DOI:
10.1039/D0RA10560K
(Review Article)
RSC Adv., 2021,
11, 12398-12422
Ionic liquids and deep eutectic solvents for the recovery of phenolic compounds: effect of ionic liquids structure and process parameters
Received
15th December 2020
, Accepted 15th March 2021
First published on 29th March 2021
Abstract
Water pollution is a severe and challenging issue threatening the sustainable development of human civilization. Besides other pollutants, waste fluid streams contain phenolic compounds. These have an adverse effect on the human health and marine ecosystem due to their toxic, mutagenic, and carcinogenic nature. Therefore, it is necessary to remove such phenolic pollutants from waste stream fluids prior to discharging to the environment. Different methods have been proposed to remove phenolic compounds from wastewater, including extraction using ionic liquids (ILs) and deep eutectic solvent (DES), a class of organic salts having melting point below 100 °C and tunable physicochemical properties. The purpose of this review is to present the progress in utilizing ILs and DES for phenolic compound extraction from waste fluid streams. The effects of IL structural characteristics, such as anion type, cation type, alkyl chain length, and functional groups will be discussed. In addition, the impact of key process parameters such as pH, phenol concentration, phase ratio, and temperature will be also described. More importantly, several ideas for addressing the limitations of the treatment process and improving its efficiency and industrial viability will be presented. These ideas may form the basis for future studies on developing more effective IL-based processes for treating wastewaters contaminated with phenolic pollutants, to address a growing worldwide environmental problem.
1. Background of study
The rapid development of population, industries, and burgeoning urbanization are contributing enormously to global environmental issues. Water pollution, in particular, is a problem that continues to worsen.1 Water is a very essential component of life, environment, and agriculture, and the quality of water influences both soil fertility and grain yield. According to the World Health Organization (WHO), in 2030, about 62% of the population of the world will suffer from water shortage. In developing countries, about 80% of wastewater is discharged into water bodies without any proper treatment.1 According to health experts, water-born diseases are increasing at a considerable rate and millions of people die annually on account of poor quality of water available for drinking. Approximately 1.2 billion people have no access to safe drinking water while 2.6 billion people have very little access.2 Water pollution is a severe problem, which is one of the leading causes of deaths and diseases worldwide.3 There are a large number of sources of water pollution, including domestic waste, industrial effluents, and the use of fertilizer, herbicides, and pesticides by the agriculture sector. These hazardous substances such as dyes, organic and inorganic pollutants, which have adverse effects on living organisms and biota, are the major cause of pollution in rivers, lakes, and oceans.4,5 There are many organic pollutants present in wastewater such as various organic solvents, phenolic compounds, dibenzofurans, dioxins, pesticides, chlorophenols, polychlorinated biphenyls, dyes, and new emergent organic pollutants.4
Among these, phenolic compounds are considered to be some of the most serious contributors to water pollution due to their high toxicity and carcinogenicity. These compounds are largely produced from different industrial processes and are often discharged into the environment without proper treatment.6 Due to the extensive usage of phenols in modern industries such as polymeric resin, paint, petroleum, and petrochemical industries, they have become prevalent in the environment causing severe water pollution.6 It is estimated that more than 10 million tons of phenol are discharged to the environment per year.11 The presence of phenol in water and air, even in very low concentrations, is a serious environmental and safety concern.7,8 The permissible level of phenol discharge from industrial effluents should not be greater than 5 ppm.12,13 Furthermore, even discharge to inland or water bodies with concentrations as low as 1 ppm is considered undesirable and toxic.9,10 The environmental standard for phenol in portable drinking water should not exceed 0.001 mg L−1 according to the World Health Organization (WHO).11 The environmental standard for phenol discharge into the environment has been set at 1 ppm by governmental organizations including the European Union and Malaysian Environmental Protection Agency.12–14 Thus, it is a matter of great importance to remove these phenolic compounds from wastewater to meet the above stringent standards.
2. Phenol properties
Phenolic compounds exist in nature in different forms. The parent compound is phenol (C6H5OH) which has a specific pungent smell that is a disgustingly sweet, medicinal, or tar-like odor. It is a flammable compound and causes disintegration of some coating materials, rubber, and some plastics on contact. It exists naturally in different types of food and animal and human waste.6,15 Table 1 lists some major physical and chemical properties of phenol. The second naturally occurring form of phenol is phenolic acids, also called phenic or carbolic acids, which were isolated in 1834 from coal tar for the first time by German chemist Rung.6 These aromatic compounds are mostly colorless white crystalline solids and are highly hygroscopic and soluble in water, carbon disulfide, oil, and in various types of an organic solvent such as ethyl alcohol, benzene, ether, hydrocarbons.18 Natural phenolic acids such as 3,4-dihydroxy benzoic, p-hydroxy benzoic, vanillic, caffeic, p-coumaric, ferulic, syringic, and sinapinic acids have been isolated from horse grams, mushroom, and human urine.16,17
Table 1 Physical and chemical properties of phenol
Chemical formula |
C6H5OH |
Molecular weight |
94.11 g mol−1 |
Boiling point |
181.75 °C |
Melting point |
40.9 °C |
Heat of fusion |
122.2 J g−1 |
Density at 40 °C |
1.0545 g mL−1 |
Density at 60 °C |
1.0413 g mL−1 |
Solubility in water |
9.3 g L−1 |
Vapor pressure at 25 °C |
0.35 mm Hg |
Color |
White crystalline |
pKa |
9.89 |
The wavelength and maximum absorbance (λmax) |
270 nm |
Henry's law constant |
0.034 pa m3 mol−1 |
3. Phenol synthesis and production
The annual production of phenol worldwide is estimated to be around 10 million tons per year.20 The major industrial method used for phenol production is the Hock process, otherwise known as the cumene-phenol process.20 In this process, phenol and acetone are simultaneously produced from benzene, propylene, and oxygen in three distinct steps.18 In the first step, cumene is synthesized by alkylation of benzene with propylene in the presence of acidic catalysts such as aluminum chloride, phosphoric acid, or zeolites. In the second step, cumene hydroperoxide is prepared from cumene by oxidation with air through free radical self-catalyzed reaction. In the third step, cumene hydroperoxide undergoes cleavage to phenol and acetone in the presence of sulphuric acid.6,19,20 Furthermore, phenol is also produced by several methods including the reaction of chlorobenzene with caustic soda at 350 °C.23,24 It worth mentioning that phenol is produced naturally during coal cooking, in which it is separated from other products in the form of sodium phenate using caustic soda, and also during the combustion of fossil fuels and tobacco. It was reported that many natural substances such as wine, tea, and smoked food contains phenolic compounds.18,21,22
4. Industrial applications of phenols
Phenolic compounds are used widely in many industries including resin production. About 35% of phenol is used to produce low-cost phenol-formaldehyde resin. Phenol-formaldehyde has a large number of industrial applications and can be used in construction, adhesive material, and appliance industries and automotive. Similarly, about 28% of phenol is used to produce epoxy resin. About 16% of phenol consumption goes towards the production of a mixture of cyclohexanone and cyclohexanol, which is further converted into caprolactam, the monomer of nylon. Adipic acid, another important monomer of nylon, is also derived from phenol. Adipic acid is produced by oxidation of the mixture of cyclohexanone and cyclohexanol by nitric acid. Phenol may be converted into xylenols, alkylphenols, chlorophenols, aniline, and other secondary intermediates in the production of surfactants, fertilizers, explosives, paints, and paint removers, textiles, rubber, and plastic plasticizers and antioxidants, and curing agents and so on.23,24 The typical phenol concentrations in waste released by various industries are given in Table 2.
Table 2 Phenol sources and their typical concentrations
Industrial source |
Concentration range (mg L−1) |
Reference |
Coal conversion |
1700–7000 |
24 |
Coke oven |
600–3900 |
25 |
Phenolic resin |
1200–1600 |
26 |
Petrochemical |
200–1220 |
27 |
Textile |
100–150 |
28 |
Fiberglass industry |
40–2564 |
29 |
Leather |
4.4–5.5 |
30 |
Pulp and paper industry |
20–80 |
31 |
Paint industry |
1.1 |
32 |
Phenol and its derivatives are also used as slimicides and disinfectants. It is used as a base compound in the formulation of carbolic soap due to its antiseptic properties and has been safely used in surgery since 1867. Various types of medicine, such as sore throat spray, as an oral analgesic for the relief of pain in or around the mouth, are prepared using phenol. In veterinary medicine, it is utilized as an internal and gastric anesthesia. Additionally, it was also utilized in the form of phenol injection for muscle tightening to treat a condition known as muscle spasticity and employed as a preservative for vaccines used against pneumonia, typhoid, smallpox, and polio. The most important pharmaceutical application of phenol is in the synthesis of aspirin. Furthermore, phenolic compounds derived from plants are known as natural antioxidants and can stop free radicals, thus protecting DNA from breakage and hence helping to prevent cancer.6
5. Toxicity of phenol
It has been observed that the release of phenolic substituted compounds from industrial effluents into water bodies causes various human health problems.33 High exposures to phenol may be fatal to human beings, with infants being more susceptible.34,35 Direct human exposure to phenol can cause excretion of dark urine, sore throat, decreased vision and can irritate eyes and skin. Furthermore, phenol exposure can cause some chronic side effects such as anorexia, weight loss, diarrhea, vertigo, growth retardation, and inflammation in the gastrointestinal, liver, kidney, central nervous system, and cardiovascular tissues.38 Phenolic contaminated wastewater is discharged frequently to the aquatic system and, as such, adversely affects the aquatic ecosystem, vertebrates, invertebrates, algae, and protozoa. This may lead to growth inhibition and a decrease in the survival of their offspring.36 Thus, wastewater containing phenolic compounds must be treated properly before discharge into the environment.
6. Phenol removal from contaminated wastewater
As explained previously, the treatment of water contaminated with phenol is very important to reduce the impact on environmental and human health problems. The technology available for removal of phenol can be generally classified into three categories: chemical, physical, and biological treatment technologies, with each having its advantages and drawbacks.4,35 However, the wastewater coming from different sources can be complex and no single method can be used to treat wastewater effluent to the desired level. Practically, a combination of methodologies must be applied for wastewater treatment to achieve the desired output quality.37,38
Chemical methods include chemical oxidation, photocatalysis, electrochemical, ion exchange, precipitation, irradiation, electroflotation, and coagulation/flocculation. The effectiveness of these methods depends on the pollutants in the wastewater and their interactions with the reactants.39 The chemicals used in these methods can either assist in the separation process or neutralize some of the toxic effects caused by the pollutants.40 However, in these methods, various expensive chemicals are used and, therefore, they are considered not economically feasible to be employed on the industrial scale.35,38,41 Furthermore, these methods generate large amounts of sludge and secondary pollutants after treatment resulting in sludge disposal problems.42,43 Powerful oxidizing agents such as hydroxyl radicals are produced during oxidative processes which are very effective in pollutants degradation. However, this approach is considered to be chemically and energy-intensive.44,45 In some chemical degradation methods, chlorine is used as an oxidizing agent which produces highly toxic compounds such as organochlorine.46 Hence, chemical methods are not preferred due to the associated high treatment and sludge disposal costs, which make such processes unattractive for use on an industrial scale.
Various physical methods such as electrodialysis, adsorption techniques, membrane filtration processes, nanofiltration, and reverse osmosis are employed for phenol removal from contaminated wastewater.47–49 The main shortcoming of the membrane separation method is the short lifetime of the membrane and, thus, it becomes economically unattractive due to the need for periodic replacement.50 Adsorption is extensively used in wastewater treatment due to its effectiveness in removing pollutants and decolorizing the water effluent.51–54 A properly designed wastewater treatment plant utilizing an adsorption system produces a high-quality treated effluent. Adsorption offers an attractive alternative treatment method for contaminated waters, particularly in cases where the adsorbent is inexpensive, locally available, abundant, and requires only simple pre-treatment before it is used.55
Biological degradation of phenol is economical compared to the chemical and physical treatment process, but the processes involved are considered complex in nature. Various types of microorganisms such as algae, fungi, yeast, and bacteria have the potential to break down various types of pollutants including phenol. In this method, different types of microorganisms such as sphingomonas, pseudomonas strains, white-rot fungi, microbial cultures, under aerobic, anaerobic, or mixed conditions, are employed in phenol removal.54,56,57 However, biological degradation methods are time-consuming and hence, are considered impractical for the treatment of large quantities of industrial wastewater contaminated with phenol.58 The advantages and disadvantages of the available phenol removal technologies are listed in Table 3.
Table 3 Advantages and disadvantages of available phenol removal technologies65,66
Methods |
Advantages |
Disadvantages |
References |
Biological treatments |
Biological degradation |
• Phenol is consumed by microorganisms such as bacteria, algae, yeast, and fungi and convert them to harmless compound |
• Can lead to toxic by-products |
67–69 |
• Growth control problem |
• Not suitable for high concentrations of phenol |
• Sludge production |
• Requires use of co-solvent when the concentration of phenol is low |
Enzyme degradation |
• Enzymatic reactions are specific in nature and happened under moderate pH and temperature |
• Non-reusability of the enzymes |
69–71 |
• Higher catalytic efficiency and lower cost than the traditional chemical methods |
• Enzyme instability in the harsh environment of the wastewater |
![[thin space (1/6-em)]](https://www.rsc.org/images/entities/char_2009.gif) |
Chemical treatments |
Oxidative process |
• In gaseous oxidation, there is no increase in the volume of wastewater and sludge |
• Use of expensive chemicals |
|
• Simplicity of application |
• Incomplete oxidation of phenol |
72 and 73 |
• Safety issue due to the use of hazardous chemicals |
• H2O2 needs to be activated by some other means |
• Wet oxidation of phenol is not economical due to the need for high pressure and temperature |
Electrochemical destruction |
• No need for expensive chemicals |
• Requires expensive equipment |
74 |
• Sludge is not produced |
• High energy consumption |
• Safety issues in handling toxic chemicals |
Photochemical |
• Phenols are greatly degraded, and sludge is not produced |
• By-products are formed |
75 and 76 |
• Expensive equipment is needed |
Fenton reagents (H2O2 + Fe(II) salts) |
• Fenton reagents are environmentally safe and therefore can be easily handled |
Sludge production |
77 and 78 |
• No need for expensive and complicated apparatus |
Irradiation |
• Effective oxidation at lab scale |
The requirement of a high amount of dissolved O2 |
|
![[thin space (1/6-em)]](https://www.rsc.org/images/entities/char_2009.gif) |
Physical treatments |
Membrane filtration |
• Removes all types of dyes |
• Concentrated sludge is produced |
79 |
Electrocoagulation |
• Economically feasible |
• Production of large amounts of sludge |
80 |
Distillation |
• Phenols are separated from aqueous media based on relative volatility |
• Needs high energy consumption |
37 |
• Used for low concentration of phenol removal |
Adsorption |
• Good for removal of phenol |
• Regeneration is difficult |
4, 81–83 |
• Need mild temperature and pressure |
• Regeneration need calcination or the use of solvent |
• Economical |
• Many adsorbents have low adsorption efficiency |
• Easy to operate and no expensive equipment's required |
• Not suitable for adsorption low level of phenol |
• Sometimes chemicals are used for adsorbent modification which are expensive and toxic |
|
• Sometimes need a high amount of adsorbent required |
Liquid–liquid extraction |
• Easy to operate |
• Sometimes has low selectivity |
66, 83–85 |
• Performed at mild conditions |
• Use of toxic, flammable, and volatile solvents in the extraction process |
• The extract can be recycled as a raw material |
• Regeneration of solvent might be expensive and challenging |
Ion exchange |
• Regeneration of adsorbent |
Not effective for all dyes |
66 |
The liquid–liquid extraction technique is currently considered the most attractive method for the removal of phenol and other pollutants from contaminated wastewater.59–61 However, this method is generally applied where the concentration of phenol is very high. Liquid–liquid extraction is based on the differential solubility of the phenols between the water phase and the water-immiscible solvent phase employed as the extractant. After contacting the phases and extracting the phenols, the two layers are separated from one another. This method is easy to operate under mild conditions and it does not cause any change to the extraction solvent or phenol. The phenol after extraction can, therefore, be recovered and reused as a raw material. Different types of organic solvents, such as hydrocarbons and oxygenated compounds, are used to extract phenol from wastewater.38,62 Most frequently, phenol is extracted from water using volatile aromatic and aliphatic organic solvents such as ketones, acetates, ethers, and alcohol. However, typical organic solvents have high vapor pressures, resulting in atmospheric contamination as volatile organic compounds (VOCs). Furthermore, they are usually highly flammable and are often toxic.63,64 Therefore, considering these environmental and safety issues, researchers are trying to design novel solvents, such as room temperature-hydrophobic ionic liquids (IL), which can efficiently extract phenol from wastewater in an environmentally benign and safe manner.
7. Overview of ILs
Though the exact definition of ILs is debatable, in general, ILs are organic salts that contain more than 95% ionic moieties (cations + anions) and have melting points below 100 °C.86–88 Therefore, various terms have been used in the literature, such as liquid electrolytes, ionic solvents, ionic fluids, ionic glasses, fused salts, and liquid salts.91–93 Due to their ionic nature, ILs have superior properties over other common organic solvents for certain applications and hence are considered to be attractive solvents to both academic and industrial fields.89–91 The most important properties of ILs are their negligible volatility, excellent solvation properties, and their thermal, electrochemical and chemical stability.92,93 The properties of ILs are effected by the chemical nature and structure of the anions and cations, as well as the alkyl chain length. Investigation of the recent literature reveals that interest in ILs as hydrophobic solvents for liquid–liquid extraction of phenol has increased considerably in the last few years. This is reflected by the significant increase in the number of publications, as illustrated in Fig. 1. This trend demonstrates the attractive properties of ILs as substitutes for organic solvents.
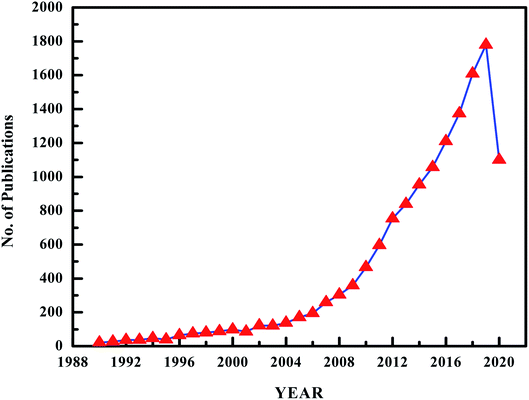 |
| Fig. 1 Number of publications per year on phenol extraction using ILs. | |
7.1 Quantitative structure–properties relationships for IL tunability
Interest in ILs for industrial use is increasing due to their physicochemical tunability that results from changing the combinations of anions and cations.Therefore, ILs can be designed according to the desired need by appropriate selection of anions and cations, while fulfilling the criterion that the designed salt has a melting point below 100 °C.86,94 To fulfill this very basic and fundamental requirement, the ions of ionic liquids should be asymmetric and univalent so that a close packing lattice structure is not possible, thus lowering the melting points to the desired level.88,91 Due to their tuneability and excellent physicochemical properties, ILs have found several applications in the field of biomass processing, carbon dioxide and sulfur dioxide capturing, electrical energy storage, organic and inorganic synthesis, extraction of compounds from water, polymerization, drug delivery, extraction of collagen, bone filler, and lubricants, to name a few.95–98
In general, ILs are designed by combining bulky organic cations such as imidazolium, pyridinium, piperidinium, pyrazolium, phosphonium, and ammonium with various organic or inorganic anions. The selection of ion pairs plays a major role in the thermo-physical properties as well as the solubility and miscibility of ILs in various organic and inorganic solvents.90,91,99–102 The physical properties such as density, surface tension, thermal properties, refractive index, viscosity, and acidity are key factors in the design and performance of ILs based industrial processes.122,123 These physicochemical properties can be tuned to a greater extent either by changing the cation or the anion.103 Hence, a wide range of experience in the selection of smart anion and cation is required to design ILs having desired physicochemical properties for the desired application. Literature review reveals that acyclic cations such as phosphonium, ammonium, sulphonium, cholinium, and cyclic cations such as imidazolium, pyridinium, pyrrolidinium, and piperidinium, are employed in the design of ILs (Fig. 2)87,89,101,104–106 with imidazolium cations being the most extensively studied.104 On the other hand, more flexibility is available for the selection of anions since these could be inorganic, such as halides, cyanate, phosphate, sulfate, nitrate, borate, as well as organic, such as phenolate, benzoate, malonate, and amines (Fig. 3). It is worth mentioning that the properties of ILs could be further altered by the functionalization of both the selected cation and anion.105–107 This functionalization could involve the incorporation of long-chain hydrocarbons, ether, hydroxyl, phenyl, nitrile, amide and halide groups into ILs.
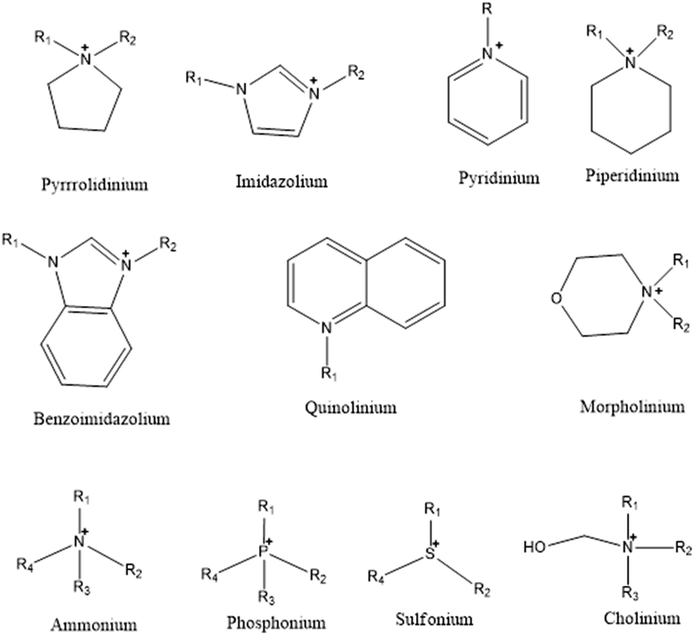 |
| Fig. 2 Structures of common IL cations. | |
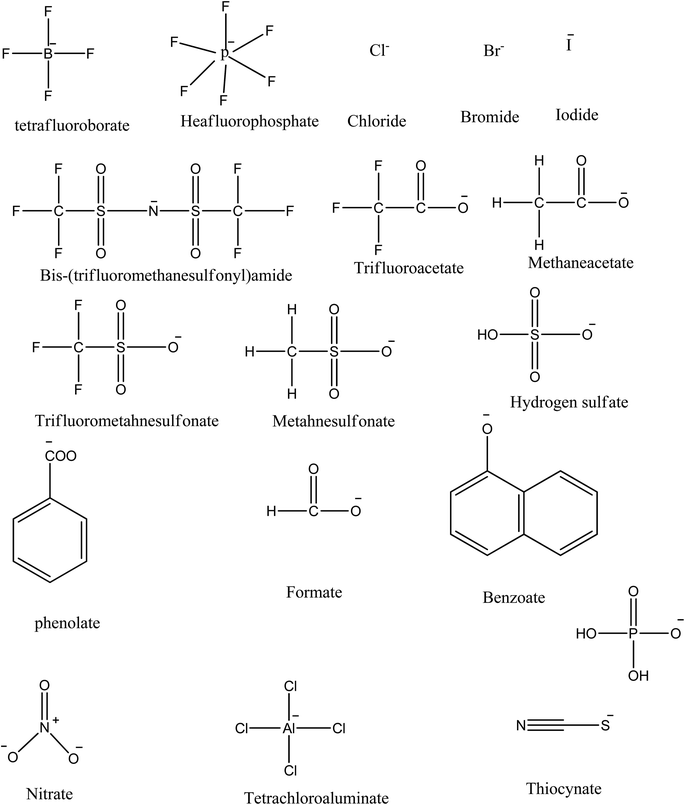 |
| Fig. 3 Structures of common IL anions. | |
7.2 Technical and ecological aspects of ILs
7.2.1 Thermal stability and melting point. Although they are often considered expensive, ILs can be economically feasible for industrial use when are thermally stable, have a wide liquid range, and can be easily regenerated for further use.108 The liquid range of an IL is the region between the melting point and the decomposition temperature. ILs with a high decomposition temperature and low melting point possess a wide liquid range and, therefore, can be used over a wider range of operating conditions. High thermal stability makes ILs suitable for thermal storage liquids applications in electric power, for example. IL thermal stability can be managed by careful selection of anions and cations.109 In general, IL melting point is a function of both the symmetry and charge of both cations and anions. Those ILs having symmetrical cations have a higher melting point than those having asymmetrical cations,110 and the melting point increases considerably with the elongation of alkyl chain length attached to the cation. For example, Shimizu et al. found that [C18mim]NTf2 melts at a higher temperature than ILs with shorter alkyl chain lengths. This increase in the melting point with an increase in alkyl chain length is due to the increase in van der Waals interaction.111 Hence, it is vital to determine the thermal properties of ILs before their selections for the desired application. ILs containing anions derived from Bronsted acids and imidazolium cations tend to be thermally stable and are considered suitable to use at high temperatures.112–114 High thermal stability is also important to avoid the risk of an explosion when ILs are used in industrial applications.115
7.2.2 Low vapor pressure. The low vapor pressure of ILs is one of the most vital and important properties of ILs, which makes them distinctive solvents for industrial applications.116,117 In contrast to the typically used volatile organic solvents, ILs have negligible vapor pressure and, therefore, do not escape into the atmosphere. ILs have very large latent heat of vaporization (ΔHvap = 120–200 kJ mol−1), which is considerably higher than common organic solvents, thus enabling them to be used for the extraction of pollutants from water and oils without contamination of the atmosphere.114
7.2.3 Broad range of solubility and miscibility. One of the most important properties of ILs is their wide range of solubility and strong solvation properties in contrast to conventional organic solvents.64,104,114 It is known that ILs have the ability to dissolve a very wide range of organic and inorganic materials. For example, ILs can dissolve CO2, H2S, SO2, cellulose, biomass, inorganic salts, transition metals, antibiotics, coal, collagen, asphaltene, and enzymes.95,96,118–120 The broad range ability of ILs to solubilize different materials can be attributed to the large freedom in the selection of anions and cation for a specific application. For example, it has been shown that, even though the polarities of ILs and short-chain alcohols are quite similar, their solvation properties are quite different.121 The solvation properties of ILs can be easily managed for the dissolution of polar and non-polar substances by the selection of appropriate cations and anions.122 Efficient and realistic use of ILs as solvents requires the knowledge of their physical and chemical properties such as density, viscosity, surface tension, and thermal stability. Just as important, yet less studied, is knowledge of IL polarity, as this property reflects the solvation capability of these fluids for a given solute as well as reaction rates, reaction mechanism, product yield, and enzymatic activity among others.123–125 In this perspective, it is crucial to obtain information regarding the polarity of ILs before their utilization for a specific application.
7.2.4 Wettability and regeneration of ILs. One of the most important properties of ILs is their wettability of hydrophilic and hydrophobic surfaces.126,127 The wettability can be adjusted, like other physicochemical properties, by the careful selection of the cation–anion combination. Hydrophobic and hydrophilic properties of ILs are controlled by both anions and cations. ILs which have longer alkyl chain length cation are more hydrophobic.128 Similarly, the ILs containing bis-(trifluoromethanesufonyl)amide ([NTf2]), hexafluorophosphate ([PF6]) and tetrafluoroborate ([BF4]) anions are hydrophobic in nature.129,130 This hydrophobic character and higher efficiency of ILs are the main features of ILs which make them distinct to be used in phase separation.131 Furthermore, the regeneration and recycling of ILs are considered the most important and vital property in ensuring the environmental and economic feasibility of their industrial application. As a result, this aspect has attracted the attention of many researchers in this field.88,122
8. Limitations of ILs
Although ILs are considered a unique class of solvents having a large number of applications in various fields, they still suffer from certain drawbacks that limit their usage on commercial scales. These limitations include toxicity, high viscosity, high cost, and biodegradability. A short discussion on each is given below.
8.1 Toxicity and biodegradability of ILs
Though ILs can be considered greener than volatile organic solvents due to their low vapour pressure, non-flammability, thermal stability, and ease of regeneration, which minimizes their loss to the environment, recent research shows that many ILs can pose some risks to both terrestrial and aquatic biota. It has been documented in the literature that the toxicity of ILs depends on their cationic and anionic nature.132,133 It has been found the alkyl chain length and the nature of the functional groups are important factors in determining the toxicity of ILs.120,121 An increase in alkyl chain length on cations causes an increase in the toxicity of ILs.134 It has been observed that the toxicity of guanidinium based ILs towards Vibrio fischeri increases as alkyl chain length increases from C7 to C12 (ref. 135) However, it has also been reported in the literature that toxicity of some ILs increases with alkyl chain length up to a certain limit and, after that, no increase in toxicity is observed, which is called the “cut-off” effect. The toxicity of phosphonium based ILs against Vibrio fischeri also increases with the increase in alkyl chain length from [P4,4,4,4] to [P6,6,6,14].135,136 However, incorporating certain functional groups onto the alkyl chain can reduce toxicity and enhance their biodegradability. Wang studied the effect of functionalization of cation on the toxicity of ILs against Clostridiums sp. and found that 1-methoxyethyl imidazolium tetrafluoroborate [Moemmi][BF4] which contains the methoxy functional group (CH3O) is comparatively less toxic than [C4mim][BF4].137 Therefore, it is necessary to choose such cations and anions or functional group carefully before designing task-specific ILs to reduce their hazardous potential. The fluorinated anions such PF6 offer potential risk due to their tendency to be hydrolyzed.133,136 It has also been found that ILs based on choline chloride are less toxic than imidazolium, pyridinium, piperidinium, and pyrrolidinium-based ILs.138
In order to minimize the loss of ILs to the environment, and improve their regenerability, attempts were made to immobilize ILs on solid supports.139 Furthermore, new types of IL containing cations derived from natural sources such as amino acids, choline, and fatty acids were developed with higher biodegradability and, thus, facilitating greener and more sustainable processes.140 The toxicity of ILs can be also mitigated by the proper selection of anions, with NO3− being shown to be environmentally friendly.141 Therefore, it is crucial to study the biodegradability of ILs as a function of ion pairs, functional groups, and alkyl chain length. Thus, well documented toxicological data of ILs is needed before they can be used extensively for industrial applications.
8.2 Viscosity of ILs
Viscosity is an important thermophysical property of ILs from an engineering point of view and it is required for many calculations such as mass transfer, modelling, fluid flow, and equipment design. However, the viscosities of ILs are higher than those for common organic solvents. This high viscosity is considered a great drawback and poses a serious limitation in industrial applications.142,143 On the other hand, high viscosity in certain applications, such as lubrication, is favorable. IL viscosity is considered an important property in the extraction process, however, there is no report available on the effect of viscosity on phenol extraction. It is expected that increasing the viscosity will lead to a decrease in the rate of molecular transport and hence reduce the efficiency of phenol extraction. Low viscosity ILs are considered better in most applications due to several factors such as handling, recovery, increased mass transfer rate, and having better extraction and catalytic properties.144–146 The viscosity of ILs can be altered through the selection of the ion pairs. Viscosity of ILs is also effected by addition of salts,147,148 temperature and addition of molecular solvents.149,150 It is noted that a large number of possible combinations of anions and cations are possible so that the desired viscosity can be achieved.
8.3 Cost of ILs
The cost of ILs is considered one of the major factors limiting the use of ILs on an industrial scale. Compared to common organic solvents, ILs are considerably more expensive. The high cost of ILs can be attributed to the high price of precursors as well as the complex synthesis and purification processes.151,152 However, it is expected that the cost of ILs will decrease with economies of scale as the technology advances and the demand increases.153,154
8.4 Purity of ILs
The purity of ILs can considerably impact their physicochemical properties. For example, the presence of a small amount of impurities or water in ILs significantly affect properties such as viscosity.155 This factor is hard to control due to the purity of the precursors during synthesis and the limited purification techniques to obtain the ILs in pure form. For example, during the synthesis of [C4mim][C8H17OSO3] excess reactants and the solubility of hydrocarbon anion limit isolation of the product in the pure form.156
9. Effect of ILs structure on phenol extraction
9.1 Effect of anion
Hydrophobic ILs such as [C6mim][BF4], [C6mim][PF6] [C8mim][BF4], and [C8mim][PF6] were used to study the effect of both anion and alkyl chain length on the extraction of phenol, p-hydroybenzoic acid and tyrosol.137,138 It was reported that ILs containing BF4 anion were more efficient in the extraction of all the above compounds compared to ILs contain PF6 anion.157 Furthermore, various phenolic compounds such as phenol, chlorophenol, 4-nitrophenol, 2,4-dinitrophenol, 2,6-dintrophenol, 1-naphthol, 2-naphthol were extracted from aqueous solution using [C4mim][PF6]. It was also reported that the nature of the IL anion affects the extraction of phenol from aqueous solution,158 with anions having hydrophobic nature being more efficient than other types. This enhanced efficiency could be attributed to the intermolecular forces that result from the hydrogen bonding with fluorine atoms on the anion and OH group on phenol as well as hydrophobic interaction between the hydrocarbon chain and the phenol ring π system. Furthermore, a large number of ILs containing different anions such as NTf2−, Cl−, BF4−, PF6− and SCN− and different cations such imidazolium, pyrrolidinium, and pyridinium are reported for phenol extraction.84,159–162 Fan et al.158 compared the efficiency of ILs containing BF4, PF6, and NTf2 anions towards phenol extraction and reported that BF4 was the most efficient. This observation was attributed to the strength of the hydrogen bonding formed between the anion and the phenol group. On the other hand, it has been concluded that BF4 has a strong electronegativity atom and therefore, has stronger H-bonding with phenol. Furthermore, imidazolium based ILs containing Cl−, Br−, BF4− and PF6− as anions were used for phenol extraction and their efficiency followed the order: Cl− > Br− > BF4− > PF6−.142 This result further supports the hypothesis that the nature of the IL anion has a key role in phenol extraction. On the other hand, ILs containing tetraethylammonium cations and various amino acid-based anions such as alanine, glycine, proline, sarcosine, and lysine showed high efficiency of phenol extraction (>97%) with small differences between them.143 In this case, the nature of the cation predominates in determining phenol removal efficiency. In another study with an imidazolium-based IL containing lactate anion, similar observations were reported with efficiency towards phenol removal >99.9%.144 This observation further supports the previous conclusion that the nature of the cation could predominate over the anionic nature in determining the extraction efficiency. To test this hypothesis, two types of ILs with different combinations of anions and cations were used for phenol extraction.145 For addressing the effect of anions, two ILs contain the same cation [C4C1Py] with bis(fluorosulfonyl)imide (Nf2) and NTf2 were tested for the extraction of phenolic compounds and showed that NTf2 is more efficient than that of Nf2.162 In addressing the effect of the nature of cations, two ILs containing C4C1Py and C4Py cation and NTf2 and Nf2 anions were tested for the removal of phenol, with the former being the most efficient. This observation was attributed to the aromatic character of cations, which supposedly enhances the extraction efficiency. In a further study,146 ILs having [C6mim] as cation with BF4 and PF6 as anion were tested for phenol removal and the results showed that the removal efficiency of phenol by BF4 based ILs was higher than that with PF6. This was due to differences in the nature of anions and their H-bonding interaction with phenolic compounds. Theoretical calculation by quantum mechanics supported the experimental result by confirming that the effective charge of BF4 anion is stronger than that of PF6.146 Hence, the H-bonding interaction of BF4 with –OH of phenol is predicted to be stronger than PF6 and will effectively remove higher amount of phenols.
9.2 Effect of cation
IL is constituents by anions and cations, the nature of cation also plays a major role in the extraction of phenolic compounds. Five ILs were selected to test with the aim to study the effect of the nature of cation on the removal efficiency of phenol.145 These include the cations [C4Py], [C4C1Py], [C4C1Pip], [C4C1Pyr], and [C4C1Pyr] with NTf2 as the anion.The results indicate that, in this case, the nature of the cation plays an important role in the extraction efficiency of phenol, with [C4C1Py] being the most efficient among those studied, followed by [C4Py]. This observation was attributed to the aromatic character of the cation which might enhance the extraction efficiency of ILs. On the other hand, another study on the extraction efficiency of various phenolic compounds such as phenol, o-cresol, and resorcinol by ILs containing non-aromatic ([C6mpyr][NTf2]) and aromatic ([C6mim][NTf2]) cations,163 showed that ILs containing non-aromatic pyrrolidinium cations have higher efficiency than ILs containing aromatic ([C6mim]) cations. Hence, the above conclusion regarding the aromaticity of cations cannot be generalized. Therefore, it can be concluded that H-bonding is the main contributor to the phenol extraction by these ILs. Egorov synthesized hydrophobic ILs containing ammonium cations, such as tetrahexylammonium dihexylsulfosuccinate (THADHSS) and trioctylmethylammonium salicylate (TOMAS) for phenol extraction.164 It was found that TOMAS was better in the extraction of phenol than THADHSS. The extraction properties of these two ILs were better than imidazolium-based ILs using for phenol extraction. The author claim that this higher extraction efficiency of both ILs might be due to the dispersive interaction of phenol with the cation of both ILs.
9.3 Effect of alkyl chain length
Pei et al. used [C6mim] and [C8mim] based ILs containing hydrophobic BF4 anion instead of organic solvents such as phenol and phenyl amines.165 This study suggested that the cation has dispersion interaction with the solute while the anion interacts with the solute via H-bonding. It was reported that the extraction efficiency of phenolic compounds increases with the increase in alkyl chain length from C6 to C8. The authors suggested that the increase in extraction efficiency is due to the more hydrophobic nature of longer alkyl chains. In another study, Sidek et al.150 used 1-allyl-3-benzyimidazolium chloride, 1,3-dibenzylimidazoilum chloride, and 1-benzyl-3-vinylimidazolium chloride for liquid–liquid extraction of phenolic compounds from hexane as the model oil. Functional groups such as ally, benzyl, and vinyl were included in the selected cations with the aim of studying their effect on phenolic compounds extraction. It was found that all these synthesized ILs have the potential to remove the phenolic compounds from the model oil. It has been concluded from the experimental data that allyl and benzyl groups significantly improve the extraction of phenolic compounds from hexane. This increase in efficiency of ILs with merging benzyl and allyl groups is due to the increase in their hydrogen bonding and also π–π interaction capacity of aromatic moieties. The NMR analysis confirmed that the benzene ring of phenol interacts with allylic and benzylic groups and also with π electrons of the imidazolium ring. This study also revealed that the long double bond group on the benzyl imidazolium has higher extraction efficiency for phenol than the shorter double bond. In the case of the long conjugated double bond, freely moving chloride anions make strong interactions with phenol compared to the localized shorter unconjugated double bond in which chlorine is tightly-attached to the C(2)–H of imidazolium ring. Furthermore, Fan et al.166 studied the effect of alkyl chain length ([Cnmim], n = 4 to 8) on phenol extraction from aqueous media using BF4 and PF6 as anions in ILs. It has been observed that the extraction efficiency of these ILs under the same experimental conditions following the trend [C4mim][PF6] < [C6mim][PF6] < [C8mim][PF6]; [C4mim][BF4] < [C6mim][BF4] < [C8mim][BF4]. The results demonstrate that for these ILs the distribution efficiency of phenol in IL increases with increasing alkyl chain length on the imidazolium cation from C4 to C8. These observations indicate that the alkyl chain length leads to higher distribution of phenol in ILs by virtue of the enhanced hydrophobic interaction between the ILs and phenolic compounds. Fan et al.158 studied the impact of ILs structure on phenolic compounds extraction from water at pH = 7. To make the comparison easy, the ILs were classified into eight groups as shown in Fig. 4. These are: (I) [C8mim][NTf2], [C4C7im][NTf2], [C4C9im][NTf2], and [C4C12im][NTf2]; (II) [C8mim][PF6], [C4C7im][PF6] [C4C9im][PF6] and [C4C12im][PF6]; (III) [C8mim][BF4], [C4C7im]BF4, [C4C9im][BF4] and [C4C12im][BF4]; (IV) [C4C6OHim][NTf2], [C4C8OHim][NTf2] and [C4C11OHim][NTf2]; (V) [C4C6OHim][PF6], [C4C8OHim]PF6, and [C4C11OHim]PF6; (VI) [C4C8OHim]BF4 and [C4C11OHim]BF4; (VII) [C4Beim][BF4] and (VIII) [C4Beim][NTf2]. As far as the alkyl chain length on cation is concerned, it has been observed that increasing the hydrophobicity of ILs by increasing alkyl chain length has no considerable effect on the phenolic compounds extraction. Khan et al. found that phenol extraction efficiency decreases with increasing alkyl chain length of cation of ILs.167,168 This finding further supports the above conclusion (Section 9.2) that hydrogen bonding plays a vital role in phenolic compound extraction in these cases.
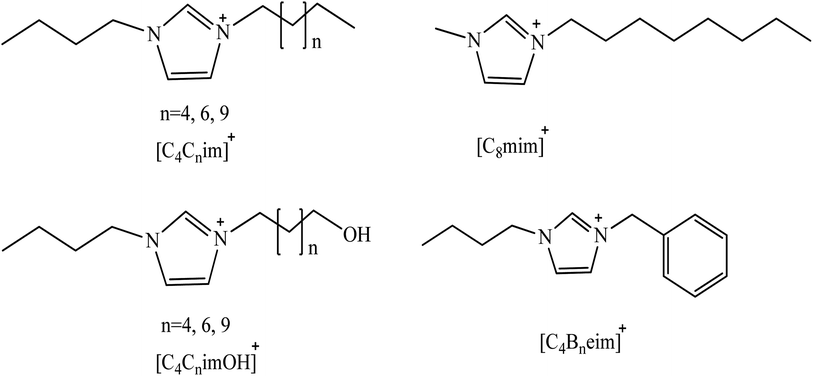 |
| Fig. 4 Structure of ILs cations used by Fan et al.140 | |
9.4 Effect of functional groups on anion and cation
Few studies in the literature are available on the effect of incorporating functional groups to the ILs on phenol extraction. It is expected that functionalizing the cations and anions of ILs will considerably affect the extraction of phenol from aqueous media or crude oil. Fan et al.158 studied the effect of functional groups on the extraction efficiency of phenol from aqueous media using imidazolium-based ILs containing various anions (BF4−, PF6−, and NTf2−) and alkyl chain length. In order to study the functional group effect on phenol extraction, benzyl, hydroxyl, and dialkyl functional group were incorporated into the IL cation. It has been shown that the incorporation of the hydroxyl group into the IL structure significantly increases the phenol extraction efficiency for ILs having NTf2 and BF4 as the anion. This increase in extraction efficiency is due to the increase in hydrogen bonding with the incorporated functional group. Furthermore, it has been confirmed by studying the thermodynamic parameters, that H-bonding plays a vital role in phenolic compound extraction. The negative values of the thermodynamic parameters of extraction such as ΔS and ΔH suggest that H-bonding is mainly responsible for phenolic compound extraction.
Yao et al.169 synthesized and employed four different dual functionalized ILs containing various cations for phenol extraction from oil. These are 1,(2-(diethylamino)ethyl)-3-methyl imidazolium chloride ([Et2NEmim][Cl]2), 1,(2-(diethylamino)ethyl)-3-methyl morpholinium chloride ([Et2NEmmor][Cl]2), 1,(2-(diethylamino)ethyl)-3-methyl pyrrolidinium chloride ([Et2NEmpyr][Cl]2) and 1,(2-(diethylamino)ethyl)-3-methyl pyridinium chloride ([Et2NEmpic][Cl]2). The extraction efficiency of these ILs was compared with the already reported ILs such as choline chloride and [C4mim]Cl. It was found that all these newly prepared ILs are very efficient in phenol extraction, with a very low mole ratio of 0.3, which is almost half the amount of that used in conventional extraction. This high efficiency was attributed to the interaction of the Cl anion with the H atom of the hydroxyl group on phenol.
Recently, phenolic compounds have been extracted using IL based aqueous biphasic systems (IL-based ABS). Aqueous solutions of hydrophobic ILs can converted into IL-based ABS by adding inorganic salts to the ILs. IL-based ABS is an alternative and attractive approach applied for separation processes instead of liquid–liquid extraction. [C8mim][PF6] and [C4mim][Cl]/(K2CO3, K2HPO4 or K3PO4) ABS were used for extraction of phenol, and 4-nitrophenol from aqueous phase.170 Other research group have used ABSs containing [C3-8mim][BF4], 6-(hydroxymethyl)oxane-2,3,4,5-tetrol and water to extract phenol.171 Various process parameters effect such initial phenol concentration, temperature, phase forming components, and alkyl chain length attached to imidazolium cation were investigated. Increases in the phase forming components, concentration, particularly the concentration of glucose and alkyl chain length attached to the imidazolium ring resulted in a considerable increase in the phenol extraction to IL-rich phase. The value of D for phenol was about 78, which is comparable with literature.
10. Effect of process parameters on phenol extraction
10.1 Effect of pH
It is well known that the pH of a sample solution could significantly influence extraction efficiency, particularly when acidic or basic solutes are extracted. Phenol speciation is known to be a function of pH, which leads to different forms due to the ionization of –OH group. At pH lower than 9.23 (<pKa), the phenol exists in the molecular form (C6H5OH), whereas at pH > 9.23 (>pKa), the phenolate ion (C6H5O−) is the predominant form. NTf2 based ILs such as [C3mim][NTf2], [C4mim][NTf2] and [C6mim][NTf2] were investigated for phenol extraction in pH range from 2 to 10. No change in phenol extraction was observed in the pH range from 2 to 6, however, it was found to sharply decrease when pH reached 10.163 Sualiman et al. also studied the effect of pH on the phenolic compounds using NTF2 based ILs and found that maximum removal is obtained at pH < 7.170 Deng et al. used trihexyltetradecylphosphonium tetrachloroferrate ([3C6PC14][FeCl4]) for the phenolic compound exaction. A higher distribution ratio was found in acidic conditions for all phenolic compounds.171 Furthermore, an investigation by Egorov confirmed that the maximum distribution of phenol occurs at acidic pH levels and a drastic decrease in distribution occurs at pH > 12.164 In another study, Vidal et al. found that the extraction of phenol was higher at acidic pH levels using [C6mim][BF4], [C8mim][BF4], [C4mim][PF6] and [C8mim][PF6].157 This higher percent removal of phenol at low pH could be attributed to the higher affinity of the IL toward the molecular form of phenol. This might be due to the hydrogen bonding between the –OH of phenol with ILs. However, at higher pH (above pKa), the OH group of phenol ionized leading to the formation of phenolate ion (C6H5O−), which results in a reduction of the strength of the H-bonding interaction between phenol and ILs.14,172,173
10.2 Effect of initial concentration
Sas et al.161 extracted chlorophenol and resorcinol from water using the bis(trifluoromethylsulfonyl)imide [NTf2]-based ionic liquids (ILs). These include 1-methyl-3-propylimidazolium bis(trifluoromethylsulfonyl)imide ([C3mim][NTf2]), 1-butyl-3-methylimidazolium bis(trifluoromethylsulfonyl)imide ([C4mim][NTf2]), and 1-hexyl-3-methylimidazolium bis(trifluoromethylsulfonyl)imide ([C6mim][NTf2]). The starting concentrations of the phenolic compounds in water were 3, 5, 10, 100, 300, 500, 2000, 5000, 10
000, and 15
000 mg L−1 at pH lower than the pKa values of the phenolic compounds in all cases. It was concluded that an increase in the initial concentration of the phenolic compounds causes an increase in the extraction efficiency by the selected ILs. Hence, it can be concluded that saturation has not been reached and the solubility of these phenolic compounds in the ILs phase is much higher than the concentrations used in these studies. Furthermore, NTf2 based ILs were used to study the effect of the initial concentration of phenol on its extraction efficiency. It was reported that the phenol extraction efficiency of all the selected ILs increases with the increasing initial concentration of phenol. Specifically, the extraction efficiency of the phenolic compounds from aqueous solution increases from 50% to more than 90% when the initial concentration of phenol is higher than 5 g L−1.160 On the other hand, a study by Gonzalez revealed that a decrease in phenolic compound extraction is observed with the increase in the initial concentration of phenol using NTf2 based ILs.148 This observation was supported by a study performed by Brinda et al.159 who found that the extraction efficiency of [Bmim][BF4] decreases with an increase in the initial concentration of phenol. In addition, a study by Ji et al. used dicationic imidazolium-based ILs such as (1,2-bis[N-(N′-methylimidazolium)]-ethane dibromide, 1,3-bis[N-(N′-methylimidazolium)]propane dibromide and 1,4-bis[N-(N′-methylimidazolium)]butane dibromide) for phenol extraction from oil. In this case, a reduction in the extraction of phenol from oil was found as the initial concentration increases from 50 to 200 g L−1.159 However, Fan et al.158 reported no effect of the initial concentration of phenol on its extraction efficiency using 1-butyl-3-(11-hydroxyundecyl)imidazolium tetrafluoroborate.
10.3 Effect of phase ratio
Phase ratio is a crucial parameter in determining the efficiency of the extraction of the phenolic compounds by ILs. This parameter allows the optimum selection of the IL flow rate in any process which leads to improved economics on an industrial scale. Fan et al.166 used various imidazolium-based ILs contain PF6 and BF4 anions and various ranged having an alkyl chain length from butyl ([C4mim]) to octyl ([C8mim]) for phenol extraction. About 1 mL of IL solution was mixed with 5 mL of water solution (W), which gives a phase ratio of 1
:
5 (IL/W). In another study, Deng et al. used [3C6PC14][FeCl4] for phenolic compound extraction using phase ratios from 1
:
40 to 1
:
280 (IL/W). It was found that the optimum phase ratio for the maximum extraction of phenol is obtained at 1
:
120 (IL/W).135 The extraction efficiency decreases after 0.05 mL
:
6 mL and therefore 0.05
:
6 mL was selected as the optimum. Fan et al.158 used hydroxyl, dialkyl, and benzyl ILs in various phase ratios for phenol extraction. By increasing the phase ratio, a decrease in extraction efficiency was observed. Maximum phenol extraction was obtained using a phase ratio 1
:
10 (IL/W). Furthermore, imidazolium and pyrrolidinium based ILs containing NTf2 as anion were used to study the effect of phase ratio on phenol extraction and reported an optimum value of 2
:
3 (IL/W) due to performance and economic values.148. [C6mim][NTf2] and ([C4mim][NTf2]) ILs was also used by Brinda et al.159 to study the effect of phase ratio in the range of 1
:
1, 1
:
2 and 1
:
5 (IL/W) on phenolic extraction from different concentrations of phenolic solutions. The extraction efficiency of phenol was found to decrease as the phase ratio increases from 1
:
3 to 1
:
5. However, no considerable difference was observed for phenol extraction when the phase ratio is between 1
:
1 and 1
:
2, indicating that the ratio of 1
:
2 is the optimum.161
The ratio of the moles of IL to phenol in the aqueous phase was also used to optimize the required amount of IL for certain applications. Hou et al.174 used [C4mim]Cl IL for the extraction of phenol from the aqueous phase. The mole ratio was varied from 0.1 to 3.5. It was found that at mole ratio of 1, 99.0% phenol was extracted, indicating that the optimum mole ratio is obtained at 1. Yao et al.175 used dual functionalized ILs ([Et2NEMPpr][Cl], ([Et2NEmim][Cl] and ([Et2NEMPic][Cl]) for phenol extraction using model oil. It has been observed that equilibrium for all the initial concentrations of 50, 100, and 200 mg L−1 of the phenolic solution is achieved when the IL to phenol mole ratio is 0.3 or more.
10.4 Effect of temperature
Hou et al.159 studied the effect of temperature in the range of 10 °C to 40 °C on phenol extraction from hexane using [C4mim][Cl] and [C4mim][PF6] ILs. The results show that the phenol extraction efficiency decreases with increasing temperature. Specifically, the extraction efficiency decreases from 99.3% to 98.7% for [C4mim][Cl], and from 85.2% to 67.3% for [C4mim][PF6] when temperature increased from 10 °C to 40 °C. Inspection of the above result reveals that the extraction of phenol using [C4mim][PF6] is more sensitive to temperature than that using [C4mim][Cl]. This was attributed to the strong interactions between phenol and [C4mim][Cl] compared to [C4mim][PF6].174 In another study, the effect of temperature on phenol extraction was reported using ILs containing imidazolium cation [C8–10mim] and PF4 and PF6 as anions in the temperature range of 15 °C to 45 °C. It was reported that, in contradiction to a previous study by Hou,159 no effect of temperature on phenol extraction efficiency was observed. Additionally, this observation was supported by a report from Vidal et al.137 who found no effect of temperature on phenol extraction using [C8mim][PF6] and [C8mim][BF4].157 On the other hand, a report by Sas et al.161 using [C4mim][NTf2] and [C6mim][NTf2] ILs for the extraction of phenol at a high and low concentration from aqueous solution indicated that the temperature effect is concentration-dependent. Specifically, no considerable effect of temperature was observed for the high concentration range, whereas for low concentration range, a significant decrease in phenol extraction was observed with increasing temperature. Furthermore, Yao et al.169 reported that, upon using dual functionalized ILs such as [Et2NPpr][Cl], [Et2NEmim][Cl], and [Et2NEMPic][Cl] for extraction of phenol from model oil, the extraction efficiency decreases by 4% upon increasing temperature from 25 °C to 65 °C. This slight decrease in phenol extraction with increasing temperature might be due to the negative enthalpy of extraction which renders the process exothermic.
11. Thermodynamics of phenol extraction
The thermodynamic study of phenol extraction is very important because it reveals the position of equilibrium as well as the spontaneity of the extraction process. Transfer of phenol from aqueous solution or from model oils to ILs phase depends on various types of intermolecular forces such van der Waals, H-bonding, electrostatic, π–π interaction, and hydrophobic interaction.
The distribution of phenol between the aqueous phase and IL phase can be presented by the equilibrium given in eqn (1).
|
Phenol(aq) ↔ phenol(IL)
| (1) |
The equilibrium constant in this case is represented by the distribution coefficient (D) and is given by eqn (2).176,177
|
 | (2) |
The distribution coefficient (D) is calculated using eqn (3).176,177
|
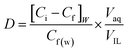 | (3) |
Thermodynamic functions for the extraction process, such as Gibbs free energy change (ΔG), enthalpy change (ΔH), and entropy change (ΔS), determine the driving force involved in the transfer of phenol from aqueous media to IL media. These parameters can be determined using the eqn (4)–(6).
|
ΔG = −RT ln D
| (4) |
Hence
|
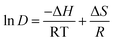 | (6) |
where
R is known as a universal gas constant in J mol
−1 K
−1.
Eqn (6) implies that a plot of ln
D vs. 1/
T will yield a straight line with slope = −Δ
H/
R and intercept = Δ
S/
R.
The value of ΔG is calculated at different temperatures using eqn (4). If the value of ΔG is negative, then the removal of phenol from aqueous media to IL media is spontaneous and feasible under a given set of experimental conditions. It should be noted that eqn (6) was derived based on the assumption that ΔH is temperature independent. However, in practice, ΔH is highly dependent on temperature and it may be negative or positive. Hence, the van't Hoff equation (eqn (7)) should be applied in this case to evaluate its value as a function of temperature.
|
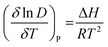 | (7) |
If ΔH is positive, indicating an endothermic process, it means that the extraction of phenol from aqueous to IL media increases with increasing temperature and vice versa.178 This dependence was also found to affect the entropy of the distribution. At around room temperature, its value is positive, indicating that a less ordered structure is presumably obtained in the IL phase. At higher temperatures, ΔS becomes negative, indicating that phenol is trapped within the structured IL, which is most likely leading to the observed decrease in the entropy of the distribution. The extraction of phenol is favored at low temperatures which is economically advantageous. Jiang et al. also observed that the removal of cresol from aqueous media using ILs was exothermic in nature and the distribution coefficient decreasing with increasing temperature.179 In another study, Chen et al. also found that the removal of methylene blue dye from the aqueous phase is exothermic and decreases with an increase in temperature from 20 °C to 45 °C and is accompanied by simultaneous decrease in the distribution coefficient.162,163
12. Phenol extraction from model oil using ILs
Besides the extraction of phenolic compounds from aqueous media, ILs were also used for the extraction of phenolic compounds from model oil. Mostly hydrophilic ILs are used to extract phenolic compounds from model oils. Imidazolium based, 1-ethyl-3-methyl imidazolium lactate ([Emim][Lac]) was used for the extraction of phenol from model oil.180 This IL has the potential to extract phenol from model oil as investigated by COSMO-SAC and demonstrated experimentally. The extraction mechanism shows that the IL extracts phenol via the formation of H-bonds. The extraction efficiency of [Emim][Lac] for phenol reached 99.9% in 30 min at room temperature using [Emim][Lac]/model oil mass ratio of 1
:
1. Amine based ILs, such as propylamine formate ([PA][FA]) and propylamine acetate ([PA][Ac], were synthesized and characterized for the extraction of phenolic compounds from coal tar model oil.180 P-Cresol was extracted by [PA][FA] and [PA][Ac] under different experimental conditions. Among the tested ILs, [PA][FA] has higher efficacy of 97.8% and a distribution coefficient of 27.59 using IL/model oil in 0.2 ratio at room temperature. ILs having dual basic sites were designed by a simple neutralization reaction of 1,1,3,3-tetramethylguanidine acid (TMG) with different acids such as acetic acid, tetrafluoroboric acid, and L-proline.181 The synthesized ILs were tested for phenol extraction from both model oil. Among the tested ILs, 1,1,3,3-tetramethylguanidine tetrafluoroborate ([TMG][BF4]) showed higher extraction efficiency and removed 98.2% phenol in 35 min at room 30 °C. Sidek et al. synthesized IL containing benzyl imidazolium cation with different substituents such as vinyl, ally, and benzyl for extraction of phenolic compounds from hexane as the model oil.182 The effects of process parameters such as phase ratio, contact time, and temperature, were studied and optimized to achieve higher removal efficiency of phenolic compounds. The ILs having allylic substituents were best among the tested ILs and demonstrated 95% efficiency for removal of phenolic compound under optimized conditions. Besides the extraction of phenol from hexane, various other model oils such as ether, heptane, cyclohexane, and petroleum were used. No considerable change in mass and efficiency of ILs were noted after six cycles. Different types of tetraethylammonium amino acid (TAAA) based ILs, having no corrosive halide, were synthesized for the extraction of phenol from the oil mixture.183 The effect of process parameters such as extraction time, phenol concentration, ILs type, types of phenol, water contents in phenol on the extraction of phenol using amino acid-based ILs were investigated. The extraction efficiency of 99.0% was achieved using IL:phenol mole ratio of 0.60. After regeneration and reuse, no decrease in efficiency of ILs for the extraction of phenol was found. Zhuang et al.184 used imidazolium-based ILs such as [C2mim][BF4], [C4mim][BF4], [C4mim][PF6] and [Emim][NTF2] for phenolic compounds extraction from model oil. The effect of the phase volume ratio of ILs to phenol, extraction time (0–80 min), and temperature on extraction efficiency was studied. It was found that all these ILs efficiently removed phenolic compounds from oil. Gai et al.181 reported on the application of ILs containing tetramethylguanidinium cation and anions derived from L-proline, acetic acid, and tetrafluoroboric acid for phenolic compounds extraction from model oil. The results revealed that the order of the efficiency of phenol extraction follow the following order: [BF4]− > [Ac]− > [Pro]−. This difference in extraction efficiency was attributed to differences in anions structure and electronegativity. The lower efficiency of [Pro] anion based ILs was due to its bulky size, which creates steric hindrance towards the interact with phenol.
13. Phenol adsorption on solid supported ILs
Liquid–liquid phase extraction of phenolic compounds from aqueous solutions using hydrophobic ILs has been extensively studied. However, extraction using ILs has serious limitations when applied in practical industrial applications for wastewater treatment. To improve its performance in practical applications, the support of ILs on solid substrates provides a potential practical route that is gaining more focus in separation fields in recent years.185 More specifically, solid-supported ILs for phenolic compounds extraction is also an area of interest. Currently, various researchers have reported the use of supported ILs on different solid supports for the extraction of phenolic compounds. Zhang et al. synthesized novel polymeric ILs using imidazolium monomers.186 The synthesized ILs have a porous structure and Lewis basic active sites which efficiently adsorbed both small and large phenolic molecules from water. The adsorption capacity of tannic acid, 4-nitrophenol, and 4-chlorophenol on the synthesized solid polymeric ILs were 911, 460, and 433 mg g−1, respectively. This higher adsorption capacity of polymeric ILs is attributed to the Lewis basic sites which are due to the N and O atoms. Besides, higher adsorption efficiency for phenolic compounds, the polymeric ILs can be easily regenerated and reused. Another research group has synthesized polystyrene-based resin supported ILs as an adsorbent for the removal of p-nitrophenol from aqueous media.187 The adsorbent was used in batch and continuous flow systems and adsorption efficiency of 1269.8 mg g−1 was achieved within 30 min. Besides promising efficiency for nitrophenol removal, the adsorbent was recycled and reused 10 times without any loss in the adsorption potential for nitrophenol.
Zhu et al. synthesized an IL functionalized polymer by grafting 1-butyl-3-vinylimidazolium bromide, which is used as a monomer, on the surface of silica.188 The silica-supported polymeric material has a rough surface with an area of 205.49 m2 g−1. Grafting ILs onto the silica surface significantly improved the adsorption efficiency for phenolic compounds (2,4-dichlophenol, 4-dinitrophenol, and bisphenol). The kinetic study revealed that the adsorption of phenolic compounds on the solid-supported ILs followed a pseudo-second order kinetic model. The adsorption capacity of this synthesized solid-supported ILs for 2,4-dichlophenol, 2,4-dinitrophenol, bisphenol, and nitrophenol were 239.7, 64.28, 56.86, and 68.39 mg g−1, respectively. Graphene oxide (GO) nanocomposite with 1-amino-3-methylimidazole chloride was synthesized and used for the removal of phenol from aqueous media.189 The GO-IL composite attained a surface area of about 110.44 m2 g−1 and a total pore volume of 0.2839 cm3 g−1. The experimental results showed that the adsorption efficiency of GO-IL nanocomposite for phenol was 95.3%. Layered double hydroxide (LDH) has good potential to adsorb phenolic compounds.190 IL functionalize Zn4Al-LDH was synthesized for the removal of phenol. Zn4Al-LDH was functionalized with Aliquat 336 using a co-precipitation ultrasonication method. Among the various synthesized adsorbents, IL-Zn4Al showed a higher adsorption efficiency (64.7 mg g−1) for phenol adsorption from aqueous media. Polymeric ILs modified with graphene oxide-grafted silica (GO-SiO2) were synthesized for the extraction of phenolic compounds.191 The surface of silica modified with polymeric ILs has higher positive potential and therefore attained strong electrostatic interaction for acidic compounds than the native GO-SiO2. Marwani et al. synthesized192 ILs based solid-supported composite using sol–gel method for phenol adsorption from aqueous media. A new composite material (SiO2-ClPrNTf2) was prepared from silica and chloropromazine bis-(trifluoromethane)sulfonimide for the adsorption of 4-chlorophenol from water. The IL supported solid material has 626.25 mg g−1 efficiency for 4-chlorophenol from water at pH 1. The performance of this IL-based composite was confirmed by applying it to a real sample with satisfactory separation results. Zhu et al.193 synthesized N-butylimidazolium functionalized chloromethylated macroporous styrene-divinylbenzene copolymer with the aim of adsorbing phenol from aqueous solutions. The synthesized IL functionalized polymer can remove phenol from both ion acidic and basic media. The maximum adsorption capacity was 92.9 mg g−1 at pH 11. The adsorption mechanism shows that, in an acidic medium, the adsorption on the surface is mainly molecular, while that in alkaline medium is through anion exchange. Balasubramanian et al.194 prepared emulsified liquid membrane (ELM), by dissolving [Bmim][PF6] in tetrabutyl phosphate for the extraction of phenolic compounds such phenol, p-chlorophenol, 2,4-dichlorophenol, 2,4,6-trichlorophenol and pentachlorophenol from synthetic an aqueous solution. The IL-based ELM has a higher removal efficiency for the extraction of phenolic compounds which follows the order: phenol (99.5%) > p-chlorophenol (95.84) > 2,4-dichlorophenol (93.12%) > 2,4,6-trichlorophenol (91.07%) > pentachlorophenol (90.53%).
Garavand et al. used a microemulsion liquid membrane.195 A microemulsion liquid membrane (MLM) extractor was constructed for the separation and the concentration of phenolic compounds from pistachio peeling effluent water streams. The extraction efficiency was 64% for the MLM compared to 46% for the corresponding emulsion liquid membrane (ELM).
14. Deep eutectic solvents for phenol extraction
Deep eutectic solvents (DESs) have emerged as green solvents to be used as an alternative to the conventional ILs. By definition, a DES is a solvent formed by a combination of proper hydrogen bond donor (HBD) and a hydrogen bond acceptor (HBA), mainly hydrogen bond interaction is the main type of complexation which results in a new solvent having a melting point lower than two components.196–198 DES having properties analogues to conventional ILs, with the advantage of being easily synthesized using low-cost precursors with high purity.199 The interest in using DES has increased due to their greener and environmentally friendly nature, cost-effectiveness, and favorable physicochemical properties. A DES is chemically tunable, so it can be synthesized easily for targeted applications by the proper selection of HBD and HBA. However, most of the DES reported in the literature have a hydrophilic nature and, therefore, cannot be used in aqueous media. This is due to the fact that the strong hydrogen bonding caused by water molecules results in breaking the HBD–HBA complexation and dissolution of the DES components. Consequently, the functionality of the DES is inhibited and this makes it difficult to be separated.199 However, in the last few years, DES having hydrophobic characteristics have emerged as new potential solvents to be used for extracting non-polar and inorganic compounds from aqueous media. It is expected that, in the near future, the use of hydrophobic DES will replace the use of hydrophobic ILs used for the extraction of various pollutants from aqueous solutions.199,200
Florindo synthesized hydrophobic DESs based on a group of fatty acids that may act as HBD and HBA for the removal of bisphenol from aqueous solutions.201 This new DES was prepared by using various types of fatty acids such as octanoic, nonanoic, decanoic, and dodecanoic acid. These fatty acids-based DES are hydrophobic and stable in water. The extraction efficiency of binary and ternary DES was around 92%. Sas et al.202 synthesized various fatty acid-based (dodecanoic acid, decanoic acid, octanoic acid) hydrophobic DES by mixing with menthol or thymol for the extraction of phenolic compounds such as phenol, chlorophenol, and o-cresol from aqueous solution. These hydrophobic DES have a high separation efficiency (about 85%) for all phenolic compounds from the aqueous phase. The extraction efficiency for phenolic compounds followed the order: 2-chlorophenol > o-cresol > phenol.
Adeyemi et al. prepared and characterized seven different types of hydrophobic DES for the extraction of phenolic compounds, such as 3-chlorophenol, 2-chlorophenol, and 2,4-dichlorophenol, from the aqueous phase.203 Various DES based on menthol with hexanoic, octanoic, and decanoic acid were prepared by mixing in 1
:
2 ratio, and menthol–thymol in 1
:
1, 1
:
2,1
:
3, 1
:
4 ratio. All these DES were found to be efficient for the extraction of chlorophenol and about 94% phenol was removed from the aqueous media under optimized conditions. The extraction efficiency of these DES followed the order 3-chlorophenol, 2-chlorophenol, and 2,4-dichlorophenol. In another study, Yang et al.204 prepared binary and ternary DES by mixing two and three carboxylic acids having different chain lengths ranging from C8 to C12. Various types of DES were synthesized by using different types of carboxylic acids and by adjusting their various molar ratios. The ternary DESs containing C8
:
C9
:
C12 mixed in 3
:
2
:
1 molar ratio had a high phenolic compounds removal efficiency of more than 91%. It was found that this DES is very effective, and its efficiency is not influenced by the volume of the sample (>1000 mL). The synthesized DES was very efficient in the removal of the phenolic compound from a large volume of water which indicates their suitability from an industrial point of view. Very recently, hydrophobic DESs were synthesized using methyltrioctylammonium chloride (N8881Cl), tetrabutylammonium chloride (C4444Cl), and menthol as HBA, and octanoic, decanoic, and dodecanoic as HBD. These HDESs were used for the extraction of phenolic compounds from synthetic winery wastewater. Among the tested DES, ammonium-based DESs such as N8881Cl–menthol and N8881Cl–octanoic acid have the highest efficiency for extraction of the phenolic compound from winery wastewater.
Lawal et al.205 have used multiwall carbon nanotubes (CNT) modified with DES for the extraction of phenol from water. The DES was prepared by the combination of methyltriphenylphosphonium bromide and glycerol. The synthesized DES was used for the surface modification of CNT. The DES modified CNT was characterized using FTIR, SEM, and XRD techniques. The phenol adsorption capacity was 298 mg g−1, which is much higher than that of pristine CNT, 128.6 mg g−1.
Gu et al.206 used liquid-phase microextraction for phenolic compounds extraction from model oil method using DES in the presence of ultrasonic waves to shorten the extraction time. DESs were synthesized from choline chloride and α-naphthaleneacetic acid with urea and ethylene glycol. All the DES demonstrated good potential for the extraction of phenol and o-cresol from model oil. Gu et al.206 synthesized DES from various typed quarterly ammonium salt for extraction of the phenolic compound from model oil.207 These DES were synthesized from different types of quaternary ammonium salts such as tetramethylammonium chloride, tetraethylammonium bromide, tetraethylammonium chloride, tetraethylammonium bromide, tetraethylammonium bromide, tetrapropylammonium chloride, tetrabutylammonium chloride, methyltrethylammonium chloride, choline chloride, choline bromide, ammonium chloride. The DESs based on the ammonium salt have higher efficiency for phenol which reached as high as 99.9%. The phenol removal efficiency was not affected by temperature. These DES are easily recycled and reused without a reduction in separation efficiency.207 Pang et al.208 synthesized DES from ammonium salts, especially choline chloride, were found to effectively remove phenolic compounds such phenol and cresol from model oil. Efficient removal of phenolic compounds was achieved in a short time and the removal efficiency of phenolic compounds was not sensitive to temperature. The choline chloride can be easily recycled and reused without weight loss. Yi et al.209 used a DES for the extraction of phenolic compounds from coal-based liquids oils. The DES was synthesized from choline chloride and glycerol and their interaction with model oil was investigated. The effect of the composition of DES, temperature, and DES amount were investigated on the extraction efficiency of phenol. The separation of phenolic compounds was found to take place via hydrogen bonding with DES. The extraction efficiency of phenol from oil was 98.3% under optimized experimental conditions. Yao et al. used quaternary ammonium-based zwitterions (L-carnitine and betaine) to form DES for removing phenol from model oil.210 The effect of process parameters such temperature, extraction time mole ratio of zwitterions to phenol, and initial phenol concentration of phenol on removing efficiency of phenol from model oils were studied and optimized to achieve maximum phenol removal of 94.6%. The DES was also found to be insoluble in the model oil. Very recently, the ammonium salt-containing hydroxyl group (trialkyl-2-3-dihyroypropylammonium chloride) has been used as a liquid eutectic forming salt to extract phenol from toluene.211 Incorporation of alkyl groups and the dihydroxypropyl group to the ammonium cation was found to enhance the extraction efficiency of phenol and also inhibit the miscibility of DESs and toluene. The extraction efficiency of these functionalized DES were greater than choline chloride based DESs for phenol. Yi et al.212 designed DES based on choline chloride and glycerol (1
:
1) for dephenolization of model oil. Choline based DES have been shown to have high efficiency for extraction of phenolic compounds from coal-based liquids phenol. The higher efficiency of choline based DES is due to the presence of the hydroxyl group, the short alkyl chain, and the small central cation atom. Three imidazolium-based diatonic ILs were synthesized and used for the formation of DES for the separation of phenolic compound from an oil mixture.213 1,2-Bis[N-(N-methylimidazolium)]ethane dibromide (DIL1), 1,3-bis[N-(N-methylimidazolium)]propane dibromide (DIL2), 1,4-bis[N-(N-methylimidazolium)]butane dibromide (DIL3) were the ILs synthesized for phenolic compound extraction from oil mixtures. All these ILs have high efficiency (96.6%) for extraction of phenolic compounds and the order of phenol extraction was found to be DIL1 < DIL2 < DIL3. These dicationic ILs are thermally stable and can be used over a broad temperature range. These ILs were recycled and reused without any change in their structure after four cycle. DIL3 was also used for extraction of phenolic compounds from coal tar with a removal efficiency of 93.1%.
15. Conclusions
In comparison to traditional solvents, ILs are alternative solvents known as greener solvents because of their negligible vapor pressure and reduced impact on the environment and human health. The structural characteristics of ILs such as anion type, cation type, alkyl chain length, and functional groups play a key role in their performance as phenol extractants. ILs having hydrophobic anions and aromatic cations are more efficient for phenol extraction. As far as the alkyl chain length on cation is concerned, it has been observed that increasing the hydrophobicity of ILs by increasing alkyl chain length has no considerable effect on the extraction of phenolic compounds. A wide range of ILs with desirable properties can be synthesized by selecting proper anions and cations which may offer great potential for the extraction of phenolic compounds. Moreover, the experimental parameters such as initial pH of the phenol solution, phase ratio (VIL
:
Vw), phenol concentration, and temperature each play a vital role in phenol extraction; therefore, their optimization is necessary to achieve maximum extraction of phenol. Phenol extraction efficiency increasing with increase in pH of phenol solution from acidic to basic, contact time and initial phenol concentration. By increasing the phase ratio, a decrease in phenol extraction efficiency was observed. Solid supported ILs are consider better for phenol extraction from aqueous and model oils due to their ease recyclability and reusability. As a result, ILs technology provides an opportunity to develop novel and improved methods for phenol removal from wastewater. In this context, task specific ILs will play a crucial role in wastewater processing technology. DESs in their pristine form, or as surface modifiers for nano-materials, were also explored for their applications in the treatment of fluidic waste either. These solvents were used successfully for the treatment of aqueous and non-aqueous waste fluids contaminated with phenolic compounds. These solvents have great industrial potential as alternatives for ILs.
Finally, it is hoped that this paper may provide valuable and useful data for researchers and industrialists working to develop novel cleaner processes using ionic liquids. It is anticipated also that these novel cleaner processes for wastewater treatment containing phenolic compounds will enhance both the sustainability and innovation in the relevant industries.
Abbreviations
EPA | Environmental Protection Agency |
ppb | Part per billion |
ppm | Part per million |
ILs | Ionic liquids |
ΔHvap | Heat of vaporization |
CO2 | Carbon dioxide |
H2S | Hydrogen sulfide |
SO2 | Sulphur dioxide |
BF4− | Tetrafluoroborate |
PF6− | Hexafluorophosphate |
Cl− | Chloride |
Br− | Bromide |
I− | Iodide |
HCOO− | Formate |
CF3COO− | Trifluoroacetate |
CH3COO− | Acetate |
HSO4− | Hydrogen sulphate |
CF3SO3− | Trifluoromethanesulfonate |
CH3SO3− | Methanesulfonate |
NTf2 | Bis-(trifluoromethanesufonyl)amide |
NO3− | Nitrate |
AlCl4− | Tetrachloroaluminate |
SCN− | Thiocyanate |
H2PO4− | Dihydrogen phosphate |
CF3SO3− | Trifluoromethanesulfonate |
CF3COO− | Trifluoroacetate |
Ac | Acetate |
Pro | Propionate |
[C3mim][NTf2] | 1-Methyl-3-propylimidazolium bis(trifluoromethylsulfonyl)imide |
[C4mim][NTf2] | 1-Butyl-3-methylimidazoliumbis(trifluoromethylsulfonyl)imide |
[C6mim][NTf2] | 1-Hexyl-3-methylimidazolium bis(trifluoromethylsulfonyl)imide |
[C6mim][BF4] | 1-Methyl-3-hexylimidazolium tetrafluoroborate |
[C6mim][PF6] | 1-Methyl-3-hexylimidazolium hexafluorophosphate |
[C8mim][BF4] | 1-Methyl-3-octylimidazolium tetrafluoroborate |
[C8mim][PF6] | 1-Methyl-3-octylimidazolium hexafluorophosphate |
[N2222]+ | Tetraethyl ammonium |
[N2222][L-Pro] | Tetraethyl ammonium prolinate |
[N2222][Ser] | Tetraethyl ammonium serine |
[N2222][Gly] | Tetraethyl ammonium glycine |
[N2222][L-Ala] | Tetraethyl ammonium alanine |
[N2222][L-Lys] | Tetraethyl ammonium lysine |
[C4C1Py]− | 3-Methyl-1-butylpyridinium |
MEA | Monoethanol amines |
DEA | Diethanol amines |
TEA | Triethanol amines |
C4Py+ | Butylpyridinium |
C4C1Py+ | 1-Methyl-3-butylpyridinium |
C4C1Pip+ | 1-Methyl-3-butylpyyrlidinium |
[C6mPyr][NTf2] | 1-Methyl-3-hexylpyridinium bis-(trifluoromethanesulfonyl)amide |
[C6mim][NTf2] | 1-Methyl-3-hexylimidazolium bis-(trifluoromethanesulfonyl)amide |
THADHSS | Tetrahexylammonium dihexylsulfosuccinate |
TOMAS | Trioctylmethylammonium salicylate |
[C8mim][NTf2] | 1-Methyl-3-octylimidazolium bis-(trifluoromethanesulfonyl)amide |
[C4C7im][NTf2] | 1-Methyl-3-heptylimidazolium bis-(trifluoromethanesulfonyl)amide |
[C4C9im][NTf2] | 1-Methyl-3-heptylimidazolium bis-(trifluoromethanesulfonyl)amide |
[C4C12im][NTf2] | 1-Methyl-3-dodecylimidazolium bis-trifluoromethanesulfonyl)amide |
[C8mim][PF6] | 1-Methyl-3-octylimidazolium hexafluorophosphate |
[C4C7im][PF6] | 1-Methyl-3-heptylimidazolium hexafluorophosphate |
[C4C9im][PF6] | 1-Methyl-3-heptylimidazolium hexafluorophosphate |
[C4C12im][PF6] | 1-Methyl-3-dodecylimidazolium hexafluorophosphate |
[C8mim][BF4] | 1-Methyl-3-octylimidazolium tetrafluoroborate |
[C4C7im][BF4] | 1-Methyl-3-heptylimidazolium tetrafluoroborate |
[C4C9im][BF4] | 1-Methyl-3-dodecylimidazolium tetrafluoroborate |
[C4C12im][BF4] | 1-Methyl-3-dodecylimidazolium tetrafluoroborate |
[C4C6OHim][NTf2] | 1-Butyl-3-(6-hydroxyhexyl)imidazolium bis-trifluoromethanesulfonyl)amide |
[C4C8OHim][NTf2] | 1-Butyl-3-(8-hydroxyoctyl)imidazolium bis-trifluoromethanesulfonyl)amide |
[C4C11OHim][NTf2] | 1-Butyl-3-(11-hydroxyundecyl)imidazolium bis-trifluoromethanesulfonyl)amide |
[C4C6OHim][PF6] | 1-Butyl-3-(6-hydroxyhexyl)imidazolium hexafluorophosphate |
[C4C8OHim][PF6] | 1-Butyl-3-(8-hydroxyoctyl) imidazolium hexafluorophosphate |
[C4C11OHim][PF6] | 1-Butyl-3-(11-hydroxyundecyl) imidazolium hexafluorophosphate |
[C4C8OHim][BF4] | 1-Butyl-3-(8-hydroxyoctyl)imidazolium tetrafluoroborate |
[C4C11OHim][BF4] | 1-Butyl-3-(11-hydroxyundecyl) imidazolium tetrafluoroborate |
[C4Beim][BF4] | 1-Butyl-3-benzylimidazolium tetrafluoroborate |
[C4Beim][NTf2] | 1-Butyl-3-benzylimidazolium bis-trifluoromethanesulfonyl)amide |
[3C6PC14][FeCl4] | Trihexyltetradecylphosphonium tetrachloroferrate |
[HMEA][HCOO] | Monethanolammonium formate |
[HDEA][HCOO] | Diethanolammonium formate |
[HTEA][HCOO] | Triethanolammonium formate |
[HMEA][Ac] | Monethanolammonium acetate |
[HDEA][Ac] | Diethanolammonium acetate |
[HTEA][Ac] | Triethanolammonium acetate |
[ABZIM][Cl] | 1-Allyl-3-benzylimidazolium chloride |
[DBZIM][Cl] | 1,3-Dibenzylimidazoilum chloride |
[BZVIM][Cl] | 1-Benzyl-3-vinylimidazolium chloride |
[Et2NEmim][Cl]2 | 1,(2-(Diethylamino)ethyl)-3-methyl imidazolium chloride |
[Et2NEmmor][Cl]2 | 1,(2-(Diethylamino)ethyl)-3-methyl morpholinium chloride |
[Et2NEmpyr][Cl]2 | 1,(2-(Diethylamino)ethyl)-3-methyl pyrrolidinium chloride |
[Et2NEmpic][Cl]2 | 1,(2-(Diethylamino)ethyl)-3-methyl pyridinium chloride |
D | Distribution coefficient |
ΔG | Change in Gibbs free energy |
ΔH | Change in enthalpy |
ΔS | Change in entropy |
R | Universal gas constant |
[Emim][Lac] | 1-Ethyl-3-methyl imidazolium lactate |
[PA][FA] | Propylamine formate |
[PA][Ac] | Propylamine acetate |
MG | 1,1,3,3-Tetramethylguanidine acid |
[TMG][BF4] | 1,1,3,3-Tetramethylguanidine tetrafluoroborate |
TAAA | Tetraethylammonium amino acid |
GO | Graphene oxide |
LDH | Layered double hydroxide |
ELM | Emulsified liquid membrane |
DESs | Deep eutectic solvents |
HBD | Hydrogen bond donor |
HBA | Hydrogen bond acceptor |
CNT | Carbon nanotubes |
Conflicts of interest
We wish to confirm that no conflict of interest associated with this publication.
Acknowledgements
This work was supported by the American University of Sharjah under grant no. FRG19-L-E13.
References
- W. H. Organization, Global strategy on human resources for health: workforce 2030, 2016 Search PubMed.
- K. Zatloukalová, L. Obalová, K. Kočí, L. Čapek, Z. Matěj and H. Šnajdhaufová, et al., Photocatalytic Degradation of Endocrine Disruptor Compounds in Water over Immobilized TiO2 Photocatalysts, Iran. J. Chem. Chem. Eng., 2017, 36, 29–38 Search PubMed.
- L. Lu, J. Li, D. H. Ng, P. Yang, P. Song and M. Zuo, Synthesis of novel hierarchically porous Fe3O4@MgAl-LDH magnetic microspheres and its superb adsorption properties of dye from water, J. Ind. Eng. Chem., 2017, 46, 315–323 CrossRef CAS.
- A. Nasrullah, B. Saad, A. Bhat, A. S. Khan, M. Danish and M. H. Isa, et al., Mangosteen peel waste as a sustainable precursor for high surface area mesoporous activated carbon: Characterization and application for methylene blue removal, J. Cleaner Prod., 2019, 211, 1190–1200 CrossRef CAS.
- A. Nasrullah, H. Khan, A. S. Khan, N. Muhammad, Z. Man and F. U. Khan, et al., Calligonum polygonoides biomass as a low-cost adsorbent: surface characterization and methylene blue adsorption characteristics, Desalin. Water Treat., 2016, 57, 7345–7357 CrossRef CAS.
- G. Busca, S. Berardinelli, C. Resini and L. Arrighi, Technologies for the removal of phenol from fluid streams: a short review of recent developments, J. Hazard. Mater., 2008, 160, 265–288 CrossRef CAS PubMed.
- Y. Hou, Y. Ren, W. Peng, S. Ren, W. J. I. Wu and E. C. Research, Separation of phenols from oil using imidazolium-based ionic liquids, Ind. Eng. Chem. Res., 2013, 52, 18071–18075 CrossRef CAS.
- O. G. Sas, P. B. Sánchez, B. González, Á. J. S. Domínguez and P. Technology, Removal of phenolic pollutants from wastewater streams using ionic liquids, Sep. Purif. Technol., 2020, 236, 116310 CrossRef CAS.
- R. Kristoffersson, S. Broberg and A. Oikari, Physiological effects of a sublethal concentration of phenol in the pike (Esox lucius L.) in pure brackish water, Ann. Zool. Fenn., 1973, 392–397 Search PubMed.
- D. Debadatta and M. Susmita, Study of individual and simultaneous degradation of chromium (vi) and phenol using two potent indigenous microorganisms, J. Environ. Res. Dev., 2015, 9, 530 CAS.
- R. Aghav, S. Kumar and S. Mukherjee, Artificial neural network modeling in competitive adsorption of phenol and resorcinol from water environment using some carbonaceous adsorbents, J. Hazard. Mater., 2011, 188, 67–77 CrossRef CAS PubMed.
- A. Brinda Lakshmi, A. Balasubramanian and S. J. C. S. Venkatesan, Extraction of phenol and chlorophenols using ionic liquid [Bmim]+[BF4]− dissolved in tributyl phosphate, Clean: Soil, Air, Water, 2013, 41, 349–355 CAS.
- Y. Chen, Y. Meng, J. Yang, H. Li, X. J. J. o. C. Liu and E. Data, Phenol distribution behavior in aqueous biphasic systems composed of ionic liquids–carbohydrate–water, J. Chem. Eng. Data, 2012, 57, 1910–1914 CrossRef CAS.
- Y. S. Ng, N. Jayakumar and M. A. J. D. Hashim, Behavior of hydrophobic ionic liquids as liquid membranes on phenol removal: Experimental study and optimization, Desalination, 2011, 278, 250–258 CrossRef CAS.
- J. E. Amoore and E. Hautala, Odor as an ald to chemical safety: odor thresholds compared with threshold limit values and volatilities for 214 industrial chemicals in air and water dilution, J. Appl. Toxicol., 1983, 3, 272–290 CrossRef CAS PubMed.
- L. Barros, M. Dueñas, I. C. Ferreira, P. Baptista and C. Santos-Buelga, Phenolic acids determination by HPLC-DAD-ESI/MS in sixteen different Portuguese wild mushrooms species, Food Chem. Toxicol., 2009, 47, 1076–1079 CrossRef CAS PubMed.
- K. Shaw and P. Wall, The phenolic acids of human urine. Paper chromatography of phenolic acids, J. Biol. Chem., 1956, 218, 293 CrossRef.
- W. Jordan, H. van Barneveld, O. Gerlich, M. Kleine-Boymann and J. Ullrich, Phenol, Ullmann's Encycl. Ind. Chem., 2000 Search PubMed.
- R. J. Schmidt, Industrial catalytic processes—phenol production, Appl. Catal., A, 2005, 280, 89–103 CrossRef CAS.
- G. Busca, Acid catalysts in industrial hydrocarbon chemistry, Chem. Rev., 2007, 107, 5366–5410 CrossRef CAS PubMed.
- H.-G. Franck and J. W. Stadelhofer, Industrial aromatic chemistry: raw materials·processes· products, Springer Science & Business Media, 2012 Search PubMed.
- H. A. Wittcoff, B. G. Reuben and J. S. Plotkin, Industrial organic chemicals, John Wiley & Sons, 2012 Search PubMed.
- D. M. Naguib and N. M. Badawy, Phenol removal from wastewater using waste products, J. Environ. Chem. Eng., 2020, 8, 103592 CrossRef CAS.
- H. Gai, X. Zhang, S. Chen, C. Wang, M. Xiao and T. Huang, et al., An improved tar–water separation process of low–rank coal conversion wastewater for increasing the tar yield and reducing the oil content in wastewater, Chem. Eng. J., 2020, 383, 123229 CrossRef CAS.
- Y. N. Dhoble and S. Ahmed, Treatment of wastewater generated from coke oven by adsorption on steelmaking slag and its effect on cementitious properties, Curr. Sci., 2019, 116, 1346 CrossRef CAS.
- A. Akyol, B. Samuk, M. Kobya and E. Demirbas, Treatment of phenol formaldehyde production wastewater by electrooxidation-electrofenton successive processes, Sep. Sci. Technol., 2019, 1–14 Search PubMed.
- N. Ghimire and S. Wang, Biological treatment of petrochemical wastewater, in Petroleum Chemicals-Recent Insight, IntechOpen, 2018 Search PubMed.
- D. Yaseen and M. Scholz, Textile dye wastewater characteristics and constituents of synthetic effluents: a critical review, Int. J. Environ. Sci. Technol., 2019, 16, 1193–1226 CrossRef CAS.
- N. Pradeep, S. Anupama, K. Navya, H. Shalini, M. Idris and U. Hampannavar, Biological removal of phenol from wastewaters: a mini review, Appl. Water Sci., 2015, 5, 105–112 CrossRef CAS.
- N. Sivaram and D. Barik, Toxic Waste From Leather Industries, in Energy from Toxic Organic Waste for Heat and Power Generation, Elsevier, 2019, pp. 55–67 Search PubMed.
- N. K. Swamy, P. Singh and I. P. Sarethy, Precipitation of phenols from paper industry wastewater using ferric chloride, Rasayan J. Chem., 2011, 4, 452–456 CAS.
- M. Aboulhassan, S. Souabi, A. Yaacoubi and M. Baudu, Treatment of paint manufacturing wastewater by the combination of chemical and biological processes, Int. J. Environ. Sci. Technol., 2014, 3, 1747–1758 Search PubMed.
- K. W. Taylor and R. Chénier, Introduction to ecological risk assessments of priority substances under the Canadian Environmental Protection Act, 1999, Hum. Ecol. Risk Assess., 2003, 9, 447–451 CrossRef.
- W. Bruce, M. Meek and R. Newhook, Phenol: hazard characterization and exposure–response analysis, J. Environ. Sci. Health, Part C: Toxicol. Carcinog., 2001, 19, 305–324 CrossRef.
- M. Alshabib and S. A. Onaizi, A review on phenolic wastewater remediation using homogeneous and heterogeneous enzymatic processes: Current status and potential challenges, Separation and Purification Technology, 2019 Search PubMed.
- S. J. Kulkarni and J. P. Kaware, Review on research for removal of phenol from wastewater, Int. J. Sci. Res., 2013, 3, 1–5 Search PubMed.
- L. G. C. Villegas, N. Mashhadi, M. Chen, D. Mukherjee, K. E. Taylor and N. Biswas, A short review of techniques for phenol removal from wastewater, Curr. Pollut. Rep., 2016, 2, 157–167 CrossRef CAS.
- W. Kujawski, A. Warszawski, W. Ratajczak, T. Porębski, W. Capała and I. Ostrowska, Removal of phenol from wastewater by different separation techniques, Desalination, 2004, 163, 287–296 CrossRef CAS.
- M. Iqbal and I. A. Bhatti, Re-utilization option of industrial wastewater treated by advanced oxidation process, Pak. J. Agric. Sci., 2014, 51, 1141–1147 Search PubMed.
- D. Mohan, K. P. Singh, G. Singh and K. Kumar, Removal of dyes from wastewater using flyash, a low-cost adsorbent, Ind. Eng. Chem. Res., 2002, 41, 3688–3695 CrossRef CAS.
- E. Pajootan, M. Arami and N. M. Mahmoodi, Binary system dye removal by electrocoagulation from synthetic and real colored wastewaters, J. Taiwan Inst. Chem. Eng., 2012, 43, 282–290 CrossRef CAS.
- M. Kobya, M. Bayramoglu and M. Eyvaz, Techno-economical evaluation of electrocoagulation for the textile wastewater using different electrode connections, J. Hazard. Mater., 2007, 148, 311–318 CrossRef CAS PubMed.
- Ö. Y. Balik and S. Aydin, Coagulation/flocculation optimization and sludge production for pre-treatment of paint industry wastewater, Desalin. Water Treat., 2016, 57, 12692–12699 CrossRef.
- N. Bilal, S. Ali and M. Iqbal, Application of advanced oxidations processes for the treatments of textile effluents, Asian J. Chem., 2014, 26, 1882 CrossRef CAS.
- M. A. Jamal, M. Muneer and M. Iqbal, Photo-degradation of monoazo dye blue 13 using advanced oxidation process, Chem. Int., 2015, 1, 12–16 Search PubMed.
- B. Merzouk, M. Yakoubi, I. Zongo, J.-P. Leclerc, G. Paternotte and S. Pontvianne, et al., Effect of modification of textile wastewater composition on electrocoagulation efficiency, Desalination, 2011, 275, 181–186 CrossRef CAS.
- T. A. Khan and E. A. Khan, Removal of basic dyes from aqueous solution by adsorption onto binary iron-manganese oxide coated kaolinite: non-linear isotherm and kinetics modeling, Appl. Clay Sci., 2015, 107, 70–77 CrossRef CAS.
- H. Shon, S. Phuntsho, D. Chaudhary, S. Vigneswaran and J. Cho, Nanofiltration for water and wastewater treatment-a mini review, Drinking Water Eng. Sci., 2013 Search PubMed.
- A. Jaradat, S. Gharaibeh and M. Abu Irjei, The application of solar distillation technique as a mean for olive mill wastewater management, Water Environ. J., 2018, 32, 134–140 CrossRef CAS.
- U. Shamraiz, R. A. Hussain, A. Badshah, B. Raza and S. Saba, Functional metal sulfides and selenides for the removal of hazardous dyes from Water, J. Photochem. Photobiol., B, 2016, 159, 33–41 CrossRef CAS PubMed.
- R. F. Gomes, A. C. N. de Azevedo, A. G. Pereira, E. C. Muniz, A. R. Fajardo and F. H. Rodrigues, Fast dye removal from water by starch-based nanocomposites, J. Colloid Interface Sci., 2015, 454, 200–209 CrossRef CAS PubMed.
- B. Mu and A. Wang, Adsorption of dyes onto palygorskite and its composites: a review, J. Environ. Chem. Eng., 2016, 4, 1274–1294 CrossRef CAS.
- R. Srinivasan, Advances in application of natural clay and its composites in removal of biological, organic, and inorganic contaminants from drinking water, Adv. Mater. Sci. Eng., 2011, 2011 Search PubMed.
- M. A. Tahir, H. N. Bhatti and M. Iqbal, Solar Red and Brittle Blue direct dyes adsorption onto Eucalyptus angophoroides bark: Equilibrium, kinetics and thermodynamic studies, J. Environ. Chem. Eng., 2016, 4, 2431–2439 CrossRef.
- G. Crini, Non-conventional low-cost adsorbents for dye removal: a review, Bioresour. Technol., 2006, 97, 1061–1085 CrossRef CAS PubMed.
- E. Hassan, Comparative study on the biosorption of Pb (II), Cd (II) and Zn (II) using Lemon grass (Cymbopogon citratus): kinetics, isotherms and thermodynamics, Chem. Int., 2016, 2, 89–102 Search PubMed.
- A. Babarinde and G. O. Onyiaocha, Equilibrium sorption of divalent metal ions onto groundnut (Arachis hypogaea) shell: kinetics, isotherm and thermodynamics, Chem. Int., 2016, 2, 37–46 CAS.
- S. P. Buthelezi, A. O. Olaniran and B. Pillay, Textile dye removal from wastewater effluents using bioflocculants produced by indigenous bacterial isolates, Molecules, 2012, 17, 14260–14274 CrossRef CAS PubMed.
- N. Rao, J. R. Singh, R. Misra and T. Nandy, Liquid–liquid extraction of phenol from simulated sebacic acid wastewater, 2009 Search PubMed.
- C. Zidi, R. Tayeb, M. B. S. Ali and M. Dhahbi, Liquid–liquid extraction and transport across supported liquid membrane of phenol using tributyl phosphate, J. Membr. Sci., 2010, 360, 334–340 CrossRef CAS.
- Z. Ullah, M. A. Bustam, Z. Man and A. S. Khan, Phosphonium-based ionic liquids and their application in separation of dye from aqueous solution, ARPN J. Eng. Appl. Sci., 2016, 11, 1653–1659 CAS.
- T. Vatai, M. Škerget and Ž. Knez, Extraction of phenolic compounds from elder berry and different grape marc varieties using organic solvents and/or supercritical carbon dioxide, J. Food Eng., 2009, 90, 246–254 CrossRef CAS.
- C. F. Poole and S. K. Poole, Extraction of organic compounds with room temperature ionic liquids, J. Chromatogr. A, 2010, 1217, 2268–2286 CrossRef CAS PubMed.
- A. S. Khan, A. Nasrullah, Z. Ullah, A. Bhat, O. B. Ghanem and N. Muhammad, et al., Thermophysical properties and ecotoxicity of new nitrile functionalised protic ionic liquids, J. Mol. Liq., 2018, 249, 583–590 CrossRef CAS.
- M. T. Yagub, T. K. Sen, S. Afroze and H. M. Ang, Dye and its removal from aqueous solution by adsorption: a review, Adv. Colloid Interface Sci., 2014, 209, 172–184 CrossRef CAS PubMed.
- W. W. Anku, M. A. Mamo and P. P. Govender, Phenolic compounds in water: sources, reactivity, toxicity and treatment methods, Phenolic compounds-natural sources, importance and applications, 2017, pp. 420–443 Search PubMed.
- M. Baker and C. Mayfield, Microbial and non-biological decomposition of chlorophenols and phenol in soil, Water, Air, Soil Pollut., 1980, 13, 411–424 CrossRef CAS.
- A. Kumar, S. Kumar and S. Kumar, Biodegradation kinetics of phenol and catechol using Pseudomonas putida MTCC 1194, Biochem. Eng. J., 2005, 22, 151–159 CrossRef CAS.
- S. Poirier, A. Bize, C. Bureau, T. Bouchez and O. Chapleur, Community shifts within anaerobic digestion microbiota facing phenol inhibition: towards early warning microbial indicators?, Water Res., 2016, 100, 296–305 CrossRef CAS PubMed.
- H. Arai, T. Ohishi, M. Y. Chang and T. Kudo, Arrangement and regulation of the genes for meta-pathway enzymes required for degradation of phenol in Comamonas testosteroni TA441, Microbiology, 2000, 146, 1707–1715 CrossRef CAS PubMed.
- M. Xiao, H. Ma, M. Sun, X. Yin, Q. Feng and H. Song, et al., Characterization of cometabolic degradation of p-cresol with phenol as growth substrate by Chlorella vulgaris, Bioresour. Technol., 2019, 281, 296–302 CrossRef CAS PubMed.
- M. Y. Kilic, W. H. Abdelraheem, X. He, K. Kestioglu and D. D. Dionysiou, Photochemical treatment of tyrosol, a model phenolic compound present in olive mill wastewater, by hydroxyl and sulfate radical-based advanced oxidation processes (AOPs), J. Hazard. Mater., 2019, 367, 734–742 CrossRef CAS PubMed.
- S. Mohammadi, A. Kargari, H. Sanaeepur, K. Abbassian, A. Najafi and E. Mofarrah, Phenol removal from industrial wastewaters: a short review, Desalin. Water Treat., 2015, 53, 2215–2234 CrossRef CAS.
- G. Loos, T. Scheers, K. Van Eyck, A. Van Schepdael, E. Adams and B. Van der Bruggen, et al., Electrochemical oxidation of key pharmaceuticals using a boron doped diamond electrode, Sep. Purif. Technol., 2018, 195, 184–191 CrossRef CAS.
- N. Rosman, W. N. W. Salleh, A. F. Ismail, J. Jaafar, Z. Harun and F. Aziz, et al., Photocatalytic degradation of phenol over visible light active ZnO/Ag2CO3/Ag2O nanocomposites heterojunction, J. Photochem. Photobiol., A, 2018, 364, 602–612 CrossRef CAS.
- T. J. Bandosz, A. Policicchio, M. Florent, W. Li, P. S. Poon and J. Matos, Solar light-driven photocatalytic degradation of phenol on S-doped nanoporous carbons: The Role of functional groups in governing activity and selectivity, Carbon, 2020, 156, 10–23 CrossRef CAS.
- M. G. Alalm, A. Tawfik and S. Ookawara, Investigation of optimum conditions and costs estimation for degradation of phenol by solar photo-Fenton process, Appl. Water Sci., 2017, 7, 375–382 CrossRef.
- R. Underhill, R. J. Lewis, S. J. Freakley, M. Douthwaite, P. J. Miedziak and O. Akdim, et al., Oxidative degradation of phenol using in situ generated hydrogen peroxide combined with Fenton's process, Johnson Matthey Technol. Rev., 2018, 62, 417–425 CrossRef CAS.
- G. La Scalia, R. Micale, L. Cannizzaro and F. P. Marra, A sustainable phenolic compound extraction system from olive oil mill wastewater, J. Cleaner Prod., 2017, 142, 3782–3788 CrossRef CAS.
- E. Hernández-Francisco, J. Peral and L. Blanco-Jerez, Removal of phenolic compounds from oil refinery wastewater by electrocoagulation and Fenton/photo-Fenton processes, J. Water Process. Eng., 2017, 19, 96–100 CrossRef.
- W. Gao and P. Fatehi, Fly ash based adsorbent for treating bleaching effluent of kraft pulping process, Sep. Purif. Technol., 2018, 195, 60–69 CrossRef CAS.
- G. Li, Q. Xu, X. Jin, R. Li, R. Dharmarajan and Z. Chen, Enhanced adsorption and Fenton oxidation of 2,4-dichlorophenol in aqueous solution using organobentonite supported nZVI, Sep. Purif. Technol., 2018, 197, 401–406 CrossRef CAS.
- L. Zhou, H. Cao, C. Descorme and Y. Xie, Phenolic compounds removal by wet air oxidation based processes, Front. Environ. Sci. Eng., 2018, 12(1), 1–20 CrossRef CAS.
- O. G. Sas, I. Domínguez, B. González and Á. Domínguez, Liquid-liquid extraction of phenolic compounds from water using ionic liquids: Literature review and new experimental data using [C2mim] FSI, J. Environ. Manage., 2018, 228, 475–482 CrossRef CAS PubMed.
- K. Abbassian, A. Kargari and T. Kaghazchi, Phenol removal from aqueous solutions by a novel industrial solvent, Chem. Eng. Commun., 2015, 202, 408–413 CrossRef CAS.
- G. Gonfa, M. A. Bustam, N. Muhammad and A. S. Khan, Evaluation of thermophysical properties of functionalized imidazolium thiocyanate based ionic liquids, Ind. Eng. Chem. Res., 2015, 54, 12428–12437 CrossRef CAS.
- A. S. Khan, Z. Man, A. Arvina, M. A. Bustam, A. Nasrullah and Z. Ullah, et al., Dicationic imidazolium based ionic liquids: Synthesis and properties, J. Mol. Liq., 2017, 227, 98–105 CrossRef CAS.
- A. S. Khan, Z. Man, M. A. Bustam, C. F. Kait, A. Nasrullah and Z. Ullah, et al., Dicationic ionic liquids as sustainable approach for direct conversion of cellulose to levulinic acid, J. Cleaner Prod., 2018, 170, 591–600 CrossRef CAS.
- A. S. Khan, A. Nasrullah, Z. Ullah, A. H. Bhat, O. B. Ghanem and N. Muhammad, et al., Thermophysical properties and ecotoxicity of new nitrile functionalised protic ionic liquids, J. Mol. Liq., 2018, 249, 583–590 CrossRef CAS.
- Z. Ullah, M. A. Bustam, Z. Man, S. N. Shah, A. S. Khan and N. Muhammad, Synthesis, characterization and physicochemical properties of dual-functional acidic ionic liquids, J. Mol. Liq., 2016, 223, 81–88 CrossRef CAS.
- Z. Ullah, M. A. Bustam, N. Muhammad, Z. Man and A. S. Khan, Synthesis and thermophysical properties of hydrogensulfate based acidic ionic liquids, J. Solution Chem., 2015, 44, 875–889 CrossRef CAS.
- Q. Li, J. Jiang, G. Li, W. Zhao, X. Zhao and T. Mu, The electrochemical stability of ionic liquids and deep eutectic solvents, Sci. China: Chem., 2016, 59, 571–577 CrossRef CAS.
- Z. Xue, L. Qin, J. Jiang, T. Mu and G. Gao, Thermal, electrochemical and radiolytic stabilities of ionic liquids, Phys. Chem. Chem. Phys., 2018, 20, 8382–8402 RSC.
- N. Muhammad, Z. Man, A. K. Ziyada, M. A. Bustam, M. A. Mutalib and C. D. Wilfred, et al., Thermophysical properties of dual functionalized imidazolium-based
ionic liquids, J. Chem. Eng. Data, 2012, 57, 737–743 CrossRef CAS.
- Z. Rashid, C. D. Wilfred, N. Gnanasundaram, A. Arunagiri and T. Murugesan, A comprehensive review on the recent advances on the petroleum asphaltene aggregation, J. Pet. Sci. Eng., 2019, 176, 249–268 CrossRef CAS.
- Z. Rashid, C. D. Wilfred, R. Iyyaswami, A. Appusamy and M. Thanabalan, Investigating the solubility of petroleum asphaltene in ionic liquids and their interaction using COSMO-RS, J. Ind. Eng. Chem., 2019, 79, 194–203 CrossRef CAS.
- M. N. Safiah, B. M. Azmi and M. Y. Normawati, CO2 capture using silica and molecular sieve impregnated with [hmim][Tf2N], Int. J. Chem. Eng. Appl., 2014, 5, 342 CAS.
- M. Zia ul Mustafa, H. bin Mukhtar, N. A. H. Md Nordin, H. A. Mannan, R. Nasir and N. Fazil, Recent Developments and Applications of Ionic Liquids in Gas Separation Membranes, Chem. Eng. Technol., 2019, 42, 2580–2593 CrossRef CAS.
- A. Sarwono, Z. Man, N. Muhammad, A. S. Khan, W. S. W. Hamzah and A. H. A. Rahim, et al., A new approach of probe sonication assisted ionic liquid conversion of glucose, cellulose and biomass into 5-hydroxymethylfurfural, Ultrason. Sonochem., 2017, 37, 310–319 CrossRef CAS PubMed.
- Z. Ullah, M. Azmi Bustam, N. Muhammad, Z. Man and A. S. Khan, Synthesis and Thermophysical Properties of Hydrogensulfate Based Acidic Ionic Liquids, J. Solution Chem., 2015, 44, 875–889 CrossRef CAS.
- Z. Ullah, M. A. Bustam, Z. Man, N. Muhammad and A. S. Khan, Synthesis, characterization and the effect of temperature on different physicochemical properties of protic ionic liquids, RSC Adv., 2015, 5, 71449–71461 RSC.
- M. A. B. Zahoor Ullah, Z. Man and A. S. Khan, Phosphonium-based ionic liquids and their application in separation of dye from aqueous solution, ARPN J. Eng. Appl. Sci., 2016, 11, 1653–1659 Search PubMed.
- T. Payagala, J. Huang, Z. S. Breitbach, P. S. Sharma and D. W. Armstrong, Unsymmetrical dicationic ionic liquids: manipulation of physicochemical properties using specific structural architectures, Chem. Mater., 2007, 19, 5848–5850 CrossRef CAS.
- A. M. Asim, M. Uroos, S. Naz, M. Sultan, G. Griffin and N. Muhammad, et al., Acidic ionic liquids: promising and cost-effective solvents for processing of lignocellulosic biomass, J. Mol. Liq., 2019, 110943 CrossRef CAS.
- Z. Ullah, A. S. Khan, N. Muhammad, R. Ullah, A. S. Alqahtani and S. N. Shah, et al., A review on ionic liquids as perspective catalysts in transesterification of different feedstock oil into biodiesel, J. Mol. Liq., 2018, 266, 673–686 CrossRef CAS.
- N. Muhammad, Z. Man, M. A. Mutalib, M. A. Bustam, C. D. Wilfred and A. S. Khan, et al., Dissolution and separation of wood biopolymers using ionic liquids, ChemBioEng Rev., 2015, 2, 257–278 CrossRef.
- S. N. Shah, K. C. Lethesh, M. A. Mutalib and R. B. M. Pilus, Evaluation of Thermophysical Properties of Imidazolium-Based Phenolate Ionic Liquids, Ind. Eng. Chem. Res., 2015, 54, 3697–3705 CrossRef CAS.
- B. Wang, L. Qin, T. Mu, Z. Xue and G. Gao, Are Ionic Liquids Chemically Stable?, Chem. Rev., 2017, 117, 7113–7131 CrossRef CAS PubMed.
- Y. Cao and T. Mu, Comprehensive investigation on the thermal stability of 66 ionic liquids by thermogravimetric analysis, Ind. Eng. Chem. Res., 2014, 53, 8651–8664 CrossRef CAS.
- H. Yu, Y.-T. Wu, Y.-Y. Jiang, Z. Zhou and Z.-B. Zhang, Low viscosity amino acid ionic liquids with asymmetric tetraalkylammonium cations for fast absorption of CO2, New J. Chem., 2009, 33, 2385–2390 RSC.
- Y. Shimizu, Y. Ohte, Y. Yamamura and K. Saito, Is the liquid or the solid phase responsible for the low melting points of ionic liquids? Alkyl-chain-length dependence of thermodynamic properties of [Cnmim][Tf2N], Chem. Phys. Lett., 2009, 470, 295–299 CrossRef CAS.
- W. Abdallah and U. Yilmazer, Novel thermally stable organo-montmorillonites from phosphonium and imidazolium surfactants, Thermochim. Acta, 2011, 525, 129–140 CrossRef CAS.
- W. H. Awad, J. W. Gilman, M. Nyden, R. H. Harris Jr, T. E. Sutto and J. Callahan, et al., Thermal degradation studies of alkyl-imidazolium salts and their application in nanocomposites, Thermochim. Acta, 2004, 409, 3–11 CrossRef CAS.
- J. M. Crosthwaite, M. J. Muldoon, J. K. Dixon, J. L. Anderson and J. F. Brennecke, Phase transition and decomposition temperatures, heat capacities and viscosities of pyridinium ionic liquids, J. Chem. Thermodyn., 2005, 37, 559–568 CrossRef CAS.
- Q. Zhang and J. n. M. Shreeve, Ionic liquid propellants: future fuels for space propulsion, Chem.–Eur. J., 2013, 19, 15446–15451 CrossRef CAS PubMed.
- Y. Chen, Y. Cao, Y. Shi, Z. Xue and T. Mu, Quantitative research on the vaporization and decomposition of [EMIM][Tf2N] by thermogravimetric analysis–mass spectrometry, Ind. Eng. Chem. Res., 2012, 51, 7418–7427 CrossRef CAS.
- G. Li, Z. Xue, B. Cao, C. Yan and T. Mu, Preparation and Properties of CX (X: O, N, S) Based Distillable Ionic Liquids and Their Application for Rare Earth Separation, ACS Sustainable Chem. Eng., 2016, 4, 6258–6262 CrossRef CAS.
- M. S. Shannon and J. E. Bara, Reactive and reversible ionic liquids for CO2 capture and acid gas removal, Sep. Sci. Technol., 2012, 47, 178–188 CrossRef CAS.
- K. Huang, Y.-L. Chen, X.-M. Zhang, S.-L. Ma, Y.-T. Wu and X.-B. Hu, Experimental study and thermodynamical modelling of the solubilities of SO2, H2S and CO2 in N-dodecylimidazole and 1,1′-[oxybis (2,1-ethanediyloxy-2,1-ethanediyl)]bis (imidazole): an evaluation of their potential application in the separation of acidic gases, Fluid Phase Equilib., 2014, 378, 21–33 CrossRef CAS.
- A. F. Ghobadi, V. Taghikhani and J. R. Elliott, Investigation on the solubility of SO2 and CO2 in imidazolium-based ionic liquids using NPT Monte Carlo simulation, J. Phys. Chem. B, 2011, 115, 13599–13607 CrossRef CAS PubMed.
- H. Mizuuchi, V. Jaitely, S. Murdan and A. Florence, Room temperature ionic liquids and their mixtures: potential pharmaceutical solvents, Eur. J. Pharm. Sci., 2008, 33, 326–331 CrossRef CAS PubMed.
- N. Meksi and A. Moussa, A review of progress in the ecological application of ionic liquids in textile processes, J. Cleaner Prod., 2017, 161, 105–126 CrossRef CAS.
- A. F. M. Cláudio, L. Swift, J. P. Hallett, T. Welton, J. A. Coutinho and M. G. Freire, Extended scale for the hydrogen-bond basicity of ionic liquids, Phys. Chem. Chem. Phys., 2014, 16, 6593–6601 RSC.
- J. A. Laszlo and D. L. Compton, Comparison of peroxidase activities of hemin, cytochrome c and microperoxidase-11 in molecular solvents and imidazolium-based ionic liquids, J. Mol. Catal. B: Enzym., 2002, 18, 109–120 CrossRef CAS.
- M. Gazitúa, R. A. Tapia, R. Contreras and P. R. Campodónico, Mechanistic pathways of aromatic nucleophilic substitution in conventional solvents and ionic liquids, New J. Chem., 2014, 38, 2611–2618 RSC.
- M. H. Ibrahim, M. Hayyan, M. A. Hashim and A. Hayyan, The role of ionic liquids in desulfurization of fuels: a review, Renewable Sustainable Energy Rev., 2017, 76, 1534–1549 CrossRef CAS.
- Y. Cao, Y. Chen, X. Sun, Z. Zhang and T. Mu, Water sorption in ionic liquids: kinetics, mechanisms and hydrophilicity, Phys. Chem. Chem. Phys., 2012, 14, 12252–12262 RSC.
- L. Shi and L. Zheng, Aggregation behavior of surface active imidazolium ionic liquids in ethylammonium nitrate: effect of alkyl chain length, cations, and counterions, J. Phys. Chem. B, 2012, 116, 2162–2172 CrossRef CAS PubMed.
- S. Bhattacharjee and S. Khan, The wetting behavior of aqueous imidazolium based ionic liquids: a molecular dynamics study, Phys. Chem. Chem. Phys., 2020, 22, 8595–8605 RSC.
- H. Xue, R. Verma and M. S. Jean’ne, Review of ionic liquids with fluorine-containing anions, J. Fluorine Chem., 2006, 127, 159–176 CrossRef CAS.
- Y.-G. Li, H.-S. Gao, W.-L. Li, J.-M. Xing and H.-Z. Liu, In situ magnetic separation and immobilization of dibenzothiophene-desulfurizing bacteria, Bioresour. Technol., 2009, 100, 5092–5096 CrossRef CAS PubMed.
- T. P. T. Pham, C.-W. Cho and Y.-S. Yun, Environmental fate and toxicity of ionic liquids: a review, Water Res., 2010, 44, 352–372 CrossRef CAS PubMed.
- M. C. Bubalo, K. Radošević, I. R. Redovniković, J. Halambek and V. G. Srček, A brief overview of the potential environmental hazards of ionic liquids, Ecotoxicol. Environ. Saf., 2014, 99, 1–12 CrossRef PubMed.
- A. Latała, M. Nędzi and P. Stepnowski, Toxicity of imidazolium and pyridinium based ionic liquids towards algae. Chlorella vulgaris, Oocystis submarina (green algae) and Cyclotella meneghiniana, Skeletonema marinoi (diatoms), Green Chem., 2009, 11, 580–588 RSC.
- S. P. Ventura, C. S. Marques, A. A. Rosatella, C. A. Afonso, F. Gonçalves and J. A. Coutinho, Toxicity assessment of various ionic liquid families towards Vibrio fischeri marine bacteria, Ecotoxicol. Environ. Saf., 2012, 76, 162–168 CrossRef CAS PubMed.
- M. Matzke, J. Arning, J. Ranke, B. Jastorff and S. Stolte, Design of inherently safer ionic liquids: toxicology and biodegradation, Handb. Green Chem., 2013, 6 Search PubMed.
- H. Wang, S. V. Malhotra and A. J. Francis, Toxicity of various anions associated with methoxyethyl methyl imidazolium-based ionic liquids on Clostridium sp, Chemosphere, 2011, 82, 1597–1603 CrossRef CAS PubMed.
- S. Viboud, N. Papaiconomou, A. Cortesi, G. Chatel, M. Draye and D. Fontvieille, Correlating the structure and composition of ionic liquids with their toxicity on Vibrio fischeri: a systematic study, J. Hazard. Mater., 2012, 215, 40–48 CrossRef PubMed.
- P. Fan, X. Qiu, F. U. Shah, Q. Ji and R. An, The effect of nanoscale friction of mesoporous carbon supported ionic liquids on the mass transfer of CO2 adsorption, Phys. Chem. Chem. Phys., 2020, 1097–1106 RSC.
- O. B. Ghanem, M. A. Mutalib, M. El-Harbawi, G. Gonfa, C. F. Kait and N. B. M. Alitheen, et al., Effect of imidazolium-based ionic liquids on bacterial growth inhibition investigated via experimental and QSAR modelling studies, J. Hazard. Mater., 2015, 297, 198–206 CrossRef.
- R. Biczak, B. Pawłowska, P. Bałczewski and P. Rychter, The role of the anion in the toxicity of imidazolium ionic liquids, J. Hazard. Mater., 2014, 274, 181–190 CrossRef CAS PubMed.
- S. Ravichandar, C. D. Wilfred, F. K. Chong and Z. G. Ng, Synthesis, characterization and application of 1-(2-cyanoethyl)-3-(3-methoxypropane) imidazolium bromide for CO2 capture, in MATEC Web of Conferences, 2017, p. 03003 Search PubMed.
- S. Aparicio, M. Atilhan and F. Karadas, Thermophysical properties of pure ionic liquids: review of present situation, Ind. Eng. Chem. Res., 2010, 49, 9580–9595 CrossRef CAS.
- T. Raj, R. Gaur, P. Dixit, R. P. Gupta, V. Kagdiyal and R. Kumar, et al., Ionic liquid pretreatment of biomass for sugars production: Driving factors with a plausible mechanism for higher enzymatic digestibility, Carbohydr. Polym., 2016, 149, 369–381 CrossRef CAS.
- J. Janikowski, M. R. Razali, S. R. Batten, D. R. MacFarlane and J. M. Pringle, Organic ionic plastic crystals and low viscosity ionic liquids based on the dicyano (nitroso) methanide anion, ChemPlusChem, 2012, 77, 1039–1045 CrossRef CAS.
- T. Abdallah, D. Lemordant and B. Claude-Montigny, Are room temperature ionic liquids able
to improve the safety of supercapacitors organic electrolytes without degrading the performances?, J. Power Sources, 2012, 201, 353–359 CrossRef CAS.
- R. Nanda, P. Rajamohanan and A. Kumar, Experimental Signature of Microheterogeneity in Ionic Liquid–H2O Systems and Their Perturbation by Adding Li+ Salts: A Pulsed Gradient Spin-Echo NMR Approach, ChemPhysChem, 2015, 16, 2936–2941 CrossRef CAS PubMed.
- R. Nanda, Thermal dynamics of lithium salt mixtures of ionic liquid in water by PGSE NMR spectroscopy, RSC Adv., 2016, 6, 36394–36406 RSC.
- R. Nanda, Unusual linear dependency of viscosity with temperature in ionic liquid/water mixtures, Phys. Chem. Chem. Phys., 2016, 18, 25801–25805 RSC.
- P. K. Sahu, R. Nanda, S. Seth, A. Ghosh and M. Sarkar, Nuclear magnetic resonance, fluorescence correlation spectroscopy and time-resolved fluorescence anisotropy studies of intermolecular interactions in bis(1-methyl-1H-imidazol-3-ium-3-yl)dihydroborate bis(trifluoromethylsulfonyl)amide and its mixtures with various cosolvents, Chem. Phys. Lett., 2016, 661, 100–106 CrossRef CAS.
- S. Zhu, Use of ionic liquids for the efficient utilization of lignocellulosic materials, J. Chem. Technol. Biotechnol., 2008, 83, 777–779 CrossRef CAS.
- B. Weyershausen and K. Lehmann, Industrial application of ionic liquids as performance additives, Green Chem., 2005, 7, 15–19 RSC.
- A. George, A. Brandt, K. Tran, S. M. N. S. Zahari, D. Klein-Marcuschamer and N. Sun, et al., Design of low-cost ionic liquids for lignocellulosic biomass pretreatment, Green Chem., 2015, 17, 1728–1734 RSC.
- H. G. Joglekar, I. Rahman and B. D. Kulkarni, The path ahead for ionic liquids, Chem. Eng. Technol., 2007, 30, 819–828 CrossRef CAS.
- S. K. Singh and A. W. Savoy, Ionic liquids synthesis and applications: An overview, J. Mol. Liq., 2020, 297, 112038 CrossRef CAS.
- A. Dharaskar Swapnil, Ionic liquids (a review): the green solvents for petroleum and hydrocarbon industries, Res. J. Chem. Sci., 2012, 2231, 606X Search PubMed.
- S. T. Vidal, M. J. Neiva Correia, M. M. Marques, M. R. Ismael and M. T. Angelino Reis, Studies on the use of ionic liquids as potential extractants of phenolic compounds and metal ions, Sep. Sci. Technol., 2005, 39, 2155–2169 CrossRef.
- Y. Fan, Y. Li, X. Dong, G. Hu, S. Hua and J. Miao, et al., Extraction of Phenols from Water with Functionalized Ionic Liquids, Ind. Eng. Chem. Res., 2014, 53, 20024–20031 CrossRef CAS.
- A. Brinda Lakshmi, A. Balasubramanian and S. Venkatesan, Extraction of phenol and chlorophenols using ionic liquid [Bmim]+[BF4]− dissolved in tributyl phosphate,", Clean: Soil, Air, Water, 2013, 41, 349–355 CAS.
- O. G. Sas, Á. Domínguez and B. González, Recovery and Elimination of Phenolic Pollutants from Water Using [NTf2] and [Nf2]-Based Ionic Liquids, J. Appl. Sci., 2019, 9, 4321 CrossRef CAS.
- O. G. Sas, I. Domínguez, Á. Domínguez and B. González, Using bis (trifluoromethylsulfonyl) imide based ionic liquids to extract phenolic compounds, J. Chem. Thermodyn., 2019, 131, 159–167 CrossRef CAS.
- O. G. Sas, P. B. Sánchez, B. González and Á. Domínguez, Removal of phenolic pollutants from wastewater streams using ionic liquids, Sep. Purif. Technol., 2020, 236, 116310 CrossRef CAS.
- E. J. González, I. Díaz, M. Gonzalez-Miquel, M. Rodríguez and A. Sueiras, On the behavior of imidazolium versus pyrrolidinium ionic liquids as extractants of phenolic compounds from water: Experimental and computational analysis, Sep. Purif. Technol., 2018, 201, 214–222 CrossRef.
- V. M. Egorov, S. V. Smirnova and I. V. Pletnev, Highly efficient extraction of phenols and aromatic amines into novel ionic liquids incorporating quaternary ammonium cation, Sep. Purif. Technol., 2008, 63, 710–715 CrossRef CAS.
- Y. Pei, J. Wang, K. Wu, Y. Zhao and J. Fan, Equilibrium partitioning of phenols and phenyl amines between [BF4]-based ionic liquids and aqueous solution, Z. Phys. Chem., 2007, 221, 825–835 CrossRef CAS.
- J. Fan, Y. Fan, Y. Pei, K. Wu, J. Wang and M. Fan, Solvent extraction of selected endocrine-disrupting phenols using ionic liquids, Sep. Purif. Technol., 2008, 61, 324–331 CrossRef CAS.
- A. S. Khan, T. H. Ibrahim, M. I. Khamis, P. Nancarrow and N. A. Jabbar, Role of cation and alkyl chain length on the extraction of phenol from aqueous solution using NTf2-based ionic liquids: Experimental and computational analysis, J. Mol. Liq., 2021, 326, 115305 CrossRef CAS.
- A. S. Khan, T. H. Ibrahim, Z. Rashid, M. I. Khamis, P. Nancarrow and N. A. Jabbar, COSMO-RS based screening of ionic liquids for extraction of phenolic compounds from aqueous media, J. Mol. Liq., 2021, 328, 115387 CrossRef CAS.
- C. Yao, Y. Hou, S. Ren, Y. Ji, W. Wu and H. Liu, Efficient separation of phenolic compounds from model oils by dual-functionalized ionic liquids, Chem. Eng. Process., 2018, 128, 216–222 CrossRef CAS.
- R. Sulaiman, I. Adeyemi, S. Abraham, S. Hasan and I. AlNashef, Liquid-liquid extraction of chlorophenols from wastewater using hydrophobic ionic liquids, J. Mol. Liq., 2019, 294, 111680 CrossRef CAS.
- N. Deng, M. Li, L. Zhao, C. Lu, S. L. de Rooy and I. M. Warner, Highly efficient extraction of phenolic compounds by use of magnetic room temperature ionic liquids for environmental remediation, J. Hazard. Mater., 2011, 192, 1350–1357 CrossRef CAS PubMed.
- J. Fan, Y. Fan, Y. Pei, K. Wu, J. Wang and M. J. S. Fan, Solvent extraction of selected endocrine-disrupting phenols using ionic liquids, Sep. Purif. Technol., 2008, 61, 324–331 CrossRef CAS.
- S. T. Vidal, M. J. Neiva Correia, M. M. Marques, M. R. Ismael and M. T. Angelino Reis, Studies on the use of ionic liquids as potential extractants of phenolic compounds and metal ions, Sep. Sci. Technol., 2005, 39, 2155–2169 CrossRef.
- Y. Hou, Y. Ren, W. Peng, S. Ren and W. Wu, Separation of phenols from oil using imidazolium-based ionic liquids, Ind. Eng. Chem. Res., 2013, 52, 18071–18075 CrossRef CAS.
- C. Yao, Y. Hou, S. Ren, Y. Ji, W. Wu and H. Liu, Efficient separation of phenolic compounds from model oils by dual-functionalized ionic liquids, Chem. Eng. Process., 2018, 128, 216–222 CrossRef CAS.
- P. Isosaari, V. Srivastava and M. Sillanpää, Ionic liquid-based water treatment technologies for organic pollutants: Current status and future prospects of ionic liquid mediated technologies, Sci. Total Environ., 2019, 690, 604–619 CrossRef CAS PubMed.
- X. Chen, F. Li, C. Asumana and G. Yu, Extraction of soluble dyes from aqueous solutions with quaternary ammonium-based ionic liquids, Sep. Purif. Technol., 2013, 106, 105–109 CrossRef CAS.
- Z. Li, R. Li, X. Yuan, Y. Pei, Y. Zhao and H. Wang, et al., Anionic structural effect in liquid–liquid separation of phenol from model oil by choline carboxylate ionic liquids, Green Energy Environ., 2019, 4, 131–138 CrossRef.
- M. Jiang, S. Shen and Y. J. J. o. M. L. Chen, Equilibria investigation and extraction thermodynamic of extracting (o-/m-/p-)-cresol from aqueous solution using 1-dodecanol, J. Mol. Liq., 2019, 283, 96–107 CrossRef CAS.
- X. Xu, A. Li, T. Zhang, L. Zhang, D. Xu and J. Gao, et al., Efficient extraction of phenol from low-temperature coal tar model oil via imidazolium-based ionic liquid and mechanism analysis, J. Mol. Liq., 2020, 306, 112911 CrossRef CAS.
- H. Gai, L. Qiao, C. Zhong, X. Zhang, M. Xiao and H. Song, Designing Ionic Liquids with Dual Lewis Basic Sites to Efficiently Separate Phenolic Compounds from Low-Temperature Coal Tar, ACS Sustainable
Chem. Eng., 2018, 6, 10841–10850 CrossRef CAS.
- N. Sidek, N. S. A. Manan and S. Mohamad, Efficient removal of phenolic compounds from model oil using benzyl Imidazolium-based ionic liquids, J. Mol. Liq., 2017, 240, 794–802 CrossRef CAS.
- Y. Ji, Y. Hou, S. Ren, C. Yao and W. Wu, Tetraethylammonium amino acid ionic liquids and CO2 for separation of phenols from oil mixtures, Energy Fuels, 2018, 32, 11046–11054 CrossRef CAS.
- X. L. Zhuang, Z. Q. Li and E. Q. Wang, Study on the extraction of phenolic compounds from model oil by using ionic liquid, in Advanced Materials Research, 2014, pp. 137–141 Search PubMed.
- X. Yan, S. Anguille, M. Bendahan and P. Moulin, Ionic liquids combined with membrane separation processes: A review, Sep. Purif. Technol., 2019, 222, 230–253 CrossRef CAS.
- L. Zhang, Y. Ding, B. Long, L. Yao, H. Yuan and Y. Dai, Hierarchical porous polymeric ionic liquids with excellent adsorption performance for phenolic compounds, J. Mol. Liq., 2020, 312, 113440 CrossRef CAS.
- M. Cheng, J. Jiang, J. Wang and J. Fan, Highly Salt Resistant Polymer Supported Ionic Liquid Adsorbent for Ultrahigh Capacity Removal of p-Nitrophenol from Water, ACS Sustainable Chem. Eng., 2019, 7, 8195–8205 CrossRef CAS.
- G. Zhu, G. Cheng, T. Lu, Z. Cao, L. Wang and Q. Li, et al., An ionic liquid functionalized polymer for simultaneous removal of four phenolic pollutants in real environmental samples, J. Hazard. Mater., 2019, 373, 347–358 CrossRef CAS PubMed.
- L. Gholami-Bonabi, N. Ziaefar and H. Sheikhloie, Removal of phenol from aqueous solutions by magnetic oxide graphene nanoparticles modified with ionic liquids using the Taguchi optimization approach, Water Sci. Technol., 2020, 81, 228–240 CrossRef CAS PubMed.
- L. Lupa, L. Cocheci, R. Pode and I. Hulka, Phenol adsorption using Aliquat 336 functionalized Zn-Al layered double hydroxide, Sep. Purif. Technol., 2018, 196, 82–95 CrossRef CAS.
- X. Hou, X. Lu, S. Tang, L. Wang and Y. Guo, Graphene oxide reinforced ionic liquid-functionalized adsorbent for solid-phase extraction of phenolic acids, J. Chromatogr. B: Anal. Technol. Biomed. Life Sci., 2018, 1072, 123–129 CrossRef CAS PubMed.
- H. M. Marwani and E. M. Bakhsh, Selective adsorption of 4-chlorophenol based on silica-ionic liquid composite developed by sol–gel process, Chem. Eng. J., 2017, 326, 794–802 CrossRef CAS.
- L. Zhu, Y. Deng, J. Zhang and J. Chen, Adsorption of phenol from water by N-butylimidazolium functionalized strongly basic anion exchange resin, J. Colloid Interface Sci., 2011, 364, 462–468 CrossRef CAS PubMed.
- A. Balasubramanian and S. Venkatesan, Removal of phenolic compounds from aqueous solutions by emulsion liquid membrane containing Ionic Liquid [BMIM]+[PF6]− in Tributyl phosphate, Desalination, 2012, 289, 27–34 CrossRef CAS.
- F. Garavand and A. Madadlou, Recovery of phenolic compounds from effluents by a microemulsion liquid membrane (MLM) extractor, Colloids Surf., A, 2014, 443, 303–310 CrossRef CAS.
- J. Gorke, F. Srienc and R. Kazlauskas, Toward advanced ionic liquids. Polar, enzyme-friendly solvents for biocatalysis, Biotechnol. Bioprocess Eng., 2010, 15, 40–53 CrossRef CAS.
- S. P. Ventura, A. M. Gonçalves, T. Sintra, J. L. Pereira, F. Gonçalves and J. A. Coutinho, Designing ionic liquids: the chemical structure role in the toxicity, Ecotoxicology, 2013, 22, 1–12 CrossRef CAS PubMed.
- K. S. Egorova and V. P. Ananikov, Toxicity of ionic liquids: eco (cyto) activity as complicated, but unavoidable parameter for task-specific optimization, ChemSusChem, 2014, 7, 336–360 CrossRef CAS PubMed.
- A. K. Dwamena, Recent advances in hydrophobic deep eutectic solvents for extraction, Separations, 2019, 6(9), 3–15 Search PubMed.
- C. Florindo, L. Branco and I. Marrucho, Development of hydrophobic deep eutectic solvents for extraction of pesticides from aqueous environments, Fluid Phase Equilib., 2017, 448, 135–142 CrossRef CAS.
- C. Florindo, L. Romero, I. Rintoul, L. C. Branco and I. M. Marrucho, From phase change materials to green solvents: hydrophobic low viscous fatty acid–based deep eutectic solvents, ACS Sustainable Chem. Eng., 2018, 6, 3888–3895 CrossRef CAS.
- O. G. Sas, M. Castro, Á. Domínguez and B. González, Removing phenolic pollutants using deep eutectic solvents, Sep. Purif. Technol., 2019, 227, 115703 CrossRef CAS.
- I. Adeyemi, R. Sulaiman, M. Almazroui, A. Al-Hammadi and I. AlNashef, Removal of chlorophenols from aqueous media with hydrophobic deep eutectic solvents: Experimental study and COSMO RS evaluation, J. Mol. Liq., 2020, 113180 CrossRef CAS.
- D. Yang, Y. Wang, J. Peng, C. Xun and Y. Yang, A green deep eutectic solvents microextraction coupled with acid-base induction for extraction of trace phenolic compounds in large volume water samples, Ecotoxicol. Environ. Saf., 2019, 178, 130–136 CrossRef CAS PubMed.
- I. A. Lawal, M. M. Lawal, M. A. Azeez and P. Ndungu, Theoretical and experimental adsorption studies of phenol and crystal violet dye on carbon nanotube functionalized with deep eutectic solvent, J. Mol. Liq., 2019, 288, 110895 CrossRef CAS.
- T. Gu, M. Zhang, T. Tan, J. Chen, Z. Li and Q. Zhang, et al., Deep eutectic solvents as novel extraction media for phenolic compounds from model oil, Chem. Commun., 2014, 50, 11749–11752 RSC.
- W. Guo, Y. Hou, W. Wu, S. Ren, S. Tian and K. N. Marsh, Separation of phenol from model oils with quaternary ammonium salts via forming deep eutectic solvents, Green Chem., 2013, 15, 226–229 RSC.
- K. Pang, Y. Hou, W. Wu, W. Guo, W. Peng and K. N. Marsh, Efficient separation of phenols from oils via forming deep eutectic solvents, Green Chem., 2012, 14, 2398–2401 RSC.
- L. Yi, J. Feng, W. Li and Z. Luo, High-performance separation of phenolic compounds from coal-based liquid oil by deep eutectic solvents, ACS Sustainable Chem. Eng., 2019, 7, 7777–7783 CrossRef CAS.
- C. Yao, Y. Hou, S. Ren, W. Wu, K. Zhang and Y. Ji, et al., Efficient separation of phenol from model oils using environmentally benign quaternary ammonium-based zwitterions via forming deep eutectic solvents, Chem. Eng. J., 2017, 326, 620–626 CrossRef CAS.
- E. L. Byrne and J. D. Holbrey, Phenol Recovery from Aromatic Solvents by Formation of Eutectic Liquids with Trialkyl-2,3-dihydroxypropylammonium Chloride Salts, Sustainable Chem., 2020, 1, 49–61 CrossRef.
- L. Yi, J. Feng, A. Rajendran and W.-Y. Li, Minimizing aromatics entrainment in dephenolization of coal-based liquids by deep eutectic solvents, Chem. Eng. Sci.: X, 2020, 8, 100070 CAS.
- Y. Ji, Y. Hou, S. Ren, C. Yao and W. Wu, Highly efficient separation of phenolic compounds from oil mixtures by imidazolium-based dicationic ionic liquids via forming deep eutectic solvents, Energy Fuels, 2017, 31, 10274–10282 CrossRef CAS.
|
This journal is © The Royal Society of Chemistry 2021 |