DOI:
10.1039/D1RA00925G
(Review Article)
RSC Adv., 2021,
11, 14251-14259
Synthetic applications of flavin photocatalysis: a review
Received
3rd February 2021
, Accepted 28th March 2021
First published on 16th April 2021
Abstract
Encouraging developments in the field of photocatalysis in last decades, biomolecules namely flavins have been observed to act as a catalyst in several photoredox-catalysed synthetic methodologies. Due to its excellent redox window and good chemical stability, this promising photoactive biological molecule has emerged as a powerful and attractive metal-free organophotocatalyst. This review highlights the design, properties, biosynthesis and application of flavin photoredox catalysts and is expected to contribute to a great extent towards the advancement and development of synthetic methodologies.
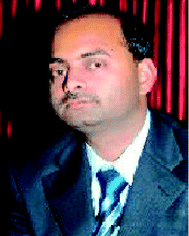 Vishal Srivastava | Vishal Srivastava is working as Assistant Professor, Department of Chemistry, C. M. P. College, (Constituent P. G. College of Central University of Allahabad) Prayagraj, India. He has completed Graduation (B.Sc.), Post-Graduation (M.Sc.) in Organic Chemistry and Doctoral Degree (D.Phil.) from Department of Chemistry, University of Allahabad, India. His current research work involves the designing of novel biologically active photoredox catalysed synthetic organic compounds. |
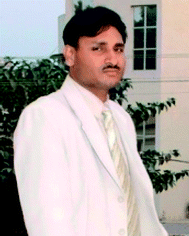 Pravin K. Singh | Pravin K. Singh is working as Assistant Professor, Department of Chemistry, C. M. P. College, Allahabad, India (Constituent P. G. College of Central University of Allahabad). Dr Singh is actively engaged in advanced research work for the development of environmentally benign, new synthetic routes for various bioactive heterocyclic compounds. He has completed his B.Sc., M.Sc., Doctorate (D.Phil.) and Post-Doctorate (D.Sc.) from the University of Allahabad, India. |
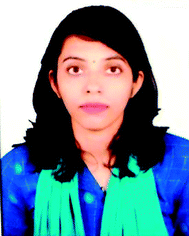 Arjita Srivastava | Arjita Srivastava is working as Assistant Professor in the Department of Chemistry, C. M. P. P. G College, Prayagraj. She has completed her B.Sc. from Ewing Christian College, University of Allahabad, and her M.Sc. in Organic Chemistry, D.Phil. from the Department of Chemistry, University of Allahabad, India. Her current research interest is mainly focused on utilizing visible light and efficient photocatalysts as green tools for the synthesis of bio-active compounds. |
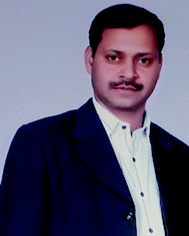 Praveen P. Singh | Praveen P. Singh is working as Assistant Professor in the Department of Chemistry at the United College of Engineering and Research, Prayagraj, India. He obtained his B.Sc., M.Sc. in Organic Chemistry from T. D. P. G. College (V. B. S. Purvanchal University) Jaunpur and D.Phil. from the Department of Chemistry, University of Allahabad, India. His current research interests include the development of synthetic receptors for the recognition of biological target structures and the application of visible light chemical photocatalysis towards organic synthesis. |
1. Introduction
In recent years, both academia and industry1–10 have attracted extensive interest in the context of green and sustainable chemistry due to the development of clean, economical and efficient chemical processes. Over the last decade, visible light photocatalysis has evolved into a widely used method in organic syntheses as it is the most sustainable reaction inducer and has been increasingly used as a powerful strategy to promote numerous synthetic transformations in organic chemistry11 as it is a safe, renewable, and inexpensive source of chemical energy. Solar energy (visible light) has great prospects in developing sustainable and eco-friendly protocols and can be used in organic synthesis12 because it is clean, easy to handle and an unlimited energy source. The conversion of solar energy into chemical energy for chemical transformations,13,14 has fascinated some researchers, who have incorporated an excellent tactic for the implementation of photoredox catalysts to commence single electron transfer (SET) processes.15,16 In organic synthesis, the visible light photoredox catalysis has received considerable attention owing to the ready availability, non-toxicity and ease-of-handling.17 In the last few decades, several metal-free organic dyes, such as eosin Y, rose bengal, Nile red, fluorescein, rhodamine B and perylene, have been applied as inexpensive and environmentally benign preferable alternatives to Ru(II) and Ir(II) complexes in visible-light promoted organic transformations involving SET.18,19 These organic dyes can serve as a superior alternative to transition metal photoredox catalysts, and have great potential for application in visible-light mediated organic synthesis.20 In spite of these facts, the general synthetic utility of organic dyes is still rather limited due to relatively few catalyst options.
The application of inexpensive organic dyes has received increasing attention since some chromophores allow access to unique chemical reactivity.21 Their use has been restrained22 due to far more limited availability of their spectroscopic, kinetic, and electrochemical data in comparison to the transition-metal catalysts and for most organic dyes the reactive triplet state is not characterized by charge separation.23 Furthermore, not all of these dyes can engage in both oxidative and reductive quenching, as well as due to its poor recyclability these organic dyes have limited widespread application.
Flavin catalysis is recognized as an attractive approach for designing green and sustainable transformations. Recently, one of the most widespread examples of dye-based photoredox catalysts is vitamin B2, riboflavin (RF)24 and its derivatives, which have received remarkable attention as unique redox organocatalysts that promote numerous catalytic oxidations.25,26 Flavin derivatives (FLs), serving as the building blocks of redox-active coenzymes, are the reaction sites of numerous thermal and photoinduced processes and are tolerant to the variation of redox potentials.27–29 Riboflavin and its derivatives exhibit some advantages: among others, they can have maximum absorption in the blue region of the visible light spectrum with high molar absorption coefficients. These photocatalysts are easily accessible, providing a versatile and green method for numerous organic chemical reactions.30 (−)-Riboflavin (vitamin B2) is a highly versatile organocatalyst for a variety of transformations31 due to its inherent energy transfer (ET) and single electron transfer (SET) modes that can be activated upon irradiation.32 Therefore, it is highly desirable to design new organic photocatalysts with broad redox capabilities. Thus, in modern organic synthesis, flavins exhibit a superior performance in comparison to other photocatalysts due to their better photostability, which will ultimately bring about the future development trend of organic photocatalysts.
Recently, flavin and its derivatives have been found to be ingenious photocatalysts for various reactions (Fig. 1) and emerged as outstanding organic photoredox catalysts for diverse synthetic organic transformations. As this is a robust emerging field, a summary of flavins for organic transformation is highly appreciable. In continuation of our study on photocatalysed organic syntheses,33 in this study, the recent trends in using flavins as a visible light photocatalyst in organic synthesis are reviewed.
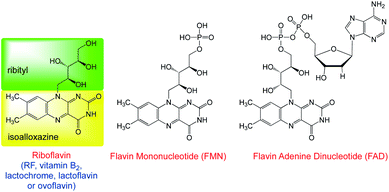 |
| Fig. 1 Naturally occurring flavins. | |
2. Biosynthesis of riboflavin
The biosynthesis of riboflavin was investigated by Bachner et al.34 in 2000. One riboflavin molecule requires one molecule of GTP and two molecules of ribulose 5-phosphate as substrates (Scheme 1). Most commercial riboflavin is currently produced or was produced earlier via microbial synthesis using specially selected strains of bacteria and fungi.
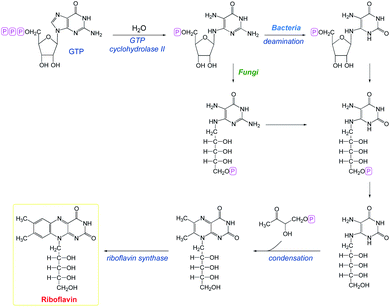 |
| Scheme 1 Biosynthesis of riboflavin.34 | |
3. Flavin-related compounds in photocatalysis
Besides flavin related compounds48 that have also been applied in photoredox catalysis, some of them are naturally occurring, while few compounds are semi-synthetic (Fig. 2).
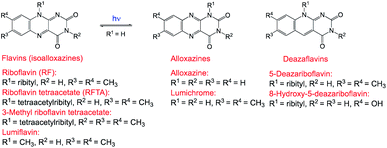 |
| Fig. 2 Structure of flavin-related compounds.48 | |
4. Photochemistry of flavin
Three redox states: oxidized, one-electron reduced (as semiquinone) or reduced by two electrons, exhibited by flavins, and each of these redox states has three different protonation states (Scheme 2). Substitution, noncovalent interactions such as hydrogen bonds, and nature of the surrounding protein affect the redox properties, absorption and reactivity of flavins. From electrochemical studies, in water and in protic organic solvents, flavins are reduced reversibly by a two-electron process; the reduction potential of RF is about −0.507 V (vs. ferrocene/ferrocenium (Fc/Fc+)) in water. Two consecutive one-electron reductions are observed in aprotic media. The reduction potentials of riboflavin tetraacetate (RFTA), −1.18 V and −1.87 V (vs. Fc/Fc+), measured in acetonitrile.35–48
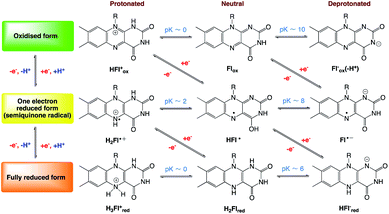 |
| Scheme 2 Different redox and protonation states of flavins (10-substituted isoalloxazines).35–48 | |
In flavin photocatalysis, its photocatalytic cycle starts with the irradiation of the oxidized form of flavin (Flox) with blue light exciting it into the singlet state (1Fl*), followed by an intersystem crossing (ISC) to the triplet state (3Fl*) rapidly. In catalysis, the triplet state is the active species and key intermediate, which can then be reduced by an appropriate substrate to the radical anion (Fl˙−), which is subsequently protonated and further reduced to the flavohydro-quinone anion (HFlred−).
The different redox and protonation states of flavin and their derivative compounds affect the photo absorption properties in context of fluorescence intensity and excited-state lifetimes, which have been found lower for alloxazines than those for flavins (Table 1 for comparison).49
Table 1 Spectral and electrochemical characteristics of the selected flavin derivatives in acetonitrile49
Compound |
λ1a (nm) |
λ2a (nm) |
λFb (nm) |
ΦFc |
τFd |
E0e (V) |
λ1 and λ2 are the positions of the two lowest energy bands in the absorption spectra. Maximum of the fluorescence emission spectrum. Fluorescence quantum yield. Fluorescence lifetime. Reversible redox potentials (Fl → Fl− and Fl− → Fl− in the brackets) measured by CV using SCE as standard electrode. Value for 3,10-dimethyl-5-ethyl flavinium (R = Me). |
Riboflavin tetraacetate |
440 |
343 |
505 |
0.37 |
6.8 ns |
−1.18 (−1.87) |
Lumiflavin |
443 |
342 |
533 |
0.16 |
7.7 ns |
−0.761 |
5-Deazariboflavin |
399 |
329 |
457 |
0.11 |
4.03 ns |
— |
Lumichrome |
380 |
334 |
437 |
0.028 |
0.64 ns |
−1.3 |
5EtFl+ClO4− |
557 |
414 |
661 |
— |
590 ps |
0.306 (−0.389)f |
5EtFlOH |
348 |
— |
496 |
0.0003 |
500 fs |
— |
The general mechanistic pathway for flavin photocatalysis has been depicted in Scheme 3.50
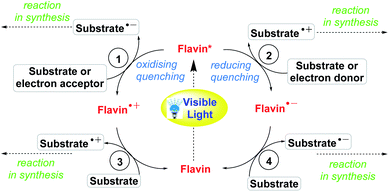 |
| Scheme 3 General mechanisms of flavin catalysis.50 | |
5. Synthetic applications of flavin
Flavin photocatalysis is an ingenious and green protocol for numerous organic transformations. Since riboflavin has emerged as a stable photocatalyst, as a result of which in recent years several new reactions have been reported indicating the scope and applicability of flavin, including cyclization, decarboxylative cyanation, aerobic oxidation, nitration, E → Z isomerization, chemoselective synthesis and oxyamination.
5.1. Cyclization of thiobenzanilides
Schmidt et al.51 reported an efficient photochemical approach for the cyclisation of thiobenzanilides. They performed visible light irradiated synthesis of benzothiazoles from thiobenzanilides using riboflavin as a photocatalyst and potassium peroxydisulfate as a sacrificial oxidizing agent up to 97% yield (Scheme 4).
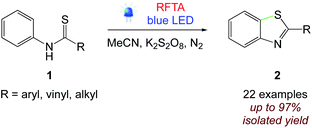 |
| Scheme 4 Visible light-mediated cyclisation of thiobenzanilides.51 | |
A plausible mechanism is shown in Scheme 5. Upon the photogeneration of singlet and/or triplet excited states of RFTA, thiobenzanilides can act as a quencher by an electron-transfer reaction, giving rise to the corresponding thiobenzanilide radical cation (1˙+) along with the radical anion RFTA˙−. Notably, the riboflavin moiety can also act as a base (pKa of [RFTA-H]˙ = 8.3), assisting the deprotonation of 1˙+. The deprotonation of the thiobenzanilide radical cation 1˙+ gives the sulfur radical, which undergoes cyclization and further rearomatization by any oxidant species affording 2-substituted benzothiazoles 2. Peroxydisulfate is necessary to close the catalytic cycle and return RFTA to its ground state.
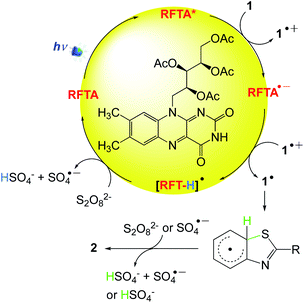 |
| Scheme 5 A plausible mechanism for the cyclisation of thiobenzanilides via visible light photocatalysis.51 | |
5.2. Direct cyclization
The photocatalytic synthesis of benzo-3,4-coumarins 4 directly from biaryl carboxylic acids 3 without the need for substrate prefunctionalization was investigated by Gilmour et al.52 (Scheme 6). This disconnection relies on the oxidation competence of photoactivated (−)-riboflavin to generate the heterocyclic core via photoinduced single electron transfer.
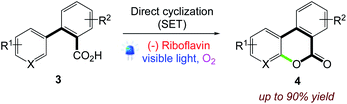 |
| Scheme 6 Direct, photocatalytic synthesis of benzocoumarins via (−)-riboflavin-mediated electron transfer.52 | |
They proposed that singlet state electron transfer from benzoic acid to excited state (−)-riboflavin [E(3RF*/RF˙−) = 1.46 V vs. SCE] is operational (Scheme 7).53 The catalytic cycle is likely initiated by the protonation of (−)-riboflavin (RF) with the strong absorption of the protonated flavin (Scheme 7, RFH+) occurring at λmax = 402 nm. Subsequent single electron transfer from the carboxylate anion (3a) to RFH+ yields the protonated flavin radical (RFH˙) and the carboxy radical (3b), which can undergo rapid cyclization to intermediate 3c. Final oxidation and rearomatization of 3c with RFH˙ in a hydrogen atom transfer or SET/deprotonation reaction releases the desired product. The reduced (−)-riboflavin (RFH2) can, in turn, be reoxidized by molecular oxygen as well as the oxidation of intermediate 3c and RFH˙ by molecular oxygen cannot be ignored.
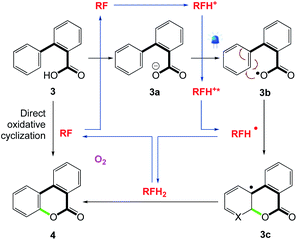 |
| Scheme 7 A plausible mechanism for the direct, photocatalytic synthesis of benzocoumarins.53 | |
5.3. Decarboxylative cyanation of aliphatic carboxylic acids
Gomez et al.54 reported the decarboxylative cyanation of aliphatic carboxylic acids at room temperature. Riboflavin tetraacetate promotes the visible light-irradiated oxidation of carboxylic acids. After decarboxylation, the generated radicals are trapped by TsCN, yielding the desired nitriles without any further additive, in a redox-neutral process (Scheme 8). This protocol can be adapted to flow conditions.
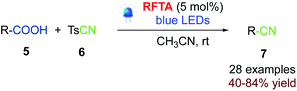 |
| Scheme 8 Decarboxylative cyanation of aliphatic carboxylic acids.54 | |
Mechanistically, they anticipated that, upon irradiation with visible light (λmax ≈ 450 nm) and after rapid intersystem crossing, the long-lived triplet-excited state of flavin would be oxidant enough (Ered = +1.50 V vs. SCE) to undergo the single-electron oxidation of aliphatic carboxylates [Ered from +1.0 V to +1.50 V vs. SCE]. Importantly, the flavin moiety can also act as a base, favoring the deprotonation of carboxylic acid prior to its single electron oxidation, or in a proton-coupled electron transfer (PCET). After the rapid decarboxylation of the aliphatic acyloxyl radical, the generated radical is intercepted by Ts-CN, affording the desired nitrile and p-toluenesulfonyl radical (Ts˙). The latter radical could be reduced by the hydroflavin radical, regenerating the photocatalyst and producing TsH after protonation, in a redox-neutral process. Alternatively, the p-toluenesulfonyl radical could abstract a hydrogen atom (HAT) from FH˙ to turn over the photocatalyst (Scheme 9).
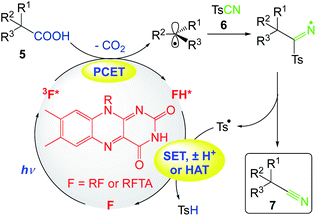 |
| Scheme 9 Plausible mechanism of the decarboxylative cyanation of aliphatic carboxylic acids.54 | |
5.4. Aerobic oxidation of benzylic C–H bonds
Pasau and co-workers55 reported a continuous mesofluidic process for benzylic C–H oxidation with moderate to good yields using a flavin photocatalyst upon visible-light irradiation and an iron additive [Fe(ClO4)2] via the incorporation of singlet oxygen (1O2) for the direct formation of oxidized C
O or CH–OH compounds (Scheme 10).
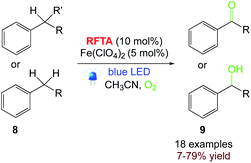 |
| Scheme 10 Aerobic oxidation of benzylic C–H bonds.55 | |
A plausible mechanism is shown in Scheme 11. Upon irradiation, photocatalyst (RFT), is excited to its triplet state (RFT*) and can undergo consecutive electron reduction and protonation, leading concomitantly to the formation of RFTH2 and the radical (R˙, 8a). At this stage, the 1O2 singlet will react with the radical species to generate the peroxo-radical (ROO˙, 8b) intermediate, affording the ketone or alcohol precursor of the desired products. 1O2 will then oxidize the intermediate RFTH2 in order to regenerate the catalyst and form the H2O2 side product. Finally, hydrogen peroxide is reduced to O2 and H2O in the presence of an iron additive.
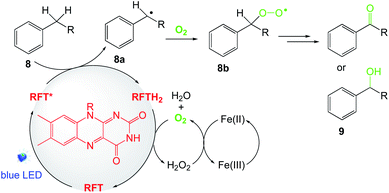 |
| Scheme 11 Plausible mechanism of the aerobic oxidation of benzylic C–H bonds.55 | |
5.5. Nitration of protected anilines
König et al.56 investigated the nitration of protected anilines with riboflavin tetraacetate (RFTA) upon visible light irradiation. In this protocol, sodium nitrite serves as the NO2 source in the visible-light driven room temperature reaction. Numerous nitro anilines are obtained in moderate to good yields without the addition of acid or stoichiometric oxidation agents. The catalytic cycle is closed by aerial oxygen as the terminal oxidant (Scheme 12).
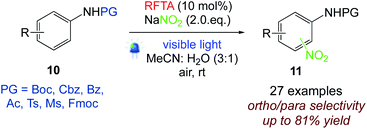 |
| Scheme 12 Nitration of protected anilines.56 | |
After excitation, photocatalyst (RFTA) oxidizes the aniline derivative 10.57,58 The acidity of radical cations increases compared to the neutral compound, so the consecutive formation of the stabilized radical 10a via the loss of a proton can occur.59,60 The radical species nitrogen dioxide is formed via different pathways and is able to react with 10a.61,62 After rearomatization, the desired para- and ortho-regioisomeric substitution products 11 are obtained (Scheme 13).
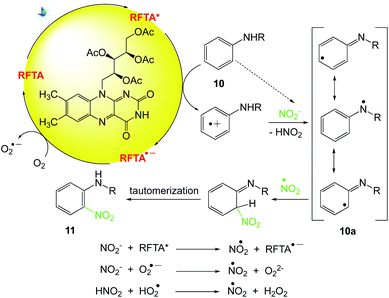 |
| Scheme 13 Plausible mechanism of nitration of protected anilines.56 | |
5.6. E → Z isomerization of polarized alkenes (olefins)
Metternich et al.63,64 reported the first highly Z-selective isomerization 13 of polarized alkenes 12, catalysed by riboflavin under visible light irradiation. This study was inspired by the susceptibility of crystalline (−)-riboflavin in the eyes of vertebrates to invert the intrinsic directionality of retinal isomerization. They demonstrated broad substrate scope (up to 99
:
1 Z
:
E) along with the evidence of mechanistic dichotomy via both singlet and triplet energy transfer mechanisms (Scheme 14). The simple E → Z isomerization of activated dienes, based on the β-ionyl motif intrinsic to retinal, is also reported by Gilmour et al.65 by using inexpensive (−)-riboflavin (vitamin B2) under irradiation at 402 nm.
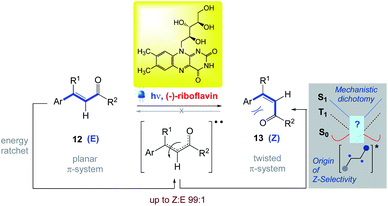 |
| Scheme 14 Photocatalytic isomerization of polarized alkenes.63,64 | |
5.7. Chemoselective synthesis of unsymmetrical disulfides
Iida et al.66 have developed the chemoselective synthesis of unsymmetrical disulfides using the oxidative heterocoupling reaction of two different thiols. The riboflavin-based catalyst successfully promoted the formation of the phototropin-like flavin–thiol adduct under visible light irradiation, the mild reactivity of adduct, played a crucial role in the chemoselective cross-coupling. This green oxidative transformation is driven by visible light due to the photo and redox-organocatalysis of flavin, and molecular oxygen under mild metal-free conditions (Scheme 15).
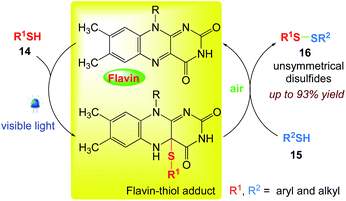 |
| Scheme 15 Flavin–thiol adduct-mediated heterocoupling reaction.66 | |
6. Cooperative catalysis
Cooperative catalysis is applicable when two catalysts and two catalytic cycles work in concert to generate a single new bond in organic transformations. Being a stable photocatalyst, flavin is also applied as a cooperative catalyst in combination with other organocatalyst and flavin–amine hybrid to carry out aerobic oxidations as well as oxyamination reactions.
6.1. Aerobic oxidation of unactivated benzylic substrates
Cibulka et al.67 reported a system with ethylene-bridged flavinium salt (combining flavin photocatalysis and organocatalysis 18b), which catalyzes the aerobic oxidation of toluenes and benzyl alcohols 19 with high oxidation potential (Eox > +2.5 V vs. SCE) to give the corresponding benzoic acids 20 under visible light irradiation (Scheme 17). This is caused by the high oxidizing power of excited 17b (E(2b*) = +2.67 V vs. SCE) involved in photooxidation and by the accompanying dark organocatalytic oxygenation provided by the in situ formed flavin hydroperoxide 18b–OOH (Scheme 16).68–76
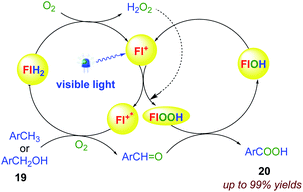 |
| Scheme 16 Aerobic oxidation of unactivated benzylic substrates.67 | |
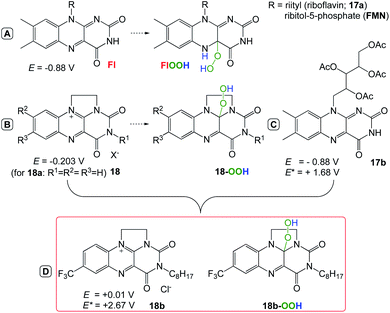 |
| Scheme 17 Activation modes of flavin “co-factors” for oxidations in natural and artificial systems.68–76 | |
6.2. Peptide bridged flavin–amine hybrid
Imada et al.77 reported a peptide-bridged flavin–amine hybrid that can catalyze the α-oxyamination of aldehydes 21 with TEMPO 22 under weak blue light irradiation to achieve an extremely high quantum yield of reaction (Φ = 0.80). The flavin ring system and a secondary amine have been integrated through a short peptide linker with the aim of using photons as efficiently as possible in the photoredox/enamine dual catalysis (Scheme 18).
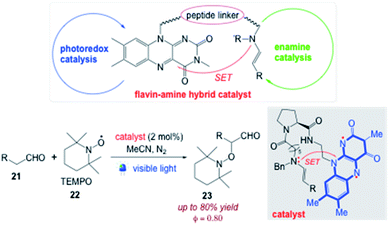 |
| Scheme 18 Efficient use of photons in photoredox/enamine dual catalysis with a peptide-bridged flavin–amine hybrid.77 | |
7. Conclusions
Flavins and their derivatives can serve as a green and versatile catalyst to carry out several organic transformations. In the last few years, few flavin catalysed chemical reactions were reported that clearly indicate that properties and possible synthetic applications of flavins and their derivatives are still in their preliminary stage, which needs to be further explored to realize its full potential. The photostability of the flavin catalyst must be enhanced. For the application of the oxidised form of the flavin catalyst with higher substrate concentration and substrate binding sites, the ISC rate to triplet state after excitation must be enhanced and further exploration is needed to use the reduced form of flavin in organic transformations. In summary, flavins and their derivatives have been used as simple, economical and metal-free photocatalysts for organic transformation recently, with a view of exploring new routes for the development of a novel visible light-induced organic synthesis and it will explore a new era for the biomaterial-based photoredox synthesis.
Conflicts of interest
There are no conflicts to declare.
References
- X. Feng, T. Yang, X. He, B. Yu and C. W. Hu, Appl. Organomet. Chem., 2018, 32, e4314 CrossRef
. - G.-P. Yang, D. Dilixiati, T. Yang, D. Liu, B. Yu and C. W. Hu, Appl. Organomet. Chem., 2018, 32, e4450 CrossRef
. - G. P. Yang, X. He, B. Yu and C. W. Hu, Appl. Organomet. Chem., 2018, 32, e4532 CrossRef
. - G. P. Yang, S. X. Shang, B. Yu and C. W. Hu, Inorg. Chem. Front., 2018, 5, 2472 RSC
. - C. Jing, X. Chen, K. Sun, Y. Yang, T. Chen, Y. Liu, L. Qu, Y. Zhao and B. Yu, Org. Lett., 2019, 21, 486 CrossRef CAS PubMed
. - G. P. Yang, X. Wu, B. Yu and C. W. Hu, ACS Sustainable Chem. Eng., 2019, 7, 3727 CrossRef CAS
. - L. Y. Xie, Y. Duan, L. H. Lu, Y. J. Li, S. Peng, C. Wu, K. J. Liu, Z. Wang and W. M. He, ACS Sustainable Chem. Eng., 2017, 5, 10407 CrossRef CAS
. - L. Y. Xie, Y. J. Li, J. Qu, Y. Duan, J. Hu, K. J. Liu, Z. Cao and W. M. He, Green Chem., 2017, 19, 5642 RSC
. - L. Y. Xie, S. Peng, J. X. Tan, R. X. Sun, X. Yu, N. N. Dai, Z. L. Tang, X. Xu and W. M. He, ACS Sustainable Chem. Eng., 2018, 6, 16976 CrossRef CAS
. - L. Y. Xie, S. Peng, F. Liu, Y. F. Liu, M. Sun, Z. Tang, S. Jiang, Z. Cao and W.-M. He, ACS Sustainable Chem. Eng., 2019, 7, 7193 CrossRef CAS
. -
(a) J. W. Tucker and C. J. R. Stephenson, J. Org. Chem., 2012, 77(4), 1617 CrossRef CAS PubMed
;
(b) D. R. Heitz, K. Rizwan and G. A. Molander, J. Org. Chem., 2016, 81(16), 7308 CrossRef CAS PubMed
;
(c) F. X. Felpin and S. Sengupta, Chem. Soc. Rev., 2019, 48, 1150 RSC
;
(d) H. Chen, L. Guo and S. Yu, Org. Lett., 2018, 20(19), 6255 CrossRef CAS PubMed
. - X. Sala, I. Romero, M. Rodriguez, L. Escriche and A. Llobet, Angew. Chem., Int. Ed., 2009, 48(16), 2842 CrossRef CAS PubMed
. - D. Mandler and I. Willner, J. Am. Chem. Soc., 1984, 106(18), 5352 CrossRef CAS
. - O. Ishitani, S. Yanagida, S. Takamuku and C. Pac, J. Org. Chem., 1987, 52(13), 2790 CrossRef CAS
. - A. Inagakia and M. Akita, Coord. Chem. Rev., 2010, 254, 1220 CrossRef
. - C. K. Prier, D. A. Rankic and D. W. C. MacMillan, Chem. Rev., 2013, 113(7), 5322 CrossRef CAS PubMed
. -
(a) D. A. Nicewicz and T. M. Nguyen, ACS Catal., 2014, 4(1), 355 CrossRef CAS
;
(b) J. Xie, H. Jina, P. Xu and C. Zhu, Tetrahedron Lett., 2014, 55(1), 218 Search PubMed
;
(c) X. Lang, X. Chen and J. Zhao, Chem. Soc. Rev., 2014, 43(1), 473 RSC
;
(d) J. Hu, J. Wang, T. H. Nguyen and N. Zheng, Beilstein J. Org. Chem., 2013, 9, 1977 CrossRef PubMed
;
(e) D. Rovelli, M. Fagnoni and A. Albini, Chem. Soc. Rev., 2013, 42(1), 97 RSC
;
(f) T. P. Yoon, M. A. Ischay and J. J. Du, Nat. Chem., 2010, 2(7), 527 CrossRef CAS PubMed
. - X. J. Yang, B. Chen, L. Q. Zheng, L. Z. Wu and C. H. Tung, Green Chem., 2014, 16, 1082 RSC
. - D. T. Yang, Q. Y. Meng, J. J. Zhong, M. Xiang, Q. Liu and L. Z. Wu, Eur. J. Org. Chem., 2013, 7528 CrossRef CAS
. -
(a) Y. Q. Zou, J. R. Chen, X. P. Liu, L. Q. Lu, R. L. Davis, K. A. Jørgense and W. J. Xiao, Angew. Chem., Int. Ed., 2012, 51, 784 CrossRef CAS PubMed
;
(b) D. P. Hari and B. König, Org. Lett., 2011, 13(15), 3852 CrossRef CAS PubMed
;
(c) M. Neumann, S. Füldner, B. König and K. Zeitler, Angew. Chem., Int. Ed., 2011, 50(4), 951 CrossRef CAS PubMed
;
(d) V. Rey, S. M. S. Catro, J. E. Arguello and A. B. Peñeñory, Tetrahedron Lett., 2009, 50(33), 4720 CrossRef CAS
. - A. H. Bonardi, F. Dumur, G. Noirbent, J. Laleveé and D. Gigmes, Beilstein J. Org. Chem., 2018, 14, 3025–3046 CrossRef CAS PubMed
. - S. P. Pitre, C. D. McTiernan and J. C. Scaianodue, ACS Omega, 2016, 1, 66–76 CrossRef CAS PubMed
. - U. Resch-Genger, M. Grabolle, S. Cavaliere-Jaricot, R. Nitschke and T. Nann, Nat. Methods, 2008, 5, 763–775 CrossRef CAS PubMed
. - B. König, S. Kümmel and R. Cibulka, Flavin photocatalysis, in Chemical Photocatalysis, ed. B. König, Walter de Gruyter, Berlin, 2013, ch. 4. DOI:10.1515/9783110269246
. -
(a) H. Okai, K. Tanimoto, R. Ohkado and H. Iida, Org. Lett., 2020, 22, 8002 CrossRef CAS PubMed
;
(b) Y. Chevalier, Y. L. T. Ki, D. le Nouen, J. P. Mahy, J. P. Goddard and F. Avenier, Angew. Chem., Int. Ed., 2018, 57, 16412–16415 CrossRef CAS PubMed
;
(c) T. Ishikawa, M. Kimura, T. Kumoi and H. Iida, ACS Catal., 2017, 7, 4986–4989 CrossRef CAS
. -
(a) H. Iida, Y. Imada and S.-I. Murahashi, Org. Biomol. Chem., 2015, 13, 7599–7613 RSC
;
(b) R. Cibulka, Eur. J. Org. Chem., 2015, 2015, 915–932 CrossRef CAS
. - W. Kaim and B. Schwederski, Coord. Chem. Rev., 2010, 254(13−14), 1580–1588 CrossRef CAS
. - M. Murakami, K. Ohkubo and S. Fukuzumi, Chem.–Eur. J., 2010, 16(26), 7820–7832 CrossRef CAS PubMed
. - W. Kaim and B. Schwederski, Pure Appl. Chem., 2004, 76(2), 351–364 CAS
. -
(a) N. P. Ramirez, B. König and J. C. Gonzalez-Gomez, Org. Lett., 2019, 21(5), 1368–1373 CrossRef CAS PubMed
;
(b) J. Špačková, E. Svobodová, T. Hartman, I. Stibor, J. Kopecká, J. Cibulková, J. Chudoba and R. Cibulka, ChemCatChem, 2017, 9(7), 1177–1181 CrossRef
;
(c) T. Hering, B. Mühldorf, R. Wolf and B. König, Angew. Chem., Int. Ed., 2016, 55(17), 5342–5345 CrossRef CAS PubMed
. - V. Massey, Biochem. Soc. Trans., 2000, 28, 283–296 CrossRef CAS PubMed
. -
(a) J. B. Metternich and R. J. Gilmour, Am. Chem. Soc., 2016, 138, 1040–1045 CrossRef CAS PubMed
;
(b) M. P. Plesniak, H.-M. Huang and D. J. Procter, Nat. Rev. Chem., 2017, 1, 0077 CrossRef
;
(c) N. J. Turro, V. Ramamurthy and J. C. Scaiano, Modern Molecular Photochemistry of Organic Molecules, University Science Books, Sausalito, CA, 2010 Search PubMed
;
(d) M. Yan, J. C. Lo, J. T. Edwards and P. S. Baran, J. Am. Chem. Soc., 2016, 138, 12692–12714 CrossRef CAS PubMed
;
(e) A. Studer and D. P. Curran, Angew. Chem., Int. Ed., 2016, 55, 58–102 CrossRef CAS PubMed
;
(f) E. Godineau and Y. Landais, Chem.–Eur. J, 2009, 15, 3044–3055 CrossRef CAS PubMed
. -
(a) V. Srivastava, P. K. Singh and P. P. Singh, Chem. Heterocycl. Compd., 2014, 50(4), 573–578 CrossRef CAS
;
(b) V. Srivastava, P. K. Singh and P. P. Singh, Croat. Chem. Acta, 2014, 87(2), 91–95 CrossRef
;
(c) V. Srivastava, P. K. Singh and P. P. Singh, Croat. Chem. Acta, 2015, 88(1), 59–65 CrossRef CAS
;
(d) V. Srivastava, P. K. Singh and P. P. Singh, Croat. Chem. Acta, 2015, 88(3), 227–233 CrossRef CAS
;
(e) V. Srivastava, P. K. Singh, S. Sinha and P. P. Singh, Rev. Roum. Chim., 2016, 61(10), 755–761 Search PubMed
;
(f) V. Srivastava, P. K. Singh and P. P. Singh, Asian J. Chem., 2016, 28(10), 2159–2163 CrossRef CAS
;
(g) V. Srivastava, P. K. Singh and P. P. Singh, Croat. Chem. Acta, 2017, 90(3), 435–441 CrossRef CAS
;
(h) V. Srivastava and P. P. Singh, RSC Adv., 2017, 7, 31377 RSC
;
(i) V. Srivastava, P. K. Singh, S. Kanaujia and P. P. Singh, New J. Chem., 2018, 42, 688 RSC
;
(j) P. K. Singh, P. P. Singh and V. Srivastava, Croat. Chem. Acta, 2018, 91(3), 383–387 CrossRef
;
(k) V. Srivastava, P. K. Singh and P. P. Singh, Tetrahedron Lett., 2019, 60, 40–43 CrossRef CAS
;
(l) V. Srivastava, P. K. Singh and P. P. Singh, Tetrahedron Lett., 2019, 60, 1333–1336 CrossRef CAS
;
(m) V. Srivastava, P. K. Singh and P. P. Singh, Tetrahedron Lett., 2019, 60, 151041 CrossRef CAS
;
(n) V. Srivastava, P. K. Singh and P. P. Singh, Rev. Roum. Chim., 2020, 65(3), 221–226 CrossRef
;
(o) V. Srivastava, P. K. Singh, A. Srivastava and P. P. Singh, RSC Adv., 2020, 10, 20046–20056 RSC
;
(p) A. Srivastava, P. K. Singh, A. Ali, P. P. Singh and V. Srivastava, RSC Adv., 2020, 10, 39495 RSC
;
(q) P. P. Singh and V. Srivastava, Org. Biomol. Chem., 2021, 19, 313–321 RSC
. - A. Bachner, S. Eberhardt, M. Fischer, K. Kis and G. Richter, Annu. Rev. Nutr., 2000, 20, 153–167 CrossRef PubMed
. - H. Schmaderer, J. Svoboda and B. König, Flavin photocatalysts with substrate binding sites, in Activating Unreactive Substrates: The Role of Secondary Interactions, ed. C. Bolm and E. Hahn, Wiley-VCH, Weinheim, 2009, pp. 349–358 Search PubMed
. - S. Ghisla, W. C. Kenney, W. R. Knappe, W. McIntire and T. P. Singer, Biochemistry, 1980, 19, 2537–2544 CrossRef CAS PubMed
. - B. König, M. Pelka, H. Zieg, T. Ritter, H. Bouas-Laurent and R. Bonneau, et al., J. Am. Chem. Soc., 1999, 121, 1681–1687 CrossRef
. - S. D. Islam, A. Penzkofer and P. Hegemann, Chem. Phys., 2003, 291, 97–114 CrossRef CAS
. - R. J. Kutta, PhD thesis, Universität Regensburg, 2012
. - U. Megerle, M. Wenninger, R. J. Kutta, R. Lechner, B. Konig and B. Dick, et al., Phys. Chem. Chem. Phys., 2011, 13, 8869–8880 RSC
. - D. Meisel and P. Neta, J. Phys. Chem., 1975, 79, 2459–2461 CrossRef CAS
. - E. Amouyal, Sol. Energy Mater. Sol. Cells, 1995, 38, 249–276 CrossRef CAS
. - P. F. Heelis, Chem. Soc. Rev., 1982, 11, 15–39 RSC
. -
(a) G. Eberlein and T. C. Bruice, J. Am. Chem. Soc., 1982, 104, 1449–1452 CrossRef CAS
;
(b) T. C. Bruice, Acc. Chem. Res., 1980, 13, 256–262 CrossRef CAS
. - V. Massey, Biochem. Soc. Trans., 2000, 28, 283–296 CrossRef CAS PubMed
. - D. Rehm and A. Weller, Berichte der Bunsengesellschaft für physikalische Chemie, 1969, 73, 834–839 CAS
. - M. Julliard and M. Chanon, Chem. Rev., 1983, 83, 425–506 CrossRef CAS
. - B. König, S. Kümmel, E. Svobodová and R. Cibulka, Phys. Sci. Rev., 2018, 20170168 Search PubMed
. -
(a) E. Sikorska, I. V. Khmelinskii, W. Prukała, S. L. Williams, M. Patel and D. R. Worrall, et al., J. Phys. Chem., 2004, 108, 1501–1508 CrossRef CAS
;
(b) M. Insińska-Rak, E. Sikorska, J. L. Bourdelande, I. V. Khmelinskii, W. Prukała and K. Dobek, et al., J. Mol. Struct., 2006, 783, 184–190 CrossRef
;
(c) V. Sichula, P. Kucheryavy, R. Khatmullin, Y. Hu, E. Mirzakulova and S. Vyas, et al., J. Phys. Chem., 2010, 114, 12138–12147 CrossRef CAS PubMed
;
(d) Y. Imada, H. Iida, S. Ono, Y. Masui and S. Murahashi, Chem.–Asian J., 2006, 1, 136–147 CrossRef CAS PubMed
;
(e) G. Porcal, S. G. Bertolotti, C. M. Previtali and M. V. Encinas, Phys. Chem. Chem. Phys., 2003, 5, 4123 RSC
;
(f) D. Zhou, E. Mirzakulova, R. Khatmullin, I. Schapiro, M. Olivucci and K. D. Glusac, J. Phys. Chem. B, 2011, 115, 7136–7143 CrossRef CAS PubMed
;
(g) C. Dang, L. Zhu, H. Guo, H. Xia, J. Zhao and B. Dick, ACS Sustainable Chem. Eng., 2018, 6(11), 15254–15263 CrossRef CAS
. - L. Marzo, S. K. Pagire, O. Reiser and B. König, Angew. Chem., Int. Ed., 2018, 57(32), 10034–10072 CrossRef CAS PubMed
. - L. M. Bouchet, A. A. Heredia, J. E. Argüello and L. C. Schmidt, Org. Lett., 2020, 22(2), 610–614 CrossRef CAS PubMed
. - T. Morack, J. B. Metternich and R. Gilmour, Org. Lett., 2018, 20(5), 1316–1319 CrossRef CAS PubMed
. - C. Y. Lu, W. F. Wang, W. Z. Lin, Z. H. Han, S. D. Yao and N. Y. Lin, J. Photochem. Photobiol., B, 1999, 52, 111–116 CrossRef CAS
. - N. P. Ramirez, B. König and J. C. G. Gomez, Org. Lett., 2019, 21(5), 1368–1373 CrossRef CAS PubMed
. - M. Lesieur, C. Genicot and P. Pasau, Org. Lett., 2018, 20(7), 1987–1990 CrossRef CAS PubMed
. - S. J. S. Düsel and B. König, J. Org. Chem., 2018, 83(5), 2802–2807 CrossRef PubMed
. - G. J. Choi and R. R. Knowles, J. Am. Chem. Soc., 2015, 137, 9226 CrossRef CAS PubMed
. - D. C. Miller, G. J. Choi, H. S. Orbe and R. R. Knowles, J. Am. Chem. Soc., 2015, 137, 13492 CrossRef CAS PubMed
. - F. G. Bordwell and J. P. Cheng, J. Am. Chem. Soc., 1989, 111, 1792 CrossRef CAS
. - J. W. Beatty and C. R. J. Stephenson, Acc. Chem. Res., 2015, 48, 1474 CrossRef CAS PubMed
. - P. S. Rao and E. Hayon, J. Phys. Chem., 1975, 79, 397 CrossRef CAS
. - P. M. Wood, Biochem. J., 1988, 253, 287 CrossRef CAS PubMed
. - J. B. Metternich, D. G. Artiukhin, M. C. Holland, M. V. B. Kühne, J. Neugebauer and R. Gilmour, J. Org. Chem., 2017, 82(19), 9955–9977 CrossRef CAS PubMed
. - J. B. Metternich and R. Gilmour, J. Am. Chem. Soc., 2015, 137(35), 11254–11257 CrossRef CAS PubMed
. - K. Livingstone, M. Tenberge, F. Pape, C. G. Daniliuc, C. Jamieson and R. Gilmour, Org. Lett., 2019, 21(23), 9677–9680 CrossRef CAS PubMed
. - M. Oka, D. Katsube, T. Tsuji and H. Iida, Org. Lett., 2020, 22(23), 9244–9248 CrossRef CAS PubMed
. - J. Zelenka, E. Svobodová, J. Tarábek, I. Hoskovcová, V. Boguschová, S. Bailly, M. Sikorski, J. Roithová and R. Cibulka, Org. Lett., 2019, 21(1), 114–119 CrossRef CAS PubMed
. -
(a) J. Dong, E. F. Fueyo, F. Hollmann, C. E. Paul, M. Pesic, S. Schmidt, Y. Wang, S. Younes and W. Zhang, Angew. Chem., Int. Ed., 2018, 57, 9238–9261 CrossRef CAS PubMed
;
(b) D. Holtmann and F. Hollmann, ChemBioChem, 2016, 17, 1391–1398 CrossRef CAS PubMed
. -
(a) E. Romero, J. R. Gómez Castellanos, G. Gadda, M. W. Fraaije and A. Mattevi, Chem. Rev., 2018, 118(4), 1742–1769 CrossRef CAS PubMed
;
(b) C. T. Walsh and T. A. Wencewicz, Nat. Prod. Rep., 2013, 30, 175–200 RSC
. -
(a) F. G. Gelalcha, Chem. Rev., 2007, 107, 3338–3361 CrossRef CAS PubMed
;
(b) S. Ghisla and V. Massey, Eur. J. Biochem., 1989, 181, 1–17 CrossRef CAS PubMed
. -
(a) T. Sakai, T. Kumoi, T. Ishikawa, T. Nitta and H. Iida, Org. Biomol. Chem., 2018, 16, 3999–4007 RSC
;
(b) R. Cibulka, Eur. J. Org. Chem., 2015, 5, 915–932 CrossRef
;
(c) G. de Gonzalo and M. W. Fraaije, ChemCatChem, 2013, 5, 403–415 CrossRef CAS
. -
(a) T. Ishikawa, M. Kimura, T. Kumoi and H. Iida, ACS Catal., 2017, 7(8), 4986–4989 CrossRef CAS
;
(b) H. Iida, T. Ishikawa, K. Nomura and S. I. Murahashi, Tetrahedron Lett., 2016, 57, 4488–4491 CrossRef CAS
;
(c) S. Murahashi, D. Zhang, H. Iida, T. Miyawaki, M. Uenaka, K. Murano and K. Meguro, Chem. Commun., 2014, 50, 10295–10298 RSC
;
(d) Y. Imada, I. Tonomura, N. Komiya and T. Naota, Synlett, 2013, 24, 1679–1682 CrossRef CAS
;
(e) Y. Imada, Y. Kugimiya, S. Iwata, N. Komiya and T. Naota, Tetrahedron, 2013, 69, 8572–8578 CrossRef CAS
;
(f) S. Chen, M. S. Hossain and F. W. Foss, ACS Sustainable Chem. Eng., 2013, 1, 1045–1051 CrossRef CAS
;
(g) A. T. Murray, P. Matton, N. W. G. Fairhurst, M. P. John and D. R. Carbery, Org. Lett., 2012, 14, 3656–3659 CrossRef CAS PubMed
;
(h) P. Meńova and R. Cibulka, J. Mol. Catal. A: Chem., 2012, 363–364, 362–370 CrossRef
;
(i) S. Chen and F. W. Foss, Org. Lett., 2012, 14, 5150–5153 CrossRef CAS PubMed
;
(j) Y. Imada, H. Iida, S. Ono and S. I. Murahashi, J. Am. Chem. Soc., 2003, 125, 2868–2869 CrossRef CAS PubMed
. -
(a) A. T. Murray, M. J. Dowley, F. Pradaux-Caggiano, A. Baldansuren, A. J. Fielding, F. Tuna, C. H. Hendon, A. Walsh, G. C. Lloyd-Jones, M. P. John and D. R. Carbery, Angew. Chem., Int. Ed., 2015, 54, 8997–9000 CrossRef CAS
;
(b) B. J. Marsh, E. L. Heath and D. R. Carbery, Chem. Commun., 2011, 47, 280–282 RSC
;
(c) B. J. Marsh and D. R. Carbery, Tetrahedron Lett., 2010, 51, 2362–2365 CrossRef CAS
;
(d) J. Žurek, R. Cibulka, H. Dvořákova and J. Svoboda, Tetrahedron Lett., 2010, 51, 1083–1086 CrossRef
;
(e) W. S. Li, N. Zhang and L. M. Sayre, Tetrahedron, 2001, 57, 4507–4522 CrossRef CAS
. - H. Iida, Y. Imada and S. I. Murahashi, Org. Biomol. Chem., 2015, 13, 7599–7613 RSC
. -
(a) B. Mühldorf and R. Wolf, ChemCatChem, 2017, 9(6), 920–923 CrossRef
;
(b) B. Mühldorf and R. Wolf, Angew. Chem., Int. Ed., 2016, 55, 427–430 CrossRef PubMed
;
(c) R. Lechner, S. Kummel and B. König, Photochem. Photobiol. Sci., 2010, 9, 1367–1377 CrossRef CAS PubMed
. - B. König, P. Mario, R. K. Roland, S. Jürgen and D. Jörg, Eur. J. Org. Chem., 2001, 2297–2303 CrossRef
. - T. Tagami, Y. Arakawa, K. Minagawa and Y. Imada, Org. Lett., 2019, 21(17), 6978–6982 CrossRef CAS PubMed
.
|
This journal is © The Royal Society of Chemistry 2021 |