DOI:
10.1039/D1RA00251A
(Paper)
RSC Adv., 2021,
11, 8485-8490
The effect of strain on water dissociation on reduced rutile TiO2(110) surface
Received
12th January 2021
, Accepted 3rd February 2021
First published on 24th February 2021
Abstract
The effect of external uniaxial strain on water dissociation on a reduced rutile TiO2(110) surface has been theoretically studied using first-principles calculations. We find that when the tensile strain along [1
0] is applied, the energy barrier of water dissociation substantially decreases with the increase of strain. In particular, water almost automatically dissociates when the strain is larger than 3%. Besides, the water dissociation mechanism changes from indirect to direct dissociation when the compressive strain is larger than 1.3% along [1
0] or 3% along [001]. The results strongly suggest that it is feasible to engineer the water dissociation on the reduced rutile TiO2(110) surface using external strain.
1 Introduction
Titanium dioxide has attracted wide attention due to its promising applications in heterogeneous catalysis, solar cells, gas sensors, photocatalysis, environmental remediation etc.1–7 The aqueous environment is one major application area for TiO2 and the (110) surface is the most stable surface for rutile TiO2. The interaction between water and the TiO2(110) surface has been extensively studied in recent years.8–10 A bridging oxygen vacancy is one of the most common defects on the surface, and plays a critical role in surface reactions.6,9–16
Previous experimental studies showed water splitting at the bridging oxygen vacancy on the reduced TiO2(110) surface, in which hydroxyl was formed.6,12,13,17–20 Later, theoretical studies showed that water dissociation on a bridging oxygen vacancy chose an indirect pathway. Initially, a hydrogen from water transfers to a neighbor in-plane oxygen (Oip) and then hops to the neighbor bridging oxygen (Obr). The obtained barrier energies of water dissociation range from 0.16 to 0.39 eV, using different theoretical methods.13,18,20–22
External strain is an important factor which may influence molecule adsorption and dissociation, since it is unavoidable in the fabrication of nanostructures and thin films.23–27 For the TiO2 surface, experimental studies have shown that when surface strain is applied through formation of subsurface Ar-filled cavities, surface strain values are up to 4%.25 The effect of strain on hydrogen adsorption on rutile TiO2(110) has been investigated using scanning tunnelling microscopy (STM), which revealed that the adsorption energy of hydrogen on the local surface is lower than that on the protrusions.28 On the other hand, theoretical studies showed that external strain could effectively tune the type of surface oxygen vacancies, diffusion pathways and barriers of the oxygen vacancies, and even surface morphologies.29–31 The adsorption and dissociation of water on a stoichiometric rutile TiO2(110) surface under external strain has been studied, and the results clearly indicated that external strain can engineer the surface adsorption and dissociation of water.32 However, the effect of strain on water dissociation on a reduced surface has not been investigated yet.
In this paper, we systematically studied water dissociation on a reduced rutile TiO2(110) surface under external uniaxial strain. We found that when tensile strain along [1
0] is applied, the energy barrier for water dissociation substantially decreases with increasing strain. In particular, water almost automatically dissociates when the strain is larger than 3%. In contrast, the dissociation path for water changes from an indirect path to a direct path when 1.3% compressive strain along [1
0] is applied. On the other hand, the energy barrier is steady against tensile strain along [001], and the dissociation mechanism also changes from an indirect path to a direct path when 3% compressive strain along [001] is applied. The results strongly suggest that it is possible to engineer water dissociation on the reduced rutile TiO2(110) surface using external strain.
2 Computational methods
Calculations are based on density functional theory in the PW91 generalized gradient approximation,33,34 using the Vienna ab initio simulation package code with projector augmented wave pseudopotentials.35,36 An energy cutoff of 500 eV is used for expanding the Kohn–Sham wave functions in order to correctly account for the effects of surface stress. The rutile TiO2(110) surface is modeled as a (2 × 3) supercell slab with x and y along [1
0] and [001] directions, respectively. The slab contains five O–Ti–O trilayers (Ti60O120) and a vacuum with thickness of 15 Å, with a Γ-centered 1 × 3 mesh, which has been tested to be well converged. The positions of atoms in the bottom trilayer were fixed to mimic the bulk, and the other atoms were relaxed until the forces were converged to 0.01 eV Å−1. In order to model reduced TiO2(110) surfaces, a bridge oxygen is removed from one side of the slab, the oxygen vacancy density is 1/6, which is enough to obtain the influence of the oxygen vacancy on the water dissociation.13,21 The nudged elastic band (NEB) method37 is used to obtain an accurate dissociation barrier for water. A (1 × 4) supercell with a 4 × 2 k-mesh sampling is used to check the [001] direct convergence. The difference is 40 meV at maximum in the dissociation barrier energies of water in an indirect path when the [1
0] strain is applied.
In our previous work we demonstrated that spontaneous in-plane polarization does occur on the TiO2(110) surface when uniaxial tensile strain is applied, furthermore, the polarization and paraelectric surface have the same surface chemistry for water adsorption.38 Therefore, we use a paraelectric surface when compressive strain or no strain is applied, and use a polarized surface when tensile strain is applied; due to the paraelectric surface being metastable, it will spontaneously relax to the polarized structure.
3 Results and discussion
As shown in Fig. 1, water adsorption on a reduced rutile TiO2(110) surface preferentially takes place at a bridging oxygen vacancy site, the coverage (θ) of water is 1/6, which is the initial state (IS) for dissociation. Then water dissociates at the oxygen vacancy site and forms a pair of hydroxyl groups, which is the final state (FS). There are two pathways for water dissociation on the surface, which are marked by colored arrows in Fig. 1(b). In the direct path, a hydrogen from water directly jumps onto the neighboring Obr in the same row. And in the indirect pathway, the hydrogen atom first jumps onto adjacent Oip, then hops to the neighboring Obr.
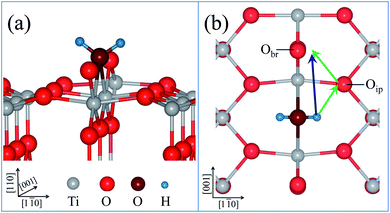 |
| Fig. 1 Configurations of water adsorption on a reduced rutile TiO2(110) surface. Stereoscopic view (a) and top view (b). Blue and green arrows to (b) represent the direct and indirect dissociation paths for water, respectively. | |
The energy profiles of water dissociation and some optimized geometrical structure of transition states are presented in Fig. 2. The transition state of the direct path is denoted as TS′, where the hydrogen is between two neighboring Obr atoms. We also locate two transition states along the indirect pathway. The first transition state is denoted as TS1, and the geometrical structure is a hydrogen atom already removed from the water molecule and close to Oip. The second transition state is denoted as TS2, the hydrogen atom leaves the Oip and moves closer to the neighbouring Obr. The energy barriers of the two paths are shown in Table 1. It is obvious that the energy barrier of the indirect pathway is lower than that of the direct path, which means that water dissociation on the reduced surface proceeds via the indirect pathway. The obtained results are in good agreement with previous results.13,18,20–22
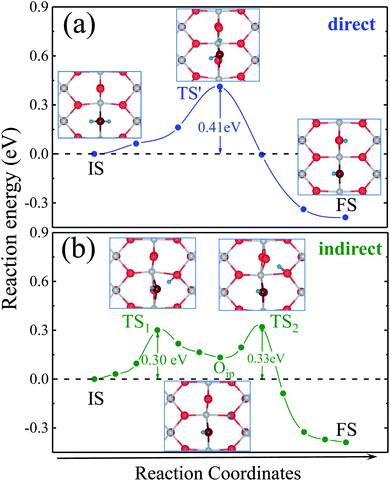 |
| Fig. 2 Energy profiles corresponding to the dissociation pathways in Fig. 1(b). The direct (a) and indirect (b) dissociation pathways. The energies are measured relative to the IS configuration. | |
The scaling relations limit the catalysis reaction, but this limitation could be broken by strain, as the binding energy is determined by the coupling of the adsorbate-induced eigenstress with the external strain.27 The energy profile of molecule dissociation can be predicted according to the surface stress profile along the dissociation pathway when external strain is applied,32 and the intrinsic surface stress can be expressed as,29
|
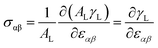 | (1) |
where
AL is the area of the surface in an unstrained state, and
γL is the Lagrangian surface energy per unit area with an unstrained state. The subscripts
α and
β denote the directions along the surface. The energy profile of molecule dissociation can be predicted by
eqn (2)where
Ei is the energy at the i
th configuration along the dissociation pathway under externally applied strain.
E0i is the energy at the i
th configuration in an unstrained state, Δ
σi is the change of surface stress between the i
th and initial state configuration with unstrained surface.
AL is the surface area occupied per water molecule when no strain is applied.
We obtained the difference of surface stress between transition states and initial state, which are shown in Table 1. It is clearly seen that the values of TS1 and TS2 along [1
0] are −0.95 and −1.10 eV/(1 × 1), which are much larger than the others, indicating that the energy barriers of TS1 and TS2 substantially decrease with increasing tensile strain along [1
0]. By substituting the data into eqn (2), the decreasing rates of TS1 and TS2 can be obtained as 0.057 and 0.066 eV per 1% tensile strain, respectively. When the tensile strain is larger than 5.6%, the dissociation barrier will disappear, which suggests that water will automatically dissociate on the reduced TiO2(110) surface when sufficient large tensile strain along [1
0] is applied. In contrast, the energy barrier of the indirect path increases with compressive strain increase, while that of the direct path changes a little for
Therefore, water dissociates through the direct path instead of the indirect pathway when compressive strain is applied along [1
0]. We also observed that the Δσ of the transition states changes little under strain along [001], which suggests that the energy barrier of dissociation varies little with strain along [001].
Table 1 Energy barrier (Eb) and the difference of the surface stress between transition states and the initial state in this work. The units are eV and eV/(1 × 1). The subscripts 11 and 22 denote the [1
0] and [001] directions, respectively
Images |
Eb |
Δσ11 |
Δσ22 |
TS′ |
0.41 |
0.18 |
0.36 |
TS1 |
0.30 |
−0.95 |
−0.09 |
TS2 |
0.32 |
−1.10 |
0.13 |
To demonstrate the predicted variation of dissociation paths under external strain, we first calculated the energy profiles under uniaxial strain in the range −4% to 4% using the nudged elastic band (NEB) method, the tensile and compressive strain are marked by positive and negative signs, respectively, as shown in Fig. 3. Firstly, we investigated the influence of strain on water dissociation on surfaces when strain is applied along [1
0]. The direct and indirect paths are shown in Fig. 3(a) and (c), respectively. It is worth noting that the energies of TS1 and TS2 along the indirect path substantially decrease simultaneously with increasing tensile strain. It is obvious that when the tensile strain is larger than 2%, the energy barrier of TS1 overtakes that of TS2 and becomes a new maximum point. As the tensile strain continues to increase to 3%, the energy barrier of the indirect dissociation path decreases to 0.02 eV. The results clearly indicate that water almost automatically dissociates on a reduced TiO2(110) surface when 3% tensile strain is applied. The energy barrier of the indirect path has a huge increase with compressive strain along the [1
0]. In contrast, the energy barrier of the direct path changes slightly.
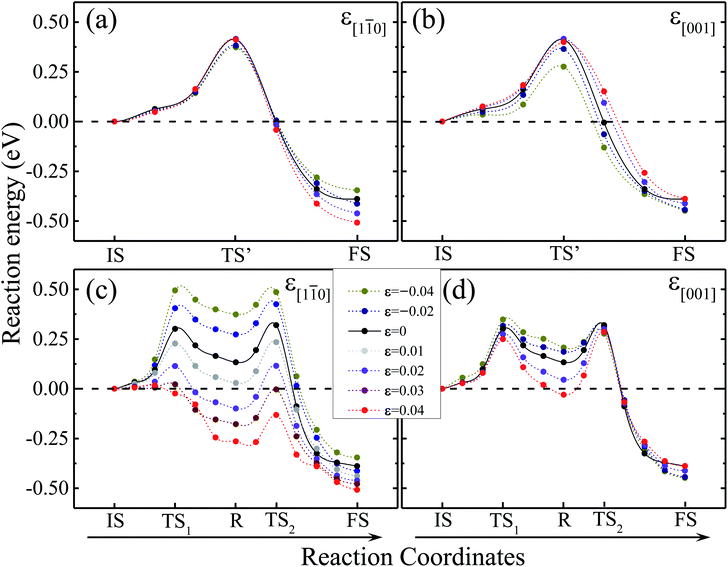 |
| Fig. 3 Variation of the energy profiles for the direct (a and b) and indirect (c and d) dissociation path under external uniaxial strain along [1 0] (a and c) and [001] (b and d). The energies are measured relative to the configuration IS. The ε = 0.01, 0.03 are presented only in (c). | |
Next we investigate the influence when strain is applied along [001]. The energy profiles of direct and indirect paths are shown in Fig. 3(b) and (d), respectively. When the strain is in the range from −4% to 4%, the energy barrier of the direct path is in the range 0.28 to 0.40 eV and the energy barrier of the indirect path is 0.28 eV to 0.35 eV. Compared with the strain along [1
0], the influence of the strain along [001] on water dissociation on a reduced TiO2 surface is weaker.
In order to get a more intuitive view of the effect of the external uniaxial strain on water dissociation on rutile reduced TiO2(110) surface, the variation of the energy barrier of each transition state along two dissociation paths are shown in Fig. 4. The solid symbols and lines denote the energy barrier of the most favorable dissociation path under the strain. Firstly, we study the influence of the strain along [1
0]. It is obvious that the energy barrier of the direct path changes a little under strain along [1
0], while that of the indirect pathway varies tremendously. It is worth noting that when the tensile strain is applied, the energy barrier of the indirect pathway is always lower than that of the direct pathway, the energy barrier of the indirect pathway substantially decreases with increasing strain. Especially, when 3% tensile strain is applied, the energy barrier is obtained as 0.02 eV, which suggests that water almost automatically dissociates on the strained surface via the indirect pathway. It is worth mentioning that when the tensile strain is larger than 2%, the TS1 becomes a new saddle point of the indirect pathway, rather than TS2. In contrast, when compressive strain is applied, the energy barrier of the indirect pathway substantially increases with increasing strain. Specifically, when the strain is larger than 1.3%, the direct pathway becomes the energetically favorable dissociation pathway due to the lower energy barrier than that of the indirect path.
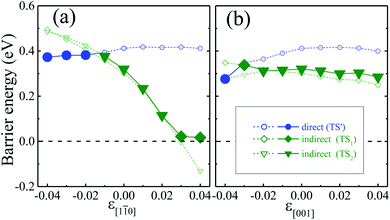 |
| Fig. 4 The dissociation energy barriers of the different pathways under an external uniaxial strain along [1 0] (a) and [001] (b). Blue and green symbols denote the direct and indirect path, respectively. Triangles and diamonds mark the TS1 and TS2 along the indirect path. Solid symbols and lines denote the energy barrier of the most favorable dissociation path on the reduced TiO2(110) under the external uniaxial strain. | |
Next we investigate the influence of strain along [001]. Obviously, the energy barriers of the two pathways change a little under tensile strain, water dissociation via the indirect pathway is the as same as the one on the nonstrained surface. In contrast, when compressive strain is applied, the energy barrier of the direct pathway decreases with increasing strain, but that of the indirect pathway increases, in particular, when 3% compressive strain along [001] is applied, the dissociation path for water changes from the indirect path to the direct path, the same as that of 1.3% compressive strain along [1
0]. By comparing the prediction and calculation results, we found that the predicted results slightly overestimate the energy barrier for the tensile strain along [1
0], which is due to the larger space for atoms to relax.
We also compare the geometries before and after the strain is applied, we found that the geometries of adsorbates rarely changed after compressive strain is applied. In contrast, when tensile strain is applied, the adsorbates on the surface get a slight deflection, the deflecting direction is along the surface polarization direction.
4 Conclusion
In summary, the effect of external uniaxial strain on water dissociation on a reduced rutile TiO2(110) surface has been theoretically studied by using first-principles calculations. We found that tensile strain along [1
0] can greatly promote the dissociation of water. In particular, water almost automatically dissociates when the strain is larger than 3%. Moreover, the dissociation path changes from an indirect path to a direct path when the compressive strain is larger than 1.3% along [1
0], or 3% along [001]. The results strongly suggest that this is a possible way to engineer water dissociation on a reduced rutile TiO2(110) surface using external strain, and the external strain also can be used to improve other chemical reactions on a reduced TiO2(110) surface.
Conflicts of interest
There are no conflicts of interest to declare.
Acknowledgements
The calculations were carried out at the High Performance Computing Center of Nanjing University and the High Performance Computing Center of the College of Physics and Electronic Engineering, Zhengzhou Normal University. This work was supported by the National Natural Science Foundation of China (grant no. 61904161), the Technologies Research and Development Program of Henan Province (grant no. 202102210201, 212102210486), the Key Young Teachers of Henan Province (grant no. 2017GGJS179), the Key Scientific Research Project of Henan College (20A140030), Science and Technology Innovation Talents in Universities of Henan Province (grant no. 18HASTIT030). The aid program for Science and Technology Innovative Research Team and the Open Research Fund of Zhengzhou Normal University.
References
- W. Li, A. Elzatahry, D. Aldhayan and D. Zhao, Core–shell structured titanium dioxide nanomaterials for solar energy utilization, Chem. Soc. Rev., 2018, 47, 8203–8237, 10.1039/C8CS00443A.
- E. J. W. Crossland, N. Noel, V. Sivaram, T. Leijtens, J. A. Alexander-Webber and H. J. Snaith, Mesoporous TiO2 single crystals delivering enhanced mobility and optoelectronic device performance, Nature, 2013, 495, 215–219, DOI:10.1038/nature11936.
- R. Asahi, T. Morikawa, T. Ohwaki, K. Aoki and Y. Taga, Visible-light photocatalysis in nitrogen-doped titanium oxides, Science, 2001, 293(5528), 269–271, DOI:10.1126/science.1061051.
- C. T. Campbell, S. C. Parker and D. E. Starr, The effect of size-dependent nanoparticle energetics on catalyst sintering, Science, 2002, 298(5594), 811–814, DOI:10.1126/science.1075094.
- M. Gratzel, Photoelectrochemical cells, Nature, 2001, 414(6861), 338–344, DOI:10.1038/35104607.
- O. Bikondoa, C. L. Pang, R. Ithnin, C. A. Muryn, H. Onishi and G. Thornton, Direct visualization of defect-mediated dissociation of water on TiO2(110), Nat. Mater., 2006, 5(3), 189–192, DOI:10.1038/nmat1592.
- G. Lu, A. Linsebigler and J. T. Yates, Photooxidation of CH3Cl on TiO2(110): a mechanism not involving H2O, J. Phys. Chem., 1995, 99(19), 7626–7631, DOI:10.1021/j100019a049.
- S. Selcuk and A. Selloni, Facet-dependent trapping and dynamics of excess electrons at anatase TiO2 surfaces and aqueous interfaces, Nat. Mater., 2016, 15(10), 1107–1112 CrossRef CAS.
- U. Diebold, The surface science of titanium dioxide, Surf. Sci. Rep., 2003, 48(5), 53–229, DOI:10.1016/S0167-5729(02)00100-0.
- M. A. Henderson, The interaction of water with solid surfaces: fundamental aspects revisited, Surf. Sci. Rep., 2002, 46(1), 1–308, DOI:10.1016/S0167-5729(01)00020-6.
- M. A. Henderson, An HREELS and TPD study of water on TiO2(110): the extent of molecular versus dissociative adsorption, Surf. Sci., 1996, 355(1), 151–166, DOI:10.1016/0039-6028(95)01357-1.
- R. Schaub, P. Thostrup, N. Lopez, E. Lægsgaard, I. Stensgaard, J. K. Nørskov and F. Besenbacher, Oxygen vacancies as active sites for water dissociation on rutile TiO2(110), Phys. Rev. Lett., 2001, 87, 266104, DOI:10.1103/PhysRevLett.87.266104.
- S. Wendt, R. Schaub, J. Matthiesen, E. Vestergaard, E. Wahlström, M. Rasmussen, P. Thostrup, L. Molina, E. Lægsgaard, I. Stensgaard, B. Hammer and F. Besenbacher, Oxygen vacancies on TiO2(110) and their interaction with H2O and O2: a combined high-resolution STM and DFT study, Surf. Sci., 2005, 598(1), 226–245, DOI:10.1016/j.susc.2005.08.041.
- Z. Zhang, Q. Ge, S. C. Li, B. D. Kay, J. M. White and Z. Dohnálek, Imaging intrinsic diffusion of bridge-bonded oxygen vacancies on TiO2(110), Phys. Rev. Lett., 2007, 99, 126105, DOI:10.1103/PhysRevLett.99.126105.
- J. Zheng, Y. Lyu, R. Wang, C. Xie, H. Zhou, S. P. Jiang and S. Wang, Crystalline TiO2 protective layer with graded oxygen defects for efficient and stable silicon-based, Nat. Commun., 2018, 9, 3572, DOI:10.1038/s41467-018-05580-z.
- S. M. Wu, X. L. Liu, X. L. Lian, G. Tian, C. Janiak, Y. X. Zhang, Y. Lu, H. Z. Yu, J. Hu, H. Wei, H. Zhao, G. G. Chang, G. Van Tendeloo, L. Y. Wang, X. Y. Yang and B. L. Su, Homojunction of oxygen and titanium vacancies and its interfacial n–p effect, Adv. Mater., 2018, 30(32), 1802173, DOI:10.1002/adma.201802173.
- I. M. Brookes, C. A. Muryn and G. Thornton, Imaging water dissociation on TiO2(110), Phys. Rev. Lett., 2001, 87, 266103, DOI:10.1103/PhysRevLett.87.266103.
- S. Wendt, J. Matthiesen, R. Schaub, E. K. Vestergaard, E. Lægsgaard, F. Besenbacher and B. Hammer, Formation and splitting of paired hydroxyl groups on reduced TiO2(110), Phys. Rev. Lett., 2006, 96, 066107, DOI:10.1103/PhysRevLett.96.066107.
- G. Ketteler, S. Yamamoto, H. Bluhm, K. Andersson, D. E. Starr, D. F. Ogletree, H. Ogasawara, A. Nilsson and M. Salmeron, The nature of water nucleation sites on TiO2(110) surfaces revealed by ambient pressure X-ray photoelectron spectroscopy, J. Phys. Chem. C, 2007, 111(23), 8278–8282, DOI:10.1021/jp068606i.
- S. C. Li, Z. Zhang, D. Sheppard, B. D. Kay, J. M. White, Y. Du, I. Lyubinetsky, G. Henkelman and Z. Dohnalek, Intrinsic diffusion of hydrogen on rutile TiO2(110), J. Am. Chem. Soc., 2008, 130(28), 9080–9088, DOI:10.1021/ja8012825.
- S. Kajita, T. Minato, H. S. Kato, M. Kawai and T. Nakayama, First-principles calculations of hydrogen diffusion on rutile TiO2(110) surfaces, J. Chem. Phys., 2007, 127(10), 104709, DOI:10.1063/1.2768951.
- J. Oviedo, R. Sánchez-de Armas, M. San Miguel and J. F. Sanz, Methanol and water dissociation on TiO2 (110): the role of surface oxygen, J. Phys. Chem. C, 2008, 112(46), 17737–17740, DOI:10.1021/jp805759y.
- M. Mavrikakis, B. Hammer and J. K. Nørskov, Effect of strain on the reactivity of metal surfaces, Phys. Rev. Lett., 1998, 81, 2819–2822, DOI:10.1103/PhysRevLett.81.2819.
- M. Lazzeri and A. Selloni, Stress-driven reconstruction of an oxide surface: The anatase TiO2(001) − (1 × 4) surface, Phys. Rev. Lett., 2001, 87, 266105, DOI:10.1103/PhysRevLett.87.266105.
- D. V. Potapenko, Z. Li, J. W. Kysar and R. M. Osgood, Nanoscale strain engineering on the surface of a bulk TiO2 crystal, Nano Lett., 2014, 14(11), 6185–6189, DOI:10.1021/nl5024194.
- D. J. Shu, F. Liu and X. G. Gong, Simple generic method for predicting the effect of strain on surface diffusion, Phys. Rev. B: Condens. Matter Mater. Phys., 2001, 64, 245410, DOI:10.1103/PhysRevB.64.245410.
- J. H. Alireza Khorshidi, J. Violet and A. A. Peterson, How strain can break the scaling relations of catalysis, Nat. Catal., 2018, 1, 263–268, DOI:10.1038/s41929-018-0054-0.
- D. V. Potapenko, G. T. Gomes and R. M. Osgood, Correlation of H adsorption energy and nanoscale elastic surface strain on rutile TiO2(110), J. Phys. Chem. C, 2016, 120(38), 21373–21380, DOI:10.1021/acs.jpcc.6b05129.
- D. J. Shu, S. T. Ge, M. Wang and N. B. Ming, Interplay between external strain and oxygen vacancies on a rutile TiO2(110) surface, Phys. Rev. Lett., 2008, 101, 116102, DOI:10.1103/PhysRevLett.101.116102.
- Z. W. Wang, D. J. Shu, M. Wang and N. B. Ming, Diffusion of oxygen vacancies on a strained rutile TiO2(110) surface, Phys. Rev. B: Condens. Matter Mater. Phys., 2010, 82, 165309, DOI:10.1103/PhysRevB.82.165309.
- L. Jia, D. J. Shu and M. Wang, Tuning the area percentage of reactive surface of TiO2 by strain engineering, Phys. Rev. Lett., 2012, 109, 156104, DOI:10.1103/PhysRevLett.109.156104.
- L. Yang, D. J. Shu, S. C. Li and M. Wang, Influence of strain on water adsorption and dissociationon
rutile TiO2(110) surface, Phys. Chem. Chem. Phys., 2016, 18, 14833–14839, 10.1039/C6CP01106C.
- J. P. Perdew, K. Burke and M. Ernzerhof, Generalized gradient approximation made simple, Phys. Rev. Lett., 1996, 77, 3865–3868, DOI:10.1103/PhysRevLett.77.3865.
- F. Allegretti, S. O’Brien, M. Polcik, D. I. Sayago and D. P. Woodruff, Adsorption bond length for H2O on TiO2(110): a key parameter for theoretical understanding, Phys. Rev. Lett., 2005, 95, 226104, DOI:10.1103/PhysRevLett.95.226104.
- G. Kresse and D. Joubert, From ultrasoft pseudopotentials to the projector augmented-wave method, Phys. Rev. B: Condens. Matter Mater. Phys., 1999, 59, 1758–1775, DOI:10.1103/PhysRevB.59.1758.
- P. E. Blöchl, Projector augmented-wave method, Phys. Rev. B: Condens. Matter Mater. Phys., 1994, 50, 17953–17979, DOI:10.1103/PhysRevB.50.17953.
- G. Mills, H. Jnsson and G. K. Schenter, Reversible work transition state theory: application to dissociative adsorption of hydrogen, Surf. Sci., 1995, 324(2), 305–337, DOI:10.1016/0039-6028(94)00731-4.
- Z. W. Wang and D. J. Shu, Intrinsic interaction between in-plane ferroelectric polarization and surface adsorption, Phys. Chem. Chem. Phys., 2019, 21, 18680–18685, 10.1039/C9CP03286J.
|
This journal is © The Royal Society of Chemistry 2021 |
Click here to see how this site uses Cookies. View our privacy policy here.