DOI:
10.1039/D1RA00657F
(Paper)
RSC Adv., 2021,
11, 19158-19168
Characterization of structural, functional and antioxidant properties and amino acid composition of pepsin-derived glutelin-1 hydrolysate from walnut processing by-products
Received
25th January 2021
, Accepted 29th April 2021
First published on 26th May 2021
Abstract
Glutelin-1 of defatted walnut meal protein (DWPG-1) was modified by pepsin enzymatic hydrolysis to improve its functional properties and antioxidant activities. The amino acid composition, structural characteristics, physicochemical and functional properties as well as antioxidant activities of the hydrolysate were compared with those of unmodified DWPG-1. The analysis of X-ray diffraction patterns, surface microstructure and particle size distribution indicated that enzymatic hydrolysis changed the structures of DWPG-1. Compared with the natural unhydrolyzed protein, the hydrolysate showed better physicochemical properties, such as surface hydrophobicity, solubility, emulsifying properties, foaming properties and water absorption capacity. In addition, the hydrolysate also exhibited significantly stronger antioxidant activities than DWPG-1. In conclusion, the results of this study prove that pepsin-mediated hydrolysis of walnut glutelin-1 can effectively modify the structure, function and antioxidant activity of DWPG-1, and could be used as an effective technology to produce bioactive multifunctional hydrolysates.
1. Introduction
Walnut (Juglans regia L.) is an important economic crop in China. Currently, edible walnuts are either eaten fresh or used to produce high-grade edible oils because of their high content of unsaturated fatty acids. Walnut meal, a by-product of the oil removal process, still contains a lot of nutrients, with a protein content as high as 40–50% and rich in essential amino acids.1,2 However, as a rich source of high-quality protein, walnut meal is mainly used as a cheap fertilizer or animal feed, and is rarely used for food production. How to make full use of walnut protein resources and improve the high-value utilization of walnut protein are of great significance to the development of the walnut industry and human health.
Protein modification is a way of improving the nutritional value or functional properties of certain proteins by modifying or changing the structure of the protein molecules, and even endowing them with new biological activities. Among various protein modification technologies, protease enzymatic modification has been widely applied to improve the bioactivities and functional properties of proteins in the food industry and other related industries due to its mild reaction conditions, controllability and excellent product performance.3 Enzymatic hydrolysis can effectively improve the functional properties of proteins (such as solubility, emulsifying, foaming and water/oil absorption properties),4 molecular weight, and the number of hydrophobic groups.5,6 Furthermore, it has been reported that enzymatic hydrolysis was a safe and effective method for releasing bioactive peptides such as antioxidant peptides, antihypertensive peptides and anticancer peptides. These active peptides play important roles in improving human physiological functions and regulating metabolic pathways, and can be used as nutritional health products, functional food ingredients and food additives to promote human's health.7,8
Generally, according to the solubility of the protein, plant protein fractions are divided into water-soluble albumin, salt-soluble globulin, alcohol-soluble gliadin, acid-soluble glutelin (glutelin-1), and alkali-soluble glutelin (glutelin-2).9,10 Previous studies have shown that the relative proportions of protein components affect their physicochemical properties, nutritional value, biological activities and their roles in food or other industries.11 The storage protein in a dilute acid-soluble seed is commonly known as glutelin-1 protein.9 Glutelin-1 is the main ingredient of walnut protein (accounting for 21 g/100 g). Although there have been some reports on enzymatic modification of improving the functional properties of walnut protein or alkaline glutelin,12,13 there are few reports on the modification of walnut acidic glutelin (glutelin-1) by enzymatic hydrolysis to enhance its functional and antioxidant properties. The food processing system is relatively complex, and the functional properties of protein are not only related to its amino acid composition, molecular size, surface microstructure and other inherent physical properties, but also related to factors such as ionic strength, temperature, and pH in the processing environment. Therefore, it is necessary to analyze the influence of factors such as pH and ionic strength on the functional properties of walnut glutelin-1. However, there are few researches reports in this area at present. Therefore, this research attempts to explore the modification of walnut glutelin-1 by pepsin-mediated hydrolysis to improve its functional and antioxidant properties. The relationship between structure–function and enzymatic hydrolysis was also needed to be further explored. The purpose of this study is to improve the high-value utilization of defatted walnut meal protein, and provide theoretical basis and guidance for high-quality deep processing in the food industry.
2. Materials and methods
2.1. Materials
Defatted walnut meal was obtained from the Plant Oil and Protein Engineering Laboratory of Shaanxi Normal University, Xi'an city of Shaanxi province, China. Pepsin (3 × 103 U g−1) were purchased from Beijing Solarbio Science & Technology Co., Ltd. (China). All chemicals and reagents were of analytical grade.
2.2. Preparation of glutelin-1 in degreased walnut meal (DWPG-1)
The glutelin-1 fractions was fractionated by employing in the method used in Zheng et al., (2017) with some modifications.14 Walnut meal was defatted by stirring in n-hexane (1
:
10, g mL−1) for 2 h. This procedure was repeated three times, then dried, ground and passed through a 0.2 mm mesh sieve. The continuous extraction method sequentially used deionized water, 0.4 M NaCl, 70% (v/v) ethanol, 50% (v/v) glacial acetic acid and 0.1 M NaOH to extract albumin, globulin, prolamin, glutelin-1 and glutelin-2 from the defatted walnut meal. Each fraction was extracted by stirring at 4 °C for 4 h at a meal to solvent ratio of 1
:
10 (g mL−1), and this step was repeated three times. The supernatants for each solvent were combined separately and then centrifuged at 12
000g for 30 min. The supernatant was collected, dialyzed against distilled water for 24 h, and then lyophilized. Defatted walnut glutelin-1 was obtained and stored at −30 °C.
2.3. Preparation of DWPG-1 hydrolysate (DWPG-1H)
Based on the author's research on the enzymatic hydrolysis process of walnut glutelin-1, due to the higher degree of hydrolysis and stronger biological activity, pepsin was a suitable protease screened out. The ratio of enzyme to substrate (g/100 g protein) was 4%. Walnut glutelin-1 solution was adjusted to pH 1.65 with 0.1 M HCl and incubated at 45 °C for about 10 min, and then the solution was subjected to hydrolyze at 45 °C for 6 h. During the reaction, the pH of the mixture was maintained at the desired value by the continuous addition of 0.1 M HCl or 0.1 M NaOH. The enzymatic hydrolysis was stopped by heating the mixtures in a 95 °C water bath for 10 min. The hydrolysate was centrifuged at 10
000 × g at 4 °C for 20 min, supernatant was collected, freeze-dried, and stored at −30 °C for further analysis.
2.4. Amino acid composition analysis
According to the method used in Zhao et al.,15 glutelin-1 and its enzymatic hydrolysate were hydrolyzed at 110–115 °C with 6 M HCl for 24 h in an inert environment. The amino acid composition of the hydrolysate other than tryptophan was measured by an automatic amino acid analyser (L-8800, Hitachi, Japan). The amino acid composition was expressed as g/100 g protein.
2.5. Structural properties
2.5.1. Surface microstructure. The surface microstructures of glutelin-1 and its enzymatic hydrolysate were analyzed by using S-3000 N scanning electron microscope. A 10 mg protein sample was dispersed in water, then the solution was dropped onto a silicon dioxide sheet and dried. After spraying gold powder, the silicon dioxide sheet was subjected to SEM observation using a S-3000 N scanning electron microscope (SEM) at 10 kV voltage. The magnification was 1200×.
2.5.2. Particle size distribution. Disperse 0.1 gram of glutelin-1 and its enzymatic hydrolysate in 10 mL distilled water, and centrifuged at 4000 rpm for 5 min. The supernatant was taken and observed with a laser particle size analyzer at 25 °C and 659 nm. In addition to measure the particle size distribution of the supernatant, we also measured the particle size distribution of glutelin-1. Due to the poor solubility of glutelin-1, we shook it well and measured its particle size distribution under the same conditions (BI-90plus laser particle size instrument, Brookhaven Instruments, USA).
2.5.3. X-ray diffraction. X-ray diffraction (XRD) was carried out to analyze the crystalline structure of glutelin-1 and its enzymatic hydrolysate. After dried at 45 °C for 2 h, each sample was grounded into powder, and then was scanned from 10° to 60° at a step size of 0.02° and a time interval of 0.5 s per step. Furthermore, the XRD patterns were obtained by using a diffractometer (D8 Advance powder X-ray diffraction instrument, Germany) with copper Kα radiation.
2.5.4. Thermal analyses (TG/DTG). The thermal properties of glutelin-1 and its hydrolysate were analyzed via a thermal analysis system (Q600SDT, TA Instrument, USA). Approximately 10 mg in each sample was placed into an aluminum pan, and a sealed empty aluminum pan was used as the control. Under a pure nitrogen atmosphere with a flow rate was 1.0 mL min−1, the sample pans were heated from room temperature (25 °C) to 600 °C at a constant heating rate of 10 °C min−1.
2.5.5. Surface hydrophobicity. Surface hydrophobicity of glutelin-1 and its enzymatic hydrolysate were determined according to the method described by Zheng et al.,16 with some modifications. A series of protein suspensions (0.1–0.5 mg mL−1) and 8.0 mM 8-anilino-1-naphthalenesulfonic acid (ANS) solutions were prepared with the same phosphate buffer (0.01 M, pH 7.0). We added 20 μL ANS solution to 2 mL of protein solution and mixed well. Their fluorescence intensity (FI) was measured via fluorescence spectrophotometer (970CRT) with a slit width of 5 nm, and the emission and excitation wavelengths were 470 nm and 390 nm respectively. FI values were plotted against protein concentrations, and the S0 value was determined from the initial slope of the regression curve. The walnut glutelin-1 and its hydrolysate without ANS were used as controls.
2.6. Physicochemical and functional properties
2.6.1. Solubility. Solubility of glutelin-1 and its enzymatic hydrolysate at different pH according to the method of Zheng et al.16 Disperse 1 gram of samples in 100 mL distilled water, adjust to pH 1–11 with 0.1 mol L−1 NaOH or 0.1 mol L−1 HCl. After stirring for at 30 °C for 30 minutes, the mixture was centrifuged at 8000g for 20 min. The supernatant protein content and the total protein content of samples was determined by the Bradford method (1976) and the micro Kjeldahl procedure respectively. The solubility of protein was calculated by the following equation:
Protein solubility = (the supernatant protein content/the total protein content) × 100% |
2.6.2. Emulsifying properties. Emulsifying activity index (EAI) and emulsion stability index (ESI) of glutelin-1 and its enzymatic hydrolysate were investigated according to the method of Zheng et al.16 with slight modifications. 4 mL sample solution was homogenized with 2 mL of soybean oil at a speed of 15
000 rpm for 2 min, and then 100 μL of the mixture was pipetted from the bottom of the container and mixed with 4.9 mL of SDS solution (1 mg mL−1). The spectrophotometer was used to measure the instant absorbance value (A0) at the time of emulsion formation at 500 nm and the absorbance value (At) after 20 minutes of emulsion formation. Furthermore, the effects of ionic strength (NaCl concentrations of 0.1, 0.2, 0.4, and 0.6 M, respectively) and pH values (pH 1.0, 3.0, 5.0, 7.0 and 9.0) on emulsion emulsification and stability were also evaluated.EAI (m−2 g−1) and ESI (%) were calculated by the following equation:
EAI (m2 g−1) = (2.303 × 2 × A0 × df)/(C × Φ × 10 000) |
C (protein concentration) = 0.001 g mL
−1;
Φ = oil volume fraction for emulsion (0.25);
df (dilution factor) = 50.
2.6.3. Foaming properties. The foaming capacity (FC) and foam stability (FS) of glutelin-1 and its enzymatic hydrolysate at different ionic strengths were adjusted by NaCl (ionic strengths 0.1, 0.2, 0.4 and 0.6 M) and different pH values (pH 1.0, 3.0, 5.0, 7.0, 9.0 and 11.0) were evaluated according to the method described by Zheng et al.16 An aliquots (30 mL) of different sample suspensions (0.5 g L−1) were homogenized at a rate of 12
000 rpm for 3 min, and the volumes of the homogenized emulsion at 0 (V0) and 30 (Vt) minutes were recorded. FC and FS were calculated with the following equations:
2.6.4. Water absorption capacity (WAC) and oil absorption capacity (OAC). WAC and OAC of glutelin-1 and its enzymatic hydrolysate were measured following the method of Zheng et al.16 The effects of different ionic strengths on WAC and OAC were investigated. In brief, 0.5 g sample was added into 10 mL distilled H2O (or 10 mL soybean oil) and stir well. The mixture was kept at room temperature for 30 minutes and then centrifuged at 5000g for 30 minutes. The water or oil were then decanted and their volume Vs were recorded. The calculation formula of WAC and OAC was as follows:
WAC (OAC) = (10 mL − Vs)/0.5 g |
2.6.5. Rheological properties. The rheological properties of different concentrations (0.02, 0.04, 0.06 and 0.08 wt%) glutelin-1 and its enzymatic hydrolysate were measured by a controlled-strain rheometer (AR-G2, TA Instruments, USA). In short, 2 mL of the sample was loaded on the sample stage, and the continuous shear test was maintained at 25 °C with a shear rate range of 0.1–100 s−1 to test the apparent shear viscosity of the sample.
2.7. Antioxidant properties
2.7.1. DPPH radical scavenging activity. The scavenging activity of 2,2-diphenyl-1-picrylhydrazyl (DPPH) radical of glutelin-1 and its enzymatic hydrolysate were investigated according to the method of X. Li et al.17 with some modifications. 1 mL of each sample at different concentrations (0.1–0.5 mg mL−1) was mixed with 4 mL of 0.1 mM DPPH, shaken vigorously, and then reacted in the dark at room temperature for 60 min, the absorbance was measured at 517 nm. BHT (0.1–0.5 mg mL−1) and GSH (0.1–0.5 mg mL−1) were used as a comparison. The scavenging activity was expressed as follows:
DPPH scavenging-activity (%) = [1 − (AS − AB)/AC] × 100 |
In which AB was the absorbance of the blank, AC was the absorbance of control, AS was the absorbance of protein samples at different concentration.
2.7.2. Hydroxyl radical (˙OH) scavenging activity. The hydroxyl radical-scavenging activity of glutelin-1 and its enzymatic hydrolysate were measured according to the method described by Zheng et al.16 Briefly, 1 mL of each sample solution at different concentrations (0.1–0.5 mg mL−1), 2 mL of 6 mmol FeSO4, and 2 mL of hydrogen peroxide (H2O2, 6 mmol) were mixed and incubated at 37 °C for 1 h. After that, the reaction solution was mixed with 2 mL ortho-hydroxybenzoic acid (6 mmol) and incubated for 30 min, and then the absorbance was measured at 510 nm, with BHT (0.1–0.5 mg mL−1) and GSH (0.1–0.5 mg mL−1) as controls. The activity was expressed as follows:
˙OH scavenging activity (%) = [1 − (AS − AB)/AC] × 100 |
In which AB was the absorbance of the blank, AC was the absorbance of control, and AS was the absorbance of protein samples at different concentration.
2.7.3. Reducing power. Reducing power was determined according to the method of Li et al.17 with minor modifications. 500 μL aliquot of at different concentrations (0.1–0.5 mg mL−1) was mixed with 1.25 mL of 0.2 M phosphate buffer (pH 6.6) and 1.25 mL potassium ferricyanide (10 mg mL−1, 0.1%). After the mixture was incubated at 50 °C for 20 min, an aliquot (2.5 mL) of TCA (100 mg mL−1) was added, mixed by shaking, and centrifuged at 4000 rpm for 10 min. 2.5 mL of supernatant, 2.5 mL of distilled water and 2.5 mL of ferric chloride (1 μ/100 mL) were mixed and reacted at room temperature for 10 min. The absorbance of the mixture was recorded at 700 nm. A control without a sample and a blank without ferric chloride were prepared in the same way. Increased absorbance of the reaction mixture indicated an increase in the reducing power. BHT (0.1–0.5 mg mL−1) and GSH (0.1–0.5 mg mL−1) were used as controls.
2.7.4. Metal chelating activity. Metal chelating activity was measured according to the method of Zheng, Li, & Zhang, (2017) with slight modifications.14 450 μL of each protein solution at different concentrations (0.05–0.25 mg mL−1) was fully mixed with 50 μL of 2 mM FeCl2 and 2.8 mL of distilled water. After vigorous shaking, the mixture was reacted with 150 μL of 2 mmol L−1 ferrozine at room temperature for 10 min. Then the absorbance value was measured at 562 nm. BHT (0.05–0.25 mg mL−1) and GSH (0.05–0.25 mg mL−1) were used as controls.
Chelating activity (%) = [1 − (AS − AB)/AC] × 100% |
In which AB was the absorbance of the blank samples, AC was the absorbance of control samples solution, and AS was the absorbance of protein samples at different concentrations.
2.8. Statistical analysis
All the measurements were performed in triplicate and the results were expressed as mean ± SD. Differences between groups were analyzed with one-way analysis of variance (ANOVA). Statistical analysis and plots were conducted using GraphPad Prism 6.0 (La Jolla, CA, USA) and P < 0.01 was considered as extremely significant.
3. Results and discussion
3.1. Amino acid composition
The amino acid composition (g/100 g protein) of DWPG-1 and DWPG-1H were shown in Table 1. The amino acid composition of DWPG-1H was similar to that of DWPG-1, but slightly different. Glu, Pro, Arg, Asp, Phe, Ser and Leu were the most abundant amino acids. Compared to glutelin-1, DWPG-1H showed relatively higher total amino acids and total essential amino acids. The total hydrophobic amino acids of DWPG-1H was higher than that of DWPG-1, which indicated that hydrolysis increased the content of hydrophobic amino acids. The composition and sequence of amino acids were considered to have important effects on the antioxidant activity of protein.17 It was reported that some amino acids such as Asp, Glu, Pro, Arg, His, Met, Leu and Val have contribute to strong antioxidant activities.15 The increased antioxidant activities of protein after enzymatic hydrolysis may be related to the cleavage of the polypeptide chain, changes in molecular size and structure, the formation of more small peptides, and the increase in amino acid sites.
Table 1 Amino acid composition (g/100 g protein) of walnut glutelin-1 and its hydrolysatea
Amino acid |
DWPG-1 |
DWPG-1H |
|
DWPG-1 |
DWPG-1H |
Note: TAAs, total amino acids; total essential amino acids (TEAAs) = Thr + Val + Met + Phe + Ile + Leu + Lys; total hydrophobic amino acids (THAAs) = Ala + Tyr + Val + Phe + Ile + Leu + Pro + Met. |
Essential amino acid |
|
|
Nonessential amino acid |
|
|
Threonine |
2.00 ± 0.11 |
2.24 ± 0.14 |
Arginine |
9.22 ± 0.21 |
9.25 ± 0.29 |
Valine |
1.30 ± 0.03 |
1.40 ± 0.05 |
Histidine |
1.43 ± 0.08 |
1.44 ± 0.11 |
Lysine |
1.36 ± 0.08 |
1.37 ± 0.10 |
Aspartic acid |
8.52 ± 0.24 |
9.12 ± 0.31 |
Methionine |
2.59 ± 0.13 |
2.38 ± 0.08 |
Serine |
4.05 ± 0.26 |
4.17 ± 0.24 |
Isoleucine |
1.17 ± 0.14 |
1.22 ± 0.13 |
Glutamic acid |
18.32 ± 0.29 |
18.37 ± 0.45 |
Leucine |
4.23 ± 0.26 |
4.63 ± 0.23 |
Tyrosine |
1.93 ± 0.13 |
1.92 ± 0.12 |
Phenylalanine |
4.82 ± 0.19 |
5.40 ± 0.17 |
Glycine |
3.32 ± 0.22 |
3.26 ± 0.15 |
Tryptophan |
ND |
ND |
Alanine |
4.19 ± 0.08 |
4.28 ± 0.12 |
|
|
|
Proline |
13.86 ± 0.27 |
14.14 ± 0.35 |
TAAs/(g/100 g) |
82.31 ± 2.72 |
84.59 ± 3.04 |
|
|
|
TEAAs/(g/100 g) |
17.47 ± 0.94 |
18.64 ± 0.90 |
|
|
|
THAAs/(g/100 g) |
34.09 ± 1.23 |
35.37 ± 1.25 |
|
|
|
3.2. Structural properties
3.2.1. Scanning electron microscopy. Walnut glutelin-1 before and after the modification had obvious differences in SEM images (Fig. 1A). The SEM images exhibited that the DWPG-1 tended to show a relatively loose large heterogeneous flaky plate like structure, while DWPG-1H was completely broken into a compact flaky structure. By analyzing the microstructure before and after modification, it can be concluded that the modification of enzymatic treatment had changed the microstructure of walnut glutelin-1. This might be because enzymatic modification accelerated the destruction of spatial structure and promoted the degradation of the peptide bond in the polypeptide chain. In addition, surface morphology analysis is commonly used to establish the relationships between protein structure and functional properties, and changes in protein surface morphology may affect its functional properties.13,18 Therefore, the differences in microstructure of walnut glutelin-1 and its enzymatic hydrolysate may be one of reasons that affect their physiochemical and functional properties.
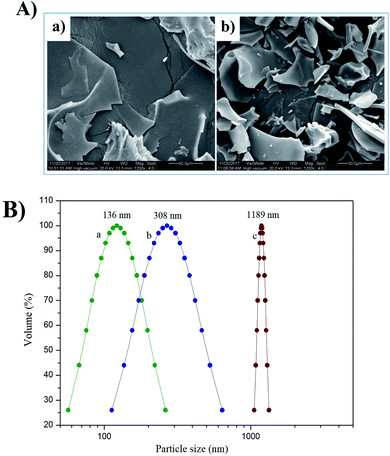 |
| Fig. 1 Scanning electron microscopy (SEM) (1200×) (A) of DWPG-1 (a) and DWPG-1H (b). Particle size distribution (B) of DWPG-1H (a); the supernatant of DWPG-1 (b); DWPG-1 (c). | |
3.2.2. Particle size distribution. The particle size distribution in the solution is used to study the aggregation, dispersion, and precipitation formation of protein particles in the solution. The particle size distribution of the samples was shown in Fig. 1B. The average particle size of DWPG-1H was 136.0 nm, the average particle size of the supernatant of walnut glutelin-1 was 308.5 nm, while the average particle size of DWPG-1 was 1189.0 nm. The grain size of DWPG-1H was mainly concentrated at 56.8–262.2 nm, the supernatant of walnut glutelin-1 was mostly at 112.7–639.5 nm, and DWPG-1 was mostly at 1056.7–1333.1 nm. This reason might be that enzymolysis decreased the molecular weight of the protein and altered the multi-level structure of glutelin-1 and decreased the intertwining of polypeptide chains.13,19
3.2.3. X-ray diffraction. As shown in Fig. 2A, DWPG-1 had a prominent diffraction peak at 2θ = 20°, while DWPG-1H showed two fairly broad diffraction peaks, which indicated that the hydrolysate was amorphous samples. Moreover, the crystallinity values of DWPG-1 were much higher than those of DWPG-1H, given that enzymatic hydrolysis might lead to disruption of the crystal structure of glutelin-1 and the decline of the crystallinity value, turning oriented macromolecules into small molecules, which was well consistent with the results of SEM images and particle size distribution (Fig. 1A and B).
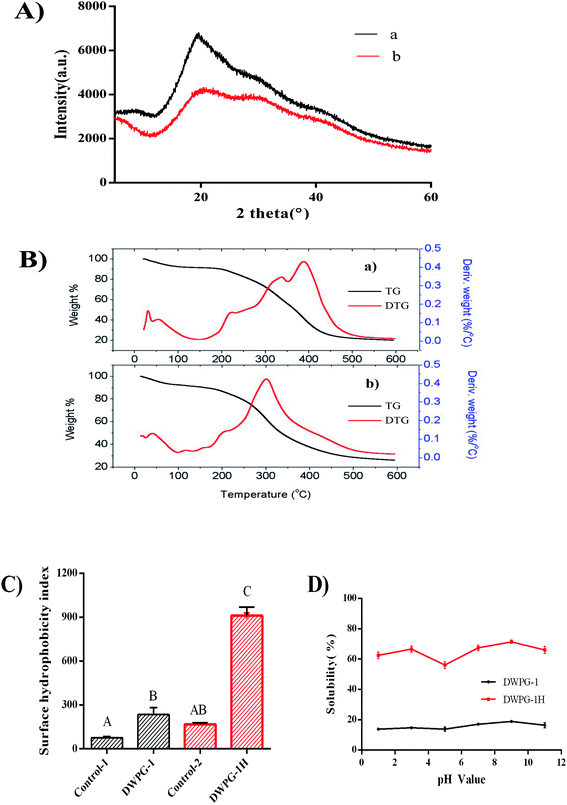 |
| Fig. 2 X-ray diffraction (XRD) patterns (A), thermal analyses (TG/DTG) (B), surface hydrophobicity (C) solubility (D) of DWPG-1 (a) and DWPG-1H (b). Control-1: the group of DWPG-1without ANS. Control-2: the group of DWPG-1H without ANS. Different letters indicate extremely significant differences in the same group (p < 0.01). | |
3.2.4. Thermal analyses (TG/DTG). The thermal degradation behavior of both DWPG-1 and DWPG-1H were analyzed by thermogravimetry (TG) and derivative thermogravimetry (DTG), and the results were shown in Fig. 2B. The TG curves illustrated the relationships between the weight loss and the temperature of thermal degradation, while the DTG curves represent the rate of weight loss. The thermal degradation profile can be characterized by three main stages. In the initial temperature range, the weight loss of the sample was minimal, which was possibly related to dehydration.20 The weight of the sample was severely lost in the second stage (200–450 °C) at a large degree, which may be due to the thermal devolatilization of protein components.21 In the third degradation stage (450–600 °C), the TG curves did not change significantly, which indicated that the weight loss was possibly due to the decomposition of solid residues.22 These changes indicated that most of the molecules in the sample had degraded and the residual weight of the sample had stabilized. According to the DTG curves, the temperature of maximum mass-loss rates for DWPG-1 and DWPG-1H were observed at 300–450 °C and 250–350 °C, respectively. DTG curve showed that the number of peaks of DWPG-1H was significantly less than that of DWPG-1, and appeared earlier. Changes in peak temperature are thought to be on account of changes in thermal stability.23 The above differences in thermal behaviors revealed that enzymatic hydrolysate was more reactive and less stable than untreated glutelin-1.24
3.2.5. Surface hydrophobicity. Increased surface hydrophobicity (S0) is an indicator of negligible aggregation, high solubility, and potentially exposed hydrophobic components that otherwise are embedded in the globular structure of the protein due to denaturation.25 Hydrophobic groups play important roles in the function properties of protein and other biological macromolecules. Therefore, the trend of the S0 changes is an important index for the study of protein modification. The differences of S0 values of DWPG-1 and DWPG-1H were shown in Fig. 2C. S0 value of the DWPG-1H was extremely significant (P < 0.01) higher than that of DWPG-1, which indicated that pepsin-derived enzymatic hydrolysis resulted in the release of more hydrophobic groups of glutelin-1. The possible reason was that enzymatic hydrolysis altered the structural rearrangement of protein molecule.26 Furthermore, proteolysis also promoted partial unfolding of globular proteins, exposing some of the non-polar groups folded originally inside the intact protein molecule to the surrounding aqueous phase, thereby increasing their surface activity.27 Studies have shown that surface hydrophobicity is an important factor affecting the solubility of protein hydrolysates.28 However, it is found that the effect of surface hydrophobicity on solubility is not as important as the size and charged groups of small peptides produced during hydrolysis.27 This might be the reason why DWPG-1H showed higher hydrophobicity but still exhibited good solubility (Fig. 2D).
3.3. Physicochemical and functional properties
3.3.1. Solubility. High water solubility plays a key role in many proteins aimed for use in the food industry, because solubility affects functional properties such as emulsification, water absorption capacity, rheological properties, and gelation. The solubility of DWPG-1 before and after modification were presented in Fig. 2D. The results showed that solubility of untreated walnut glutelin-1 was very low, and enzymatic modification significantly improved the solubility. The marked improvement in solubility of DWPG-1 may be attributed to the release of soluble peptides from insoluble aggregates or precipitates and more exposed ionizable amino and carboxyl groups obtained by enzymatic hydrolysis.29
3.3.2. Emulsifying properties. The EAI and ESI of DWPG-1 before and after modification were determined, and the results were shown in Fig. 3A–D. The emulsifying properties of the hydrolysate were remarkably higher than those of the unmodified walnut glutelin-1. This indicated that the enzymatic modification improved the emulsifying properties of DWPG-1. Previous studies had pointed out that hydrolysis leaded to changes in the molecular structure of proteins, and enhanced emulsifying capacity by promoting absorption at the oil–water interface, and improved the accessibility of hydrophobic groups which generally drives the initial anchoring of proteins on the oil side of the interface.30 Additionally, small molecule peptides can quickly diffuse to the oil–water interface and have a stronger emulsion formation ability than large molecule proteins.26 The emulsification performance of walnut glutelin-1 was obviously improved, on one hand, it may be related to the increased exposure of hydrophobic amino acids after enzymatic hydrolysis (Fig. 2C), on the other hand, it may also be related to the decrease of particle size of glutelin-1 by enzymatic modification (Fig. 1B).
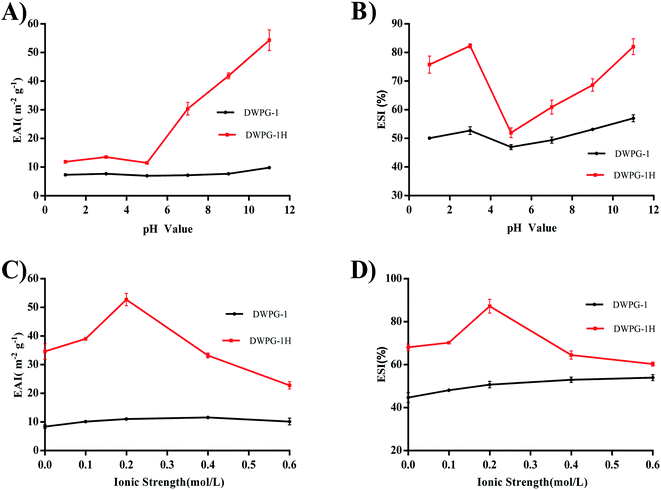 |
| Fig. 3 Emulsifying activity index (EAI) and emulsion stability index (ESI) of DWPG-1 and DWPG-1H at different pH (A and B); ionic strength (C and D). | |
The results also indicated that the ESI and EAI values of DWPG-1 and DWPG-1H changed with the variation of pH value. The minimum EAI and ESI values appeared at pH 5.0. This might due to the closeness between the pH value 5.0 and the isoelectric point (pI) of DWPG-1. Alkaline pH could improve the emulsion capacity more than acidic pH, for the negative charge expansion of peptides in the alkaline environment enhanced the emulsifying performance of DWPG-1H.31 Another factor affecting the emulsifying properties of proteins was the presence of salts. The ESI and EAI of DWPG-1 and DWPG-1H both increased in low concentration salt solution and decreased in high concentration salt solution. This might be due to the promotion of “salting-in” in low concentration salt solution, and “salting-out” occurred in high concentration salt solution.16
3.3.3. Foaming properties. The results of FC and FS showed that the modified walnut glutelin-1 exhibited excellent foaming properties, indicating that the hydrolysate of glutelin-1 had a more flexible protein structure in aqueous solutions and a stronger interaction at the air–water interface to form a more stable foam (Fig. 4A–D). Studies have reported that high surface hydrophobicity was considered as an important factor to promote protein foaming properties, the native proteins can neither diffuse nor adsorb at the air–water interface due to lacking of hydrophobic groups.30 Compared with DWPG-1, the hydrolysate exposed more hydrophobic groups from the inner spatial structure (Fig. 2C), from which it can be inferred that the hydrolysate molecular diffusing or migrating to the air–water interface was more quickly to form more stable foams and lowered the surface tension.32
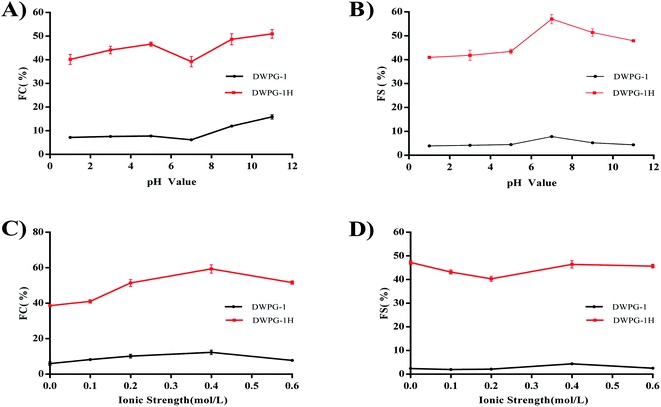 |
| Fig. 4 The foaming capacity (FC) and foam stability (FS) of DWPG-1 and DWPG-1H at different pH (A and B); ionic strength (C and D). | |
The foaming properties of DWPG-1 and DWPG-1H were affected by pH value. Furthermore, DWPG-1H was found to exhibit better foaming properties in both acidic and alkaline pH values. The samples exhibited higher FC at lower ionic strength (≤0.4 M) than at high ionic strength. The decrease in FC at high ionic strength maybe due to the reduced solubility of the peptides and electrostatic repulsion between the peptide molecules.16
3.3.4. Water absorption capacity and oil absorption capacity. The WAC of DWPG-1 before and after modification were presented in Fig. 5A. The hydrolysate exhibited a higher water absorption than that of unmodified protein. At low salt concentration, hydrated salt ions binding weakly to charged groups of protein did not affect the hydration shell of charged groups of the protein; at high salt concentration, a large amount of the existing water was bound to salt ions, and the interactions between protein molecules were enhanced, which leaded to protein dehydration and reduction of WAC.33
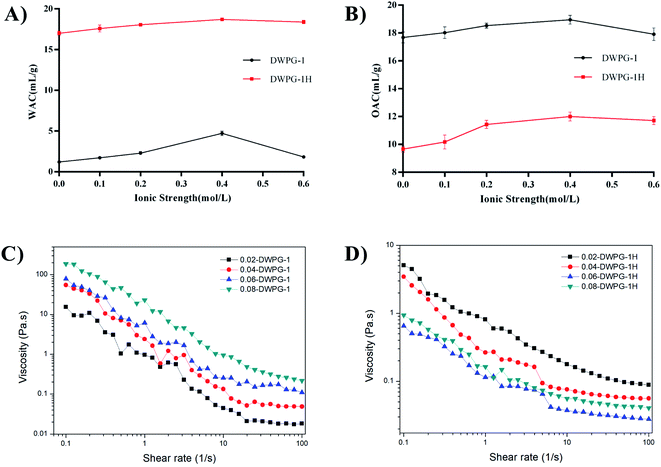 |
| Fig. 5 Water absorption capacity (WAC) and oil absorption capacity (OAC) of DWPG-1 and DWPG-1H. (A) WAC; (B) OAC. The rheological properties of different concentrations DWPG-1 (C) and DWPG-1H (D). | |
As shown in Fig. 5B, the OAC of modified walnut glutelin-1 was significantly lower than that of unmodified DWPG-1. Hydrophobic interactions and the specificity of different proteases may also contribute to changes in OAC in the protease hydrolysates.34 According to reports, the protein appears in the form of a network, and the disruption of the network would lead to a reduction in OAC. Combined with the previous analysis, we speculate that the decrease of the ability of walnut glutelin-1 to wrap or adsorb oil after enzymatic hydrolysis may be related to the destruction of its structure and the decrease of the particle size of the protein (Fig. 1B).
3.3.5. Rheological properties. The viscosity analysis of DWPG-1 before and after modification were shown in Fig. 5C and D, and the viscosities gradually decreased with increasing shear rate. Compared with unmodified walnut glutelin-1, enzymatic modification significantly reduced the apparent viscosity and became less affected by the change of protein concentration. The higher solubility of the hydrolysate made its suspension easier to flow under shear, which resulted in a relatively lower viscosity. Our findings were consistent with other reports on the association between the viscosity properties and solubility of walnut proteins (Fig. 2D).23 Additionally, the decreased apparent viscosity of the hydrolysate was mainly due to the reduction of protein–protein interactions after enzymatic hydrolysis. Research by Cheruppanpullil et al., (2008) revealed that the viscosity of soybean hydrolysate material was related to its surface microstructure. With the increase of hydrolysis degree, macromolecular proteins were degraded gradually, and the spatial structure of polypeptide chain was destroyed, forming homogeneous and compact surface structure, and the viscosity decreased.35
3.4. Antioxidant activities
The antioxidant activity of protein may be affected by enzymatic modification, depending on the sequence, molecular distribution, structure and exposure of terminal amino acid of the peptides.36,37 DPPH free radical and hydroxyl radical were widely used to evaluate the scavenging ability of antioxidants.28 The reducing ability of molecules in peptides was an important indicator of antioxidant capacity.38 Transition metal ions play important roles in catalyzing the formation of reactive oxygen species and promoting oxygen damage in cells and organs, while peptides can inhibit the negative effects of metal ions through chelation.39 Therefore, the DPPH radical, hydroxyl radical scavenging test, reducing power and metal ion chelating activity were used to evaluate the potential antioxidant activity of walnut glutelin-1 and its hydrolysate (Fig. 6A–D). The results of this study showed that radical scavenging activity, reducing power and chelating activity of walnut glutelin-1 were augmented through the enzymatic hydrolysis. It could be seen that the hydrolysate exhibited higher antioxidant activities than that of the unmodified protein. This may be due to the increased usability of hydrogen ions obtained by using smaller peptides.17 Compared with unhydrolyzed proteins, the hydrolysate had a higher ability to terminate the radical chain reactions.12 Existing research results showed that proteins with smaller molecular weight and stronger hydrophobicity tend to have stronger free radical scavenging ability. This may be due to the polypeptide chain of the protein was cleaved under the hydrolysis of enzymes, and the molecular size and structure were changed. The active regions or peptides were released, and the antioxidant activities of the protein were increased accordingly.40,41
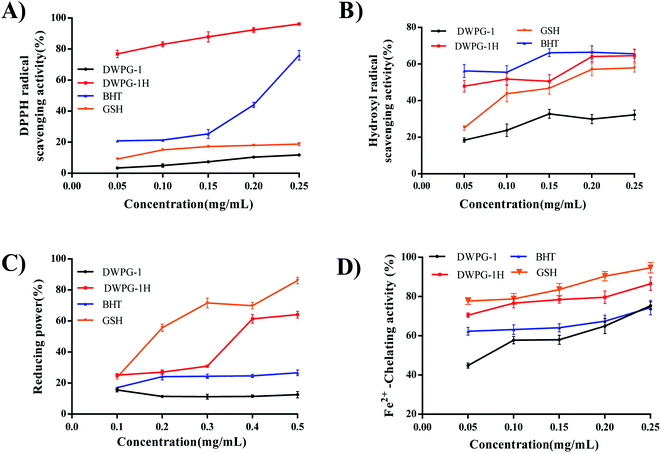 |
| Fig. 6 Antioxidant activities of DWPG-1 and DWPG-1H. (A) DPPH radical scavenging activity; (B) hydroxyl radical scavenging power; (C) reducing power; (D) Fe2+-chelating activity. BHT and GSH were used as positive controls. | |
4. Conclusions
The results of this study indicated that the pepsin-mediated hydrolysis can be used as an effective tool to improve the functional and antioxidant properties of walnut glutelin-1. The surface hydrophobicity, solubility, emulsifying and foaming properties, water absorption activity, DPPH radical scavenging activity, hydroxyl radical scavenging power, reducing power and Fe2+-chelating activity of the hydrolysate were considerably improved by enzymatic hydrolysis compared with the unmodified walnut glutelin-1. Under the action of enzymes, the polypeptide chain of the protein was cut, which has changed the size and structure of the molecule, released more biologically active regions or peptides, and improved the functional and antioxidant properties of walnut glutelin-1. In summary, this study suggested that pepsin-mediated hydrolysis can be used to produce multi-functional hydrolysate from walnut glutelin-1, which could provide a strong foundation for its later application in food products.
Conflicts of interest
There are no conflicts of interest to declare.
Acknowledgements
This work is supported by Shaanxi Provincial Department of Science and Technology [grant number: 2019NY-133, 2021NY-132], the Key Laboratory of Se-enriched Products Development and Quality Control (Ministry of Agriculture), National and Local Joint Engineering Laboratory for Selenium-enriched Food Development [grant number: Se-2019B03]. Undergraduate Innovation and Entrepreneurship Training Program of Shaanxi Xueqian Normal University [grant number: 2020DC090].
References
- K. W. C. Sze-Tao and S. K. Sathe, J. Sci. Food Agric., 2000, 80, 1393–1401 Search PubMed.
- Z. Zhu, W. Zhu, J. Yi, N. Liu, Y. Cao, J. Lu, E. A. Decker and D. J. McClements, Food Res. Int., 2018, 106, 853–861 CrossRef CAS PubMed.
- Y. Zhang, Y. Zhang, X. Liu, L. Huang, Z. Chen and J. Cheng, Food Chem., 2017, 227, 211–218 CrossRef CAS PubMed.
- Q. Li, S. Tang, F. K. Mourad, W. Zou, L. Lu and Z. Cai, Food Hydrocolloids, 2020, 101, 105521 CrossRef CAS.
- M. Galante, R. De Flaviis, V. Boeris and D. Spelzini, LWT, 2020, 118, 108845 CrossRef CAS.
- X. Zang, C. Yue, Y. Wang, M. Shao and G. Yu, J. Cereal Sci., 2019, 85, 168–174 CrossRef CAS.
- I. W. Y. Cheung and E. C. Y. Li-Chan, J. Funct. Foods, 2017, 28, 254–264 CrossRef CAS.
- R. Jahanbani, S. M. Ghaffari, M. Salami, K. Vahdati, H. Sepehri, N. N. Sarvestani, N. Sheibani and A. A. Moosavi-Movahedi, Plant Foods Hum. Nutr., 2016, 71, 402–409 CrossRef CAS PubMed.
- K. Kwon, K. Park and K. Rhee, J. Agric. Food Chem., 1996, 44, 1741–1745 CrossRef CAS.
- B. Hu and A. Esen, J. Agric. Food Chem., 1981, 29, 497–501 CrossRef CAS PubMed.
- M. Angelia, R. Garcia, K. Caldo, K. Prak, S. Utsumi and E. Tecson-Mendoza, Food Sci. Technol. Res., 2010, 16, 225–232 CrossRef CAS.
- M. Moghadam, M. Salami, M. Mohammadian, Z. Emam-Djomeh, R. Jahanbani and A. A. Moosavi-Movahedi, Food Biosci., 2020, 36, 100611 CrossRef CAS.
- Q. Sun, Z. F. Ma, H. Zhang, S. Ma and L. Kong, Int. J. Food Prop., 2019, 22, 265–279 CrossRef CAS.
- Y. Zheng, Y. Li and Y. Zhang, RSC Adv., 2017, 7, 54196–54202 RSC.
- Q. Zhao, H. Xiong, C. Selomulya, X. D. Chen, H. Zhong, S. Wang, W. Sun and Q. Zhou, Food Chem., 2012, 134, 1360–1367 CrossRef CAS PubMed.
- Y. Zheng, Y. Li, Y. Zhang, R. Zhang, Q. Zhang, Y. Zhang and S. Zhao, RSC Adv., 2015, 5, 12613–12623 RSC.
- X. Li, J. Deng, S. Shen, T. Li, M. Yuan, R. Yang and C. Ding, J. Food Sci. Technol., 2015, 52, 5681–5690 CrossRef CAS PubMed.
- X. Zhao, H. Liu, X. Zhang and H. Zhu, Int. J. Biol. Macromol., 2019, 125, 1214–1220 CrossRef CAS PubMed.
- A. M. Shi, B. Jiao, H. Z. Liu, S. Zhu, M. Jiang Shen, X. L. Feng, H. Hu, L. Liu, S. Faisal, Q. Wang and B. Adhikari, LWT, 2018, 97, 662–667 CrossRef CAS.
- M. A. Malik, H. K. Sharma and C. S. Saini, Food Hydrocolloids, 2017, 72, 312–322 CrossRef CAS.
- Y. Zhao, N. Sun, Y. Li, S. Cheng, C. Jiang and S. Lin, Food Res. Int., 2017, 100, 850–857 CrossRef CAS PubMed.
- Y. Zhang, B. Wang, W. Zhang, W. Xu and Z. Hu, J. Cereal Sci., 2019, 87, 318–324 CrossRef CAS.
- A. M. Shi, B. Jiao, H. Z. Liu, S. Zhu, M. Jiang Shen, X. L. Feng, H. Hu, L. Liu, S. Faisal, Q. Wang and B. Adhikari, LWT, 2018, 97, 662–667 CrossRef CAS.
- G. Maffei, M. P. Bracciale, A. Broggi, A. Zuorro, M. L. Santarelli and R. Lavecchia, Bioresour. Technol., 2018, 249, 592–598 CrossRef CAS PubMed.
- X. Mao and Y. Hua, Int. J. Mol. Sci., 2012, 13, 1561–1581 CrossRef CAS PubMed.
- A. Mokni Ghribi, I. Maklouf Gafsi, A. Sila, C. Blecker, S. Danthine, H. Attia, A. Bougatef and S. Besbes, Food Chem., 2015, 187, 322–330 CrossRef CAS PubMed.
- Q. Liu, B. Kong, Y. Xiong and X. Xia, Food Chem., 2010, 118, 403–410 CrossRef CAS.
- V. Klompong, S. Benjakul, D. Kantachote and F. Shahidi, Food Chem., 2007, 102, 1317–1327 CrossRef CAS.
- S. W. Yin, C. H. Tang, J. S. Cao, E. K. Hu, Q. B. Wen and X. Q. Yang, Food Chem., 2008, 106, 1004–1013 CrossRef CAS.
- A. G. B. Wouters, I. Rombouts, E. Fierens, K. Brijs and J. A. Delcour, Compr. Rev. Food Sci. Food Saf., 2016, 15, 786–800 CrossRef CAS PubMed.
- M. Ai, T. Tang, L. Zhou, Z. Ling, S. Guo and A. Jiang, Food Hydrocolloids, 2019, 87, 933–942 CrossRef CAS.
- P. Dash and G. Ghosh, J. Food Sci. Technol., 2017, 54, 4162–4172 CrossRef CAS PubMed.
- L. Samuel, K. O. Adebowale, B. M. Ogunsanwo, O. A. Sosanwo and S. Bankole, Food Chem., 2005, 92, 681–691 CrossRef.
- Z. Haque and Z. Mozaffar, Food Hydrocolloids, 1992, 5, 559–571 CrossRef CAS.
- R. Cheruppanpullil, P. Kumar and V. Prakash, Food Chem., 2008, 106, 1166–1174 CrossRef.
- H. Guan, X. Diao, F. Jiang, J. Han and B. Kong, Food Chem., 2018, 245, 89–96 CrossRef CAS PubMed.
- B. Sarmadi and A. Ismail, Peptides, 2010, 31, 1949–1956 CrossRef CAS PubMed.
- A. Shavandi, Z. Hu, S. S. Teh, J. Zhao, A. Carne, A. Bekhit and A. E. D. A. Bekhit, Food Chem., 2017, 227, 194–201 CrossRef CAS PubMed.
- Y. Li, Y. Zheng, Y. Zhang, J. Xu and G. Gao, Molecules, 2018, 23, 1–11 Search PubMed.
- M. Eisenmenger and J. Reyes-De-Corcuera, Enzyme Microb. Technol., 2009, 45, 331–347 CrossRef CAS.
- J. B. Jeong, B. de Lumen and H. Jeong, Cancer Lett., 2010, 293, 58–64 CrossRef CAS PubMed.
|
This journal is © The Royal Society of Chemistry 2021 |
Click here to see how this site uses Cookies. View our privacy policy here.