chem., 2010, 47, 292 CAS.
- M. Dabiri, M. Baghbanzadeh and E. Arzroomchilar, Catal. Commun., 2008, 9, 939–942 CrossRef CAS.
- A. Ilangovan, S. Muralidharan, P. Sakthivel, S. Malayappasamy, S. Karuppusamy and M. P. Kaushik, Tetrahedron Lett., 2013, 54, 491–494 CrossRef CAS.
- F. Rashedian, D. Saberi and K. Niknam, J. Chin. Chem. Soc., 2010, 57, 998–1006 CrossRef CAS.
- P. S. Bhale, S. B. Dongare and Y. B. Mule, Chem. Sci. Trans., 2015, 4, 246–250 Search PubMed.
- Z. H. Zhang and Y. H. Liu, Catal. Commun., 2008, 9, 1715–1719 CrossRef CAS.
- Z.-H. Zhang and X.-Y. Tao, Aust. J. Chem., 2008, 61, 77 CrossRef CAS.
- D. S. Aher, K. R. Khillare, L. D. Chavan and S. G. Shankarwar, ChemistrySelect, 2020, 5, 7320–7331 CrossRef CAS.
- D. S. Aher, K. R. Khillare, L. D. Chavan and S. G. Shankarwar, RSC Adv., 2021, 11, 2783–2792 RSC.
- S. K. Bhorodwaj and D. K. Dutta, Appl. Clay Sci., 2011, 53, 347–352 CrossRef CAS.
- L. V. Chopda and P. N. Dave, ChemistrySelect, 2020, 5, 2395–2400 CrossRef CAS.
- G. M. Ziarani, A. Badiei, M. Hassanzadeh and S. Mousavi, Arabian J. Chem., 2014, 7, 335–339 CrossRef CAS.
- M. Nasr-Esfahani, M. Montazerozohori and T. Abdizadeh, C. R. Chim., 2015, 18, 547–553 CrossRef CAS.
- T.-S. Jin, J.-S. Zhang, T.-T. Guo, A.-Q. Wang and T.-S. Li, Synthesis, 2004, 2004, 2001–2005 CrossRef.
- C. R. McElroy, A. Constantinou, L. C. Jones, L. Summerton and J. H. Clark, Green Chem., 2015, 17, 3111–3121 RSC.
- R. A. Sheldon, ACS Sustainable Chem. Eng., 2018, 6, 32–48 CrossRef CAS.
- M. Tobiszewski, M. Marć, A. Gałuszka and J. Namieśnik, Molecules, 2015, 20, 10928–10946 CrossRef CAS PubMed.
- R. A. Sheldon, Green Chem., 2007, 9, 1273 RSC.
Footnote |
† Electronic supplementary information (ESI) available. See DOI: 10.1039/d1ra01179k |
|
This journal is © The Royal Society of Chemistry 2021 |