DOI:
10.1039/D0RA09785C
(Paper)
RSC Adv., 2021,
11, 9336-9348
Fabrication of TiO2 microspikes for highly efficient intracellular delivery by pulse laser-assisted photoporation
Received
18th November 2020
, Accepted 15th February 2021
First published on 2nd March 2021
Abstract
The introduction of foreign cargo into living cells with high delivery efficiency and cell viability is a challenge in cell biology and biomedical research. Here, we demonstrate a nanosecond pulse laser-activated photoporation for highly efficient intracellular delivery using titanium dioxide (TiO2) microspikes as a substratum. The TiO2 microspikes were formed on titanium (Ti) substrate using an electrochemical anodization process. Cells were cultured on top of the TiO2 microspikes as a monolayer, and the biomolecule was added. Due to pulse laser exposure of the TiO2 microspike–cell membrane interface, the microspikes heat up and induce cavitation bubbles, which rapidly grow, coalesce and collapse to induce explosion, resulting in very strong fluid flow at the cell membrane surface. Thus, the cell plasma membrane disrupts and creates transient nanopores, allowing delivery of biomolecules into cells by a simple diffusion process. By this technique, we successfully delivered propidium iodide (PI) dye in HeLa cells with high delivery efficiency (93%) and high cell viability (98%) using 7 mJ pulse energy at 650 nm wavelength. Thus, our TiO2 microspike-based platform is compact, easy to use, and potentially applicable for therapeutic and diagnostic purposes.
1. Introduction
Titanium (Ti) and Ti-based alloys are commonly used for dental prosthetics and orthopedic applications owing to their higher mechanical strength, biocompatibility, corrosion resistance, and micro/nanostructured features.1–3 In recent years, micro/nanotechnology has emerged as a vital research area with various applications. Micro/nanostructured metal oxide synthesis has gained significant research interest due to its favorable application for micro and nanodevice fabrication.4 The micro/nanostructures on titanium metal are advantageous, as they are less costly, possess a tailorable surface chemistry, well-ordered micro/nanostructures, flexibility, high surface area, mechanical rigidity, and outstanding biocompatibility.5–8 Nanostructured materials such as nanorods, nanowires, nanoparticles, nanofilms, nanotubes, etc., have been constructed due to their promising applications in numerous fields. The electrochemical formation of porous/micro/nanostructures for different types of materials such as Al,9 Fe,10 Hf,11 Mg,12 Nb,13 Ni,14 Ru,15 Si,16 W,17 and Ti18–20 already exists. Besides the metal substrate, nanostructures can also be formed using anodization on Ti alloy substrates, such as Ti–6Al–7Nb,8,21 Ni–Ti,22,23 Ti–6Al–4V,7 Ti13Nb13Zr24,25 and Ti15Mo.26 Among the many nanostructured oxide materials, TiO2-based nanostructures have significant characteristics, such as improved ion-exchangeable ability, photocatalytic properties, environmental safety, and nontoxicity. However, the formation of TiO2 microspikes on titanium substrate by electrochemical anodization process and their biomedical applications, such as drug delivery or intracellular delivery, have not yet been studied.
Intracellular delivery is a critical task in nanomedicine-based drug delivery. The past few decades have witnessed the progress of nanomedicine from biologically inert materials to smart systems, intended at evolving in vivo functionality. Probing the nano-bio interactions and the necessary functionalities at the molecular, cellular, and tissue levels are often ignored. Development towards a response to these queries will yield more effective chemo-physical approaches.32 The intracellular delivery of foreign macromolecules into cells is essential to basic biomedical research and the analysis of many diseases.27–31 The capability to transport foreign macromolecules into living cells with high transfection efficacy and high cell viability is of great interest in cell biology for the applications in therapeutics, diagnostics, and drug delivery.27–31 In the last two decades, different drug delivery methods have been developed to control drug dosage, target delivery and reduce the side effects. These methods can be classified as viral, chemical and physical.31,33–37 Viral vectors are predominantly used for gene therapy; however, this technique is cell-specific and generates an immune response with high toxicity.38 Chemical methods, such as basic protein and calcium phosphate, are frequently restricted by the low efficacy of plasmid delivery in various cell types due to their toxicity and plasmid degradation.39 Different physical methods, such as jet injection, microinjection, mechanoporation, electroporation, photoporation, etc., are being widely used for highly efficient cargo delivery with low toxicity.40–49
Physical approaches are widely being developed, which use physical energy to deform the cell plasma membrane and deliver cargo into cells.27,31,35,50 For example, in electroporation, an electric field is required to deform cell membranes and create transient membrane pores for cargo delivery. However, it has limitations, such as electric field distortion, pH variation, sample contamination, and high toxicity effect, reducing cell viability.41,42 The microinjection technique is time-consuming, and highly skilled technicians are required, which means delivery throughput is low, and therefore, microinjection not widely used.27,28 The sonoporation method creates temporary membrane pores using shockwaves to carry biomolecules into cells. However, it produces high toxicity, and the substantial shear force easily causes cell damage and produces low cell viability.51 On the other hand, photoporation technique involves light–matter interactions to disrupt the plasma membrane and deliver biomolecules into cells.47,49 It is a contactless delivery technique, less invasiveness, less toxic for cells, and has been widely used in the past few years. However, its transfection efficacy in terms of different cell types and cell viability needs to be enhanced.
To address the various hurdles for cargo delivery into cells, we proposed a TiO2 microspike-based photoporation platform to achieve highly controllable intracellular delivery with high delivery efficiency and cell viability. In this work, we fabricated microspikes on titanium substrate by electrochemical anodization process, and cells were cultured on them. Pulsed laser on the microspikes induces heat, resulting in cavitation bubble formation and jet flow on the cell membrane. Thus, the cell membrane creates transient membrane pores that allow delivery of molecules into cells with high efficiency and cell viability. Our TiO2-based photoporation platform is flexible and highly effective for intracellular delivery, and it is appropriate for cellular therapy and diagnostics.
2. Materials and methods
2.1. Sample preparation
The titanium substrate was purchased from Sigma Aldrich, USA, with 0.25 mm sheet thickness. The sheet was cut to 20 × 20 mm in length and width. Afterward, the small sheets were etched with Kroll's reagent to eliminate the native oxides. Finally, they were ultrasonically cleaned using acetone and isopropyl alcohol (IPA), then dried under nitrogen.
2.2. Electrochemical anodization
TiO2 microspikes were fabricated on a Ti (titanium) substrate using the electrochemical anodization technique. To fabricate microspikes, initially, Ti substrate was introduced into the beaker containing electrolyte, then a counter electrode was inserted with a fixed separation distance of 2 cm. A platinum electrode acted as a cathode, and Ti was used as anode. For Ti anodization, different voltages, time, and electrolyte compositions were used to fabricate the microspikes. The electrolyte was prepared by mixing 50 ml of 0.08 M hydrofluoric acid (HF) in 50 ml of 1 M sulfuric acid (H2SO4). The 100 ml electrolyte solution was continuously maintained, and the magnetic stirrer was rotated continuously at 300 rpm. The anodization voltage was varied from 0 to 40 V for 1 h with a DC power supply using ramp mode (2 V min−1). The anodic current density was verified throughout the experimental process.25 After fabrication, the sample was cleaned using a standard cleaning procedure with acetone, IPA (isopropyl alcohol), and deionized water, then dried by nitrogen gas. Finally, the cleaned sample was heated on a hot plate at 120 °C for five minutes.
2.3. Cell culture
For our photoporation experiment, human cervical cancer cells (HeLa) were used, purchased from the National Centre for Cell Science (NCCC), Pune, India. To culture HeLa cells, initially, the old medium was withdrawn from the cell culture dish using a pipette. Phosphate-Buffered Saline (PBS) was added into the cell culture dish, which was shaken in different orientations to properly clean the cell surface. The PBS cleaning procedure was carried out three times, and finally, it was completely removed from the dish surface. Afterward, 1 ml trypsin (0.05% trypsin–EDTA, GIBCO) was added to the cell culture dish. The culture was incubated for 5–7 minutes in an incubator at 37 °C in a 5% CO2 atmosphere. As a result, cells were detached from the cell culture dish surface. Finally, 9 ml of DMEM (Dulbecco's Modified Eagle Medium, Thermo Fisher Scientific, USA, with 10% fetal bovine serum [FBS], Gibco, USA, and 1% penicillin–streptomycin) was added into the dish and mixed adequately with cells. The cells with DMEM medium were transferred on UV-treated microspike chip surface with a cell concentration of 1.2 × 106 cells per ml and incubated overnight in an incubator for the photoporation experiment.
2.4. Photoporation experimental procedure
After overnight cell culture, the HeLa cells have firmly adhered on the microspike chip surface. Then, we cleaned the cell surface using PBS, and the process was continuously repeated three times. Afterward, a fresh PBS solution with diluted PI dye was added into the chip surface with a PI dye concentration of 0.05 μl ml−1. Then, the pulse laser was exposed and scanned on top of the chip surface. Photoporation experiments were carried out using Ekspla NT 342B-10-AW10 tunable nanosecond pulsed laser at 650 nm wavelength, with 5 ns pulse width and 10 Hz pulse frequency. For successful photoporation experiments, different pulse laser energies, PI dye concentrations, and laser scanning speeds were used to achieve highly efficient intracellular delivery with high cell viability.
2.5. Fluorescence microscopy
We used a non-inverted industrial fluorescence microscope (Nikon LV150N, Tokyo, Japan) with brightfield, darkfield, simple polarizing, double beam interferometry, and DIC attachments. To visualize fluorescence images, a 20× objective lens (NA/WD = 0.45/4.5 mm) was used, and a DS-Ri2 microscope CMOS camera (Nikon, 6fps [4908 × 3246 pixels] to 45 fps [1636 × 1088 pixels]) was used to capture the fluorescence images. Fluorescence excitation was provided by a 100 W mercury lamp. The excitation and emission of propidium iodide (PI) dye and calcein-AM were EX 535–550 nm/DM 575 nm/DM 580 nm and EX 470–540 nm/DM 505 nm/EM 510 nm.
2.6. Cell viability studies
Initially, PI dye delivery (red fluorescence) and calcein-AM stained live cells (green fluorescence) were recorded by a fluorescence microscope. Then, the images were analyzed using the Image J software.52 The MTT (3-[4,5-dimethylthiazol-2-yl]-2,5-diphenyltetrazolium bromide, analytical grade, TCI, Japan) assay was accomplished using HeLa cells to measure the cell viability of all the tested samples, following the well-defined process in previous studies.12,53 Each sample was added to a 30 mm cell culture plate, and HeLa cells were seeded with a density of 0.5 × 105 cells per mL. The MTT assay was performed after 2 h, 3 days, and 7 days before and after laser exposure of the samples. For the assay, 50 μl of 5 mg ml−1 MTT (stock) was added in each plate and then incubated at a temperature of 37 °C in a 5% CO2 incubator for four hours, resulting in the formation of formazan crystals. Afterward, the cell culture medium containing MTT was removed from the culture plate by aspiration. Then, formazan crystals were dissolved by adding 500 μl dimethyl sulfoxide (DMSO, Sigma-Aldrich). Finally, 200 μl of dissolved formazan crystals with DMSO solution was transferred into a 96-well plate for the reading of optical density at 570 nm through a plate reader (AS ONE MPR-A 100).54
2.7. Characterization techniques
Attenuated total reflectance Fourier transform infrared spectra (ATR-FTIR) were recorded in the range of 400 to 4000 cm−1 using a Jasco FT/IR-6300 spectrometer to determine oxide formation on the microspike chip surface. Raman analyses were carried out using Jasco NRS-1000 laser Raman spectrophotometer with a 532 nm laser. The sample surface morphology was examined by a Quanta 400 field emission scanning electron microscope (FESEM) equipped with an Inca Penta Fetx3 (Oxford) energy-dispersive X-ray spectroscopy (EDS) analyzer for elemental analysis. The 3D profiles of FESEM images were obtained using the scanning probe image processor WSxM 7.0 software.5 Water contact angles were measured using Surface Electro-Optics Phoenix contact angle meter through the sessile drop process. Water droplets of around ∼8 μL were cautiously dropped onto the titanium and microspike surface using a syringe. The contact angle was measured at different places on each sample, then the average value was reported. The composition of the samples was analyzed by X-ray photoelectron spectroscopy (XPS) using Quantera SXM-CI, scanning X-ray Microprobe, ULVAC-PHI, INC XPS system with 25 W, 15 kV non-monochromatic Al Kα radiation with 1486.6 eV energy. Core level spectra of Ti 2p, O 1s, and C 1s were obtained at the pass energy of 12.6 eV. The Ti 2p and O 1s core-level spectra were fitted with Gaussian–Lorentzian peaks to identify various species after subtracting a Shirley-type background using the CASAXPS software.6 COMSOL multiphysics simulation software was used to calculate the spatial distribution of temperature.
3. Results and discussion
3.1. Morphology of microspikes
The surface morphology of the microspike device is shown in Fig. 1a. Fig. 1b shows the high-resolution FESEM image of a single microspike. 3D profiles of the FESEM images were acquired using the scanning probe image processor WSxM 7.0 software. Fig. 1c and d show the 3D images of the microspikes acquired from FESEM images. From these images, the high degree of arrangement of the microspike structure is confirmed. The height of microspikes is ∼1.5 to 2 μm, with diameters of ∼4 to 5 μm, and the average interspike spacing is ∼8 to 10 μm. The wettability of the titanium substrate and microspikes was estimated using the water contact angle measurement. The water contact angle is ∼66° on a bare titanium substrate, whereas water completely spreads on the microspike samples, confirming the super hydrophilicity.
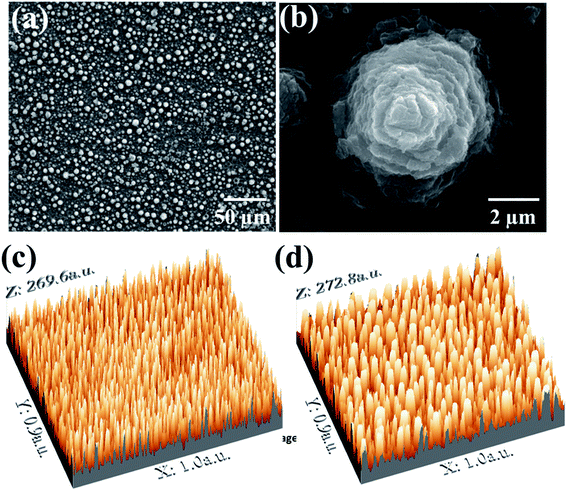 |
| Fig. 1 The TiO2 micro-spikes fabrication on Ti substrate using the electrochemical anodization process (a) the FESEM image of micro-spikes (b) single microspike at higher magnification (c and d) the 3D construction of micro-spikes from FESEM image at different magnifications. | |
3.2. TiO2 microspike characterizations
3.2.1. ATR-FTIR analysis. The ATR-FTIR measurements were carried out in the range of 400–4000 cm−1 to define the oxide formation (Fig. 2a) on microspikes. The bands in the range of 500–800 cm−1 could be due to the formation of Ti–O and Ti–O–Ti bonds, which originate from the oxide layer of the TixOy.55,56 The peak from the 800 cm−1 to 900 cm−1 range is assigned to the formation of a O–O bond. The bands positioned at around 1620 to 1630 cm−1 and 3000 to 3350 cm−1 indicate the features of surface adsorbed hydroxyl groups and H2O.57,58
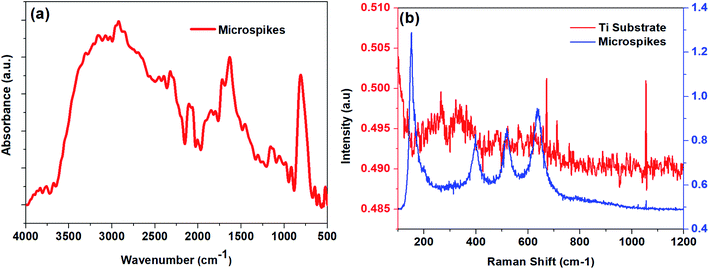 |
| Fig. 2 (a) ATR-FTIR spectrum of micro-spikes (b) Raman spectra of micro-spikes and Ti substrate. | |
3.2.2. Raman spectroscopy analysis. The Raman spectroscopy results (Fig. 2b) confirmed that the typical structures of titanium oxides are anatase and rutile in the tetragonal space group. The anatase structure has six Raman active modes, including 3Eg, 2B1g, 1A1g, with Raman frequencies at 639 (Eg), 519 (B1g), 513 (A1g), 399 (B1g), 197 (Eg), and 144 (Eg) cm−1.59–61 The rutile has four Raman active modes, B1g, B2g, Eg, and A1g. The bulk Raman frequencies of the rutile phase are at 143 (B1g), 447 (Eg), 612 (A1g), and 826 (B1g) cm−1.59 The existence of anatase and rutile peak shift from their positions, along with scattered Raman peaks, may be due to the abundant band structure.61,62
3.2.3. EDS and XPS analysis. The composition obtained using EDS on titanium substrate is 77.5 wt% Ti and 22.5 wt% oxygen. Microspikes have the elemental composition of 56.57 wt% oxygen and 43.43 wt% Ti. Furthermore, detailed XPS characterization has been carried out to understand the surface nature of the titanium substrate and microspikes. The Ti 2p and O 1s core-level spectra of Ti substrate and microspikes before and after etching were recorded and are presented in Fig. 3. The Ti 2p and O 1s core-level spectra from the substrate and microspike samples were fitted with Gaussian–Lorentzian peaks and are shown in Fig. 4 and Fig. 5, respectively. The bare Ti substrate shows several peaks indicating the presence of different Ti species, and they are resolved by curve fitting. The Ti 2p3/2 peaks at 454.1 and 458.4, along with spin–orbit peaks in the curve-fitted core-level spectrum, confirmed the presence of Ti metal and Ti4+ species in the substrate.63,64 However, the relative concentration of TiO2 species obtained from the area under the peak is much more than that of Ti metal in the substrate, indicating surface oxidation of the Ti substrate (Fig. 4a). The mild sputter etching of the substrate shows only Ti metal peaks (2p3/2 at 454.2 eV), as presented in Fig. 4b. The Ti 2p3/2,1/2 peaks at 458.7 and 464.4 eV in microspikes indicate the presence of TiO2 species, which is clear from its Ti 2p core-level spectrum (Fig. 4c). The Ti 2p core-level spectrum of microspikes after sputter etching is broad in nature, containing several Ti species, and is decomposed into component peaks by curve-fitting, as displayed in Fig. 4d. The decomposed Ti 2p3/2 peaks observed at 454.2, 456.3, and 458.1 eV are assigned to Ti metal, Ti sub-oxide (TixOy/defects), and TiO2 species, respectively.63,65–68 The relative concentration of the TiO2 is the dominating one among all the Ti species. It is important to note that an intermediate sub-oxide species was observed around 456.3 eV, which could be due to defect formation.66–69 The surface concentrations, binding energies of the Ti substrate, and microspike component species evaluated from XPS studies are shown in Tables 1 and 2. The O 1s core levels of Ti substrate and of the microspikes before and after etching conditions were also recorded and are shown in Fig. 5. The broad nature of their spectra suggests the existence of different oxygen species present on their surface, and the spectra can be curve-fitted into several components of oxygen species.65 An intense peak around 530 eV is ascribed to oxide species present in the substrate and microspikes at different conditions. The observed peaks around 531 eV and >532.5 eV correspond to oxygen adsorbed on the surface or are related to the hydroxyl (OH−) species and surface-adsorbed H2O.6,65 In the case of microspike O 1s after sputter etching, the peak around 531.5 eV could correspond to TixOy (may overlap with OH−), which is due to the oxygen vacancies (defective oxides).67 The O 1s component (defects and OH−) peaks' area under the curve (AUC) was measured and is presented in Fig. 6a. The increase in the AUC of TixOy after etching microspike samples confirms the oxygen defects (TixOy) on microspikes. Similarly, the AUC of the microspike Ti 2p (from Fig. 4d) is presented in Fig. 6b. This substantiates the presence of Ti and TixOy, along with TiO2. The corresponding defect oxide peaks are also observed in the microspike Ti 2p spectrum after etching (Fig. 3d, 4d, and 5d).
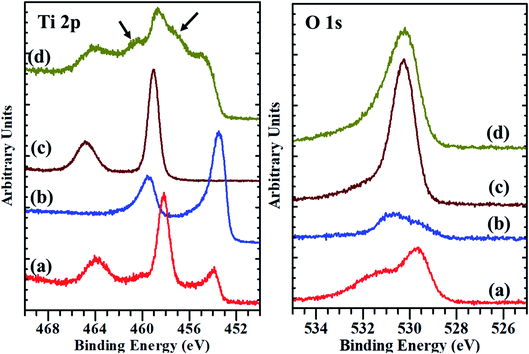 |
| Fig. 3 The XPS core-level spectra of Ti 2p and O 1s: (a) substrate before etching, (b) substrate after etching, (c) micro-spikes before etching, (d) micro-spikes after etching. | |
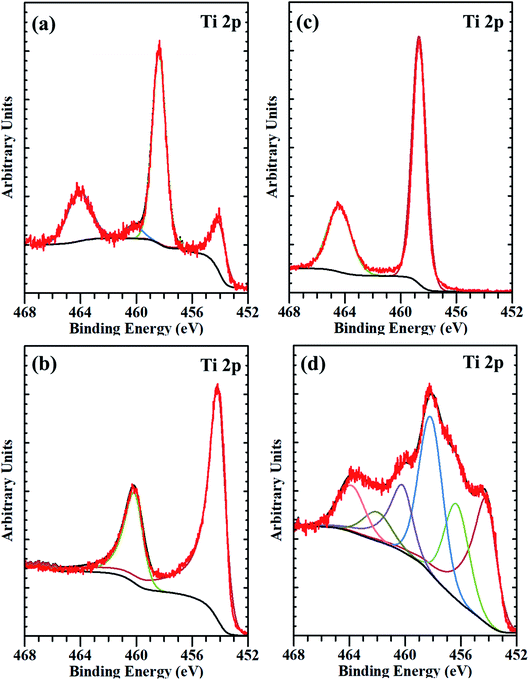 |
| Fig. 4 The deconvoluted XPS spectra of Ti 2p core-level from (a) substrate before etching, (b) substrate after etching, (c) micro-spikes before etching, and (d) micro-spikes after etching. | |
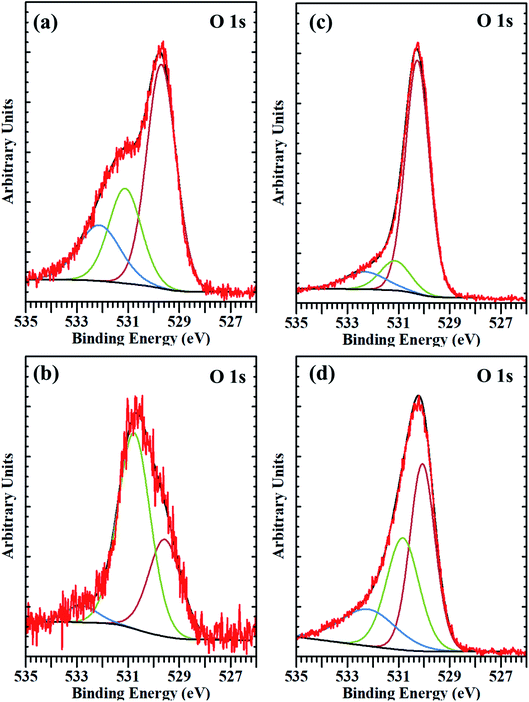 |
| Fig. 5 The deconvoluted XPS spectra of O 1s core-level from (a) substrate before etching, (b) substrate after etching, (c) micro-spikes before etching, and (d) microspikes after etching. | |
Table 1 Composition of substrate and microspike samples obtained from XPS
Sample/species |
Titanium substrate before etching (at%) |
Titanium substrate after etching (at%) |
Microspikes before etching (at%) |
Microspikes after etching (at%) |
Ti 2p |
20.7 |
65.6 |
31.5 |
32.1 |
O 1s |
79.3 |
34.4 |
68.5 |
67.9 |
Table 2 Binding energy of Ti 2p and O 1s core levels in different compounds with relative peak area percentage
Sample |
Ti species |
Ti 2p3/2 binding energy (eV) |
Relative peak area (%) |
O species |
O binding energy (eV) |
Relative peak area (%) |
Ti substrate before etching |
Ti0 |
454.1 |
20.5 |
Oxide |
530.0 |
54.6 |
Ti4+ |
458.4 |
79.5 |
OH− |
531.1 |
25.7 |
Adsorbed H2O |
532.1 |
19.7 |
Ti substrate after etching |
Ti0 |
454.2 |
100 |
Oxide |
530.0 |
31.4 |
OH− |
530.8 |
63.3 |
Adsorbed H2O |
532.8 |
5.3 |
Microspikes before etching |
Ti4+ |
458.7 |
100 |
Oxide |
530.3 |
77.3 |
OH− |
531.1 |
12.4 |
Adsorbed H2O |
532.3 |
10.3 |
Microspikes after etching |
Ti0 |
454.2 |
30 |
Oxide |
530.1 |
44.4 |
TixOy (Ti3+/Ti2+) |
456.3 |
27 |
OH−/defects |
531.5 |
35.1 |
Ti4+ |
458.2 |
43 |
Adsorbed H2O |
532.2 |
19.5 |
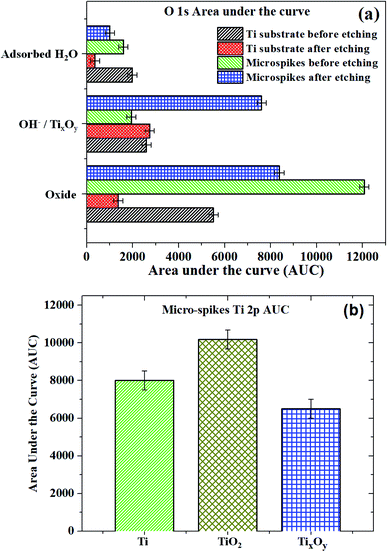 |
| Fig. 6 Area under the curve (AUC) obtained from components peaks of (a) O 1s and (b) Ti 2p of micro-spikes after etching. | |
3.3. Theoretical study
To unveil the causes of intracellular delivery in microspikes structures, we performed Comsol Multiphysics simulations of electromagnetic radiation in the mixed titanium oxide structures. To reduce the mesh size, we simplified the complex structure of microspikes consisting of a 2 μm wide TiO2 (anatase phase is considered here) rectangular microsubstrate, which emerged in a water environment. The surface of this substrate is decorated with semi-circular Ti3O5 structures. Here, we have considered Ti3O5 as the dominant suboxide of Ti species, as seen in the peak at ∼456.3 eV in XPS spectra (Fig. 3d).70 Since the shape and size distributions of these Ti3O5 structures are unknown, we have taken the arbitrary diameter of around 400 nm in the model. To simulated resistive heating due to pulsed laser exposure with the fluence of 35 mJ cm−2 (5 ns pulse duration), we used the emw module of Comsol Multiphysics. In the simulation, values of refractive indices are n = 3.2 + i1.8 for Ti3O5,71 n = 2.55 for TiO2 and n = 1.33 for water. The spatial distribution of resistive heating, as shown in Fig. 7a, clearly shows that the heating effect is localized into the Ti3O5 structures, which act as heat sources due to pulsed laser exposure. To probe the temporal and spatial distribution of temperatures, the following transient model has been adapted: |
 | (1) |
|
 | (2) |
|
 | (3) |
Here, Tl is the lattice temperature of Ti3O5, Ts is the temperature of TiO2 micro-substrate and Tw is water temperature. Q(t) is the transient heat due to pulsed laser irradiation. gab(Ta − Tb) is the heat transfer from medium a to b, with gab being the boundary interface heat exchange with the Ti3O5/water interface, glw = 28 × 108 W m−2 K−1; Ti/TiO2 interface gls = 28 × 108 W m−2 K−1; and TiO2/water interface gsw = 31 × 108 W m−2 K−1, calculated following ref. 72. kl = 0.4 W m−1 K−1 is the thermal conductivity of lattice and Cl = 3.6 × 106 J m−3 K−1 is the lattice specific heat of Ti3O5.73 Cw = 4182 J kg−1 K−1 and Cs = 938 J kg−1 K−1 are the heat capacities of water and TiO2 substrate (obtained from Dulong Petit law), respectively. The mass density is ρw = 1000 kg m−3 of water and ρn = 4260 kg m−3 of TiO2, respectively. The transient temperatures Tl and Tw are shown in Fig. 7b for an arbitrary point on the Ti3O5/water interface. The temperature distribution in the model at the peak of temperature transient is shown in Fig. 7c and d at 23 ns and 100 ns, respectively. It is shown that the temperature diffuses from the Ti3O5 structures to the surrounding environments after pulse laser exposure. The temperature rise is significantly high enough (>550 K) to induce cavitation bubbles at the Ti3O5/water interface.50,74 These bubbles were generated, expanded, and destroyed, eventually resulting in strong jet flow in the vicinity of the cell membrane. As a result, the cell membranes deformed, generating transient pores which allow extracellular molecules to diffuse into the cell cytosol. It is worth mentioning that we did not include any phase transitions induced by the temperature rise of the materials in the simulation. Also, note that the heating effect would be different for different shapes and sizes of materials. This model is just presented to establish the fact that the heating effect plays an important role in intracellular delivery in the microspike platform.
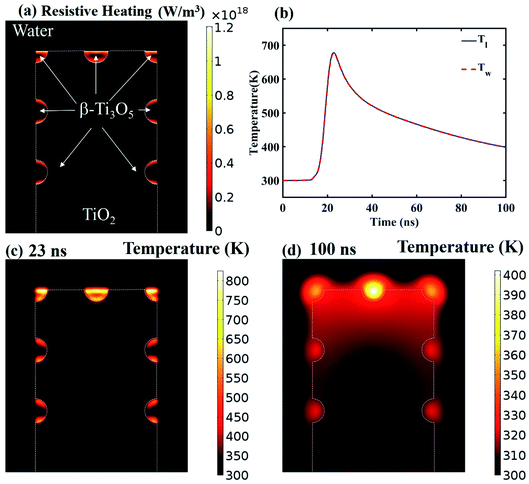 |
| Fig. 7 (a) Spatial distribution of resistive heating in the model structure. (b) Temporal heating and cooling at an arbitrary point of β-Ti3O5/water interface. (c) Spatial distribution of the temperatures at 23 ns (the peak position of the transient in (b)). (d) Spatial distribution of the temperatures at 100 ns of the transient. | |
3.4. Laser exposure on microspike device
3.4.1. Intracellular delivery. Photoporation experiments were carried out using a tunable nanosecond pulse laser. After performing the cell culture overnight on a microspike device, the culture was cleaned by PBS and PI dye was added to the device surface, and then it was exposed to a pulse laser. The PI dye is cell impermeable, and it cannot enter inside live cells until the membrane ruptures and creates transient membrane nanopores. The dye only can enter due to cell membrane deformation and produce red fluorescence color in the cell cytosol by a simple diffusion process.47,75Fig. 8 shows the schematic of pulse laser-assisted intracellular delivery using the microspike device. Due to pulse laser exposure on the microspike device, the microspikes heat up, resulting in vaporization of surrounding cell medium and the creation of cavitation bubbles. These cavitation bubbles can rapidly grow, coalesce and collapse, resulting in steady fluid flow on the microspikes–cell membrane interface. As a result, the cell membrane deformed and created transient hydrophilic nanopores, which allowed PI molecules to diffuse inside cells. After laser exposure with PI molecules, the microspike device surface was washed with PBS, and calcein-AM was added to the device and incubated for ten minutes. Calcein-AM is a cell-permeable dye, and it can cause hydrolysis inside live cells, thus producing green fluorescence.47,75
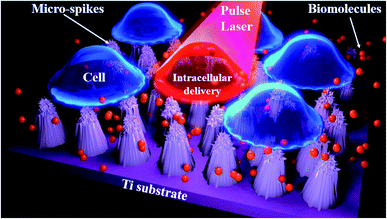 |
| Fig. 8 The schematic of pulse laser-assisted intracellular delivery using micro-spikes. Due to pulse laser interaction with micro-spikes, it heats up and induce cavitation bubbles resulting strong fluid flow at cell membrane surface. Thus, cell membrane can deform and create transient membrane pores and delivery molecules into cells. | |
Fig. 9a shows the PI dye delivery in HeLa cells using 7 mJ laser fluence at 650 nm wavelength, with 5 ns pulse width and 10 Hz pulse frequency. The red fluorescence image of the figure confirms that PI dye is delivered successfully into the HeLa cells. Fig. 9b shows calcein-AM imaging, where most of the cells are live after delivery. Fig. 9c shows the merged image of PI dye delivery and calcein-AM imaging, which also confirmed that most of the cells are live, with green and yellowish-green fluorescence. Fig. 9d–f show the same at higher magnification on different laser exposure areas of the device.
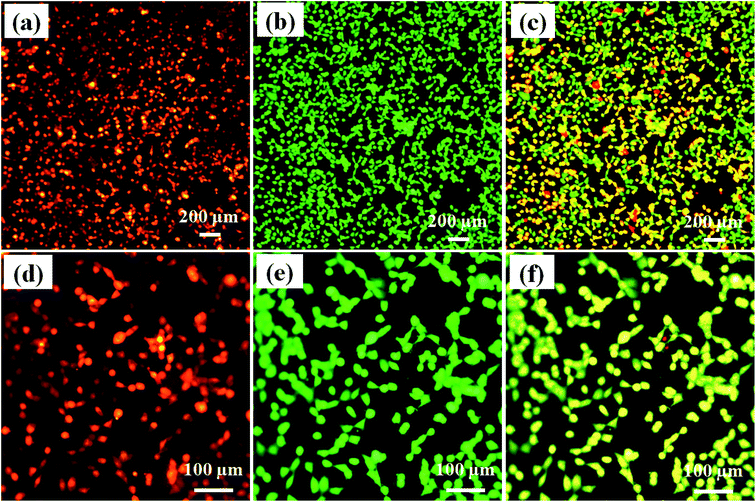 |
| Fig. 9 PI dye delivery and cell viability (a) PI dye delivery in HeLa cells (b) cell viability using calcein AM (c) merge image. (d–f) at higher magnification. | |
3.4.2. Delivery efficiency and cell viability. The delivery efficiency and cell viability reached approximately 93% and 98%, as shown in Fig. 10a. For the delivery efficiency calculation, we counted the number of fluorescent cells delivered PI dye (Fig. 10a), divided by the total number of cells and multiplied by 100%. To calculate cell viability, the same procedure was followed after calcein-AM staining and imaging (Fig. 10b).
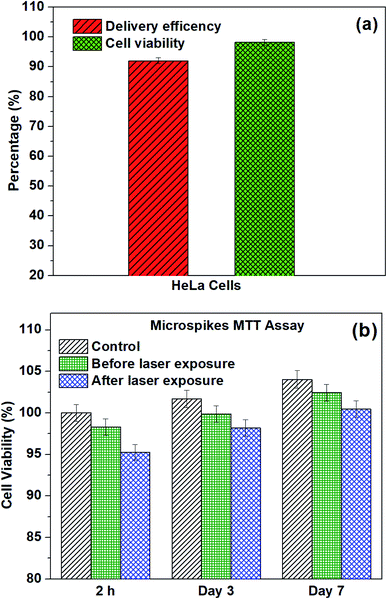 |
| Fig. 10 (a) Delivery efficiency and cell viability on microspikes using image analysis and (b) cell viability of HeLa cells using MTT assay on different days before and after laser exposure on micro-spikes. | |
Moreover, the 3-(4,5-dimethylthiazol-2-yl)-2,5-diphenyltetrazolium bromide assay was used to identify cell viability after laser exposure on microspikes with HeLa cells. To check the device biocompatibility, we also compared the microspikes device's cell viability and proliferation efficiency without laser exposure. The MTT experiments were conducted for 2 h, 3 days and 7 days on all samples to inspect cell proliferation and cell viability. Substantial improvement of viable cells adhering on the chip surface before and after laser exposure was reflected in the increased absorbance at an optical density (OD) of 570 nm after 3 and 7 days incubation. The absorbance values were converted into the percentage of cell viability, as shown in Fig. 10b. After 2 h of laser exposure (microspikes), the cell viability is ∼95.25% ± 0.95, which is ∼3% deviated from the microspike device (without laser exposure), viz ∼98.30% ± 0.98 and 5% deviation for cells only (without the device). These results also substantiate the cell viability results obtained using fluorescence images (Fig. 10a). The numbers of viable cells on microspikes further increase after incubation for 3 days (∼99.8 ± 0.99 before and 98.2% ± 0.98 after laser exposure). No apparent cytotoxicity was observed even after longer incubation (7 days). These results suggest that the fabricated device (microspikes) does not show significant cytotoxicity. However, after laser exposure, about ∼3% cell death was observed. Conversely, the number of cells and proliferation efficiency increases after incubation for 3 and 7 days. The fluorescence microscopy images confirmed the attachment of HeLa cells after laser exposure on samples. The cells have well-spread morphology, representing that they are firmly attached to the surface of the microspikes. Cell growth increases with an increase in the incubation time.
3.5. Laser exposure on a bare titanium substrate (without microspikes)
The experiments were performed on titanium substrate (without microspikes) to cross-check the delivery efficiency/mechanism. Fig. 11a shows the PI dye-stained nuclei of dead cells due to laser exposure (same parameters of microspikes) on the titanium substrate. Fig. 11b shows calcein-AM stained live cells after laser exposure. Cell viability was calculated using image analysis and MTT assay before and after laser exposure on titanium substrate, as shown in Fig. 12. These results clearly show that no PI dye delivery took place in the absence of microspikes, strongly validating that PI dye delivery into cells is mainly because of the laser and microspike interaction-based photoporation or optoporation mechanism.
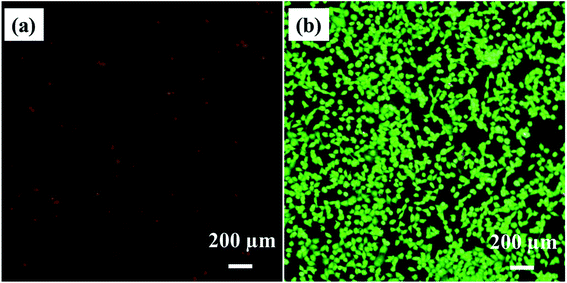 |
| Fig. 11 Laser exposure on titanium substrate (without micro-spikes) (a) PI dye staining on the nucleus of dead cells (b) cell viability test using calcein-AM confirmed that most of the cells are live (green color). | |
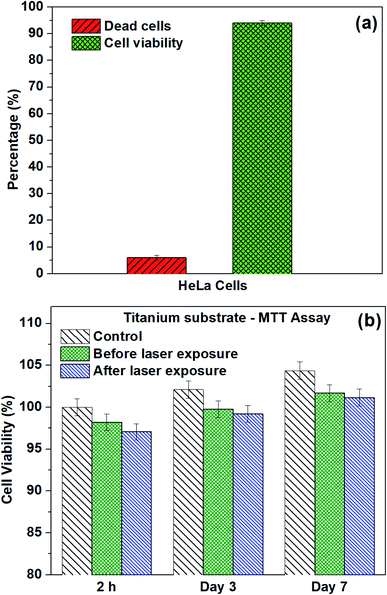 |
| Fig. 12 (a) Dead cells and viable cells after laser exposure on titanium substrate using image analysis and (b) cell viability of HeLa cells using MTT assay on different days before and after laser exposure on titanium substrate (without microspikes). | |
3.6. Intracellular delivery mechanism on microspikes
We hypothesize the possible photoporation mechanism of intracellular delivery using microspikes as follows. The PI dye is cell impermeable; it can only enter the cell and produce red fluorescence color if any mechanical membrane rupture forms by application of some external energy to create transient membrane pores. Due to pulse laser exposure on the microspike device, the microspikes heated up, vaporizing the surrounding cell medium and creating cavitation bubbles. These laser-induced cavitation bubbles rapidly grow and burst, resulting in steady fluid flow on the TiO2 microspike–cell membrane interface. As a result, the cell membrane deformed and created temporary hydrophilic nanopores, which allowed PI molecules to diffuse inside the cells.
The core-level spectra of Ti 2p and O 1s were studied by X-ray photoelectron spectroscopy (XPS). The Ti 2p spectrum of the microspike samples shows that it has Ti0, Ti2+/Ti3+, and Ti4+. Simultaneously, the O 1s spectrum validates the high concentration of oxygen vacancy defects in these samples' lattice. These defects are initiated by the Ti3+ unsaturated coordination, forming the basis for more free electrons. The oxygen defects have quasi-metallic and metallic properties, even though they are semiconducting. Atmospheric contamination shows oxides that are a few Å to nm thick, whereas the interaction between the laser and microspikes is perhaps at a few nm depth. The Ti0, Ti2+/Ti3+, and Ti4+ (on microspikes) below the oxide layer probably interact with irradiated nanosecond pulse laser to cause the laser-induced cavitational bubbles, which disrupt the cell membrane for intracellular delivery; this is not possible on the pristine titanium substrate.
The oxide formed by electrochemical anodization may be deficient in oxygen, which leads to the formation of a mixed oxide. XPS analysis indicates that the formed oxide layer mostly contains TiO2, TixOy, and Ti, where the TixOy may have quasi-metallic and the Ti metallic properties. The formed Ti3O5 and TixOy have quasi-metallic properties and exhibit absorbance in the green to red region. These oxides may photothermally create heat, PNBs, cavitational bubbles, and/or reactive oxygen species (ROS), which aid in creating resealable pores on the cells for entry of PI from the outside to the inside of the cells.76 However, the exact mechanism for intracellular delivery by the microspike-based photoporation is uncertain. It can be understood that intracellular delivery may occur due to the combination of the mechanisms stated above.
4. Conclusions
In summary, microspikes have been fabricated on titanium substrate using an electrochemical anodization process. The interaction between laser and microspikes induced cavitation bubbles, which rapidly grow, coalesce, and collapse. As a result, a strong fluid flow is generated at the microspike and cell membrane interface, which disrupts the plasma membrane and creates transient membrane pores for highly efficient intracellular delivery into the cell. We successfully delivered propidium iodide dye into HeLa cells with high delivery efficiency and high cell viability. Thus, our platform can perform highly efficient intracellular drug delivery and shows potential applicability in cellular therapy and diagnostics.
Conflicts of interest
The authors declare no conflict of interest.
Acknowledgements
We greatly appreciate the financial support from the DBT/Wellcome Trust India Alliance Fellowship under grant number IA/E/16 1 503062 and the Indian Institute of Technology Madras Exploratory research grant ED1920904RFER008754. The authors would like to thank Dr Parthasarathi Bera, Surface Engineering Division, CSIR-National Aerospace Laboratories, India, for discussion on the XPS analysis. The authors would like to thank Dr Pallavi Gupta and Ms. B. Nandhini for their help. XPS measurements were carried out using a Quantera SXM-CI, scanning X-ray Microprobe, ULVAC-PHI, INC XPS system in the Cooperative Research Facility Center at the Toyohashi University of Technology. Help from Mr Saitou Toshihide, CRFC, Toyohashi University of Technology, Japan, for XPS studies is gratefully acknowledged. The author would like to thank SAIF, IITM, for the FESEM and EDS studies.
References
- M. Kaur and K. Singh, Mater. Sci. Eng., C, 2019, 102, 844–862 CrossRef CAS.
- L. Mohan, D. Durgalakshmi, M. Geetha, T. S. N. S. Narayanan and R. Asokamani, Ceram. Int., 2012, 38, 3435–3443 CrossRef CAS.
- D. Regonini, C. R. Bowen, A. Jaroenworaluck and R. Stevens, Mater. Sci. Eng., R, 2013, 74, 377–406 CrossRef.
- K. Kalantar-zadeh, A. Z. Sadek, H. Zheng, J. G. Partridge, D. G. McCulloch, Y. X. Li, X. F. Yu and W. Wlodarski, Appl. Surf. Sci., 2009, 256, 120–123 CrossRef CAS.
- L. Mohan, C. Anandan and N. Rajendran, Mater. Sci. Eng., C, 2015, 50, 394–401 CrossRef CAS.
- C. Anandan, L. Mohan and P. D. Babu, Appl. Surf. Sci., 2014, 296, 86–94 CrossRef CAS.
- L. Mohan, C. Anandan and N. Rajendran, Electrochim. Acta, 2015, 155, 411–420 CrossRef CAS.
- L. Mohan, C. Anandan and N. Rajendran, RSC Adv., 2015, 5, 41763–41771 RSC.
- M. Ghorbani, F. Nasirpouri, A. Iraji zad and A. Saedi, Mater. Des., 2006, 27, 983–988 CrossRef CAS.
- H. E. Prakasam, O. K. Varghese, M. Paulose, G. K. Mor and C. A. Grimes, Nanotechnology, 2006, 17, 4285–4291 CrossRef CAS.
- H. Tsuchiya and P. Schmuki, Electrochem. Commun., 2005, 7, 49–52 CrossRef CAS.
- L. Mohan, S. Kar, B. Nandhini, S. S. Dhilip Kumar, M. Nagai and T. S. Santra, Mater. Today Commun., 2020, 101403 Search PubMed.
- R. L. Karlinsey, Electrochem. Commun., 2005, 7, 1190–1194 CrossRef CAS.
- Y. F. Yuan, X. H. Xia, J. B. Wu, J. L. Yang, Y. B. Chen and S. Y. Guo, Electrochem. Commun., 2010, 12, 890–893 CrossRef CAS.
- V. D. Patake and C. D. Lokhande, Appl. Surf. Sci., 2008, 254, 2820–2824 CrossRef CAS.
- E. Reimhult, K. Kumar and W. Knoll, Nanotechnology, 2007, 18, 275303 CrossRef.
- N. R. de Tacconi, C. R. Chenthamarakshan, G. Yogeeswaran, A. Watcharenwong, R. S. de Zoysa, N. A. Basit and K. Rajeshwar, J. Phys. Chem. B, 2006, 110, 25347–25355 CrossRef CAS.
- K. Kalantar-zadeh, A. Z. Sadek, J. G. Partridge, D. G. McCulloch, Y. X. Li, X. F. Yu, P. G. Spizzirri and W. Wlodarski, Thin Solid Films, 2009, 518, 1180–1184 CrossRef CAS.
- K. Indira, S. Ningshen, U. K. Mudali and N. Rajendran, Mater. Charact., 2012, 71, 58–65 CrossRef CAS.
- K. Indira, U. K. Mudali and N. Rajendran, Ceram. Int., 2013, 39, 959–967 CrossRef CAS.
- L. Mohan, S. Viswanathan, C. Anandan and N. Rajendran, RSC Adv., 2015, 5, 93131–93138 RSC.
- S. Viswanathan, L. Mohan, S. John, P. Bera and C. Anandan, Surf. Interface Anal., 2017, 49, 450–456 CrossRef CAS.
- S. Viswanathan, L. Mohan, P. Bera and C. Anandan, RSC Adv., 2016, 6, 74493–74499 RSC.
- H. Wu, Y. Wang, Y. Ma, T. Xiao, D. Yuan and Z. Zhang, Ceram. Int., 2015, 41, 2527–2532 CrossRef CAS.
- L. Mohan, C. Dennis, N. Padmapriya, C. Anandan and N. Rajendran, Mater. Today Commun., 2020, 101103 CrossRef CAS.
- M. Supernak-Marczewska, A. Ossowska, P. Strąkowska and A. Zieliński, Adv. Mater. Sci., 2018, 18, 17–23 CAS.
- Essentials of single-cell analysis: concepts, applications and future prospects, ed. F.-G. Tseng and T. S. Santra, Springer, 2016 Search PubMed.
- T. S. Santra and F.-G. Tseng, Handbook of Single-cell Technologies, Springer Verlag, Singapore, 2021 Search PubMed.
- D. W. Pack, A. S. Hoffman, S. Pun and P. S. Stayton, Nat. Rev. Drug Discovery, 2005, 4, 581–593 CrossRef CAS.
- Z. Gu, A. Biswas, M. Zhao and Y. Tang, Chem. Soc. Rev., 2011, 40, 3638–3655 RSC.
- P. Shinde, A. Kumar, K. Illath, K. Dey, L. Mohan, S. Kar, T. K. Barik, J. Sharifi-Rad, M. Nagai and T. S. Santra, in Delivery of Drugs, Elsevier, 2020, pp. 161–190 Search PubMed.
- J. Li and K. Kataoka, J. Am. Chem. Soc., 2021, 143(2), 538–559 CrossRef CAS.
- S. Nayak and R. W. Herzog, Gene Ther., 2010, 17, 295–304 CrossRef CAS.
- R. M. Schmid, H. Weidenbach, G. F. Draenert, S. Liptay, H. Lührs and G. Adler, Gut, 1997, 41, 549–556 CrossRef CAS.
- S. Lakshmanan, G. K. Gupta, P. Avci, R. Chandran, M. Sadasivam, A. E. S. Jorge and M. R. Hamblin, Adv. Drug Delivery Rev., 2014, 71, 98–114 CrossRef CAS.
- P. Shinde, L. Mohan, A. Kumar, K. Dey, A. Maddi, A. N. Patananan, F. G. Tseng, H. Y. Chang, M. Nagai and T. S. Santra, Int. J. Mol. Sci., 2018, 19(10), 3143 CrossRef.
- A. Kumar, L. Mohan, P. Shinde, H.-Y. Chang, M. Nagai and T. S. Santra, in Handbook of Single Cell Technologies, Springer, Singapore, 2018, pp. 1–29 Search PubMed.
- Z. C. Hartman, D. M. Appledorn and A. Amalfitano, Virus Res., 2008, 132, 1–14 CrossRef CAS.
- R. G. Crystal, Science, 1995, 270, 404–410 CrossRef CAS.
- M. R. Capecchi, Cell, 1980, 22, 479–488 CrossRef CAS.
- T. S. Santra and F. G. Tseng, Micromachines, 2013, 4, 333–356 CrossRef.
- S. Kar, L. Mohan, K. Dey, P. Shinde, H.-Y. Chang, M. Nagai and T. S. Santra, J. Micromech. Microeng., 2018, 28, 123002 CrossRef CAS.
- D. H. Fuller, P. Loudon and C. Schmaljohn, Methods, 2006, 40, 86–97 CrossRef CAS.
- S. Ohta, K. Suzuki, Y. Ogino, S. Miyagawa, A. Murashima, D. Matsumaru and G. Yamada, Dev., Growth Differ., 2008, 50, 517–520 CrossRef CAS.
- A. Sharei, J. Zoldan, A. Adamo, W. Y. Sim, N. Cho, E. Jackson, S. Mao, S. Schneider, M.-J. Han, A. Lytton-Jean, P. A. Basto, S. Jhunjhunwala, J. Lee, D. A. Heller, J. W. Kang, G. C. Hartoularos, K.-S. Kim, D. G. Anderson, R. Langer and K. F. Jensen, Proc. Natl. Acad. Sci. U. S. A., 2013, 110, 2082–2087 CrossRef CAS.
- M. Hima, G. Pallavi, L. Mohan, M. Nagai, S. Wankhar and T. S. Santra, in Microfluidics and Bio-MEMS Devices and Applications, ed. T. S. Santra, Jenny Stanford Publisher Pvt. Ltd., Singapore, 2020, p. 68 Search PubMed.
- T. S. Santra, S. Kar, C.-W. Chen, J. Borana, T.-C. Chen, M.-C. Lee and F.-G. Tseng, Nanoscale, 2020, 12, 12057 RSC.
- T. S. Santra, P.-C. Wang, H.-Y. Chang and F.-G. Tseng, Appl. Phys. Lett., 2013, 103, 233701 CrossRef.
- T. S. Santra, S. Kar, H.-Y. Chang and F.-G. Tseng, Lab Chip, 2020, 20, 4194–4204 RSC.
- P. Shinde, S. Kar, L. Mohan, H.-Y. Chang, F.-G. Tseng, M. Nagai and T. S. Santra, ACS Biomater. Sci. Eng., 2020, 6(10), 5645–5652 CrossRef CAS.
- I. Lentacker, I. De Cock, R. Deckers, S. C. De Smedt and C. T. W. Moonen, Adv. Drug Delivery Rev., 2014, 72, 49–64 CrossRef CAS.
- I. Horcas, R. Fernández, J. M. Gomez-Rodriguez, J. Colchero, J. Gómez-Herrero and A. M. Baro, Rev. Sci. Instrum., 2007, 78, 13705 CrossRef CAS.
- L. Mohan, M. Chakraborty, S. Viswanathan, C. Mandal, P. Bera, S. T. Aruna and C. Anandan, Surf. Interface Anal., 2017, 49(9), 828–836 CrossRef CAS.
- L. Mohan, M. D. Raja, T. S. Uma, N. Rajendran and C. Anandan, J. Mater. Eng. Perform., 2016, 25, 1508–1514 CrossRef CAS.
- N. Liu, X. Chen, J. Zhang and J. W. Schwank, Catal. Today, 2014, 225, 34–51 CrossRef CAS.
- Q. Chen, G. H. Du, S. Zhang and L.-M. Peng, Acta Crystallogr., Sect. B: Struct. Sci., 2002, 58, 587–593 CrossRef CAS.
- T. Gao, H. Fjellvåg and P. Norby, Inorg. Chem., 2009, 48, 1423–1432 CrossRef CAS.
- M. T. Aytekin Aydın, H. L. Hoşgün, A. Dede and K. Güven, Spectrochim. Acta, Part A, 2018, 205, 503–507 CrossRef.
- L. Stagi, C. M. Carbonaro, R. Corpino, D. Chiriu and P. C. Ricci, Phys. Status Solidi, 2015, 252, 124–129 CrossRef CAS.
- M. Salis, P. C. Ricci and A. Anedda, J. Raman Spectrosc., 2009, 40, 64–66 CrossRef CAS.
- A. Ould-Hamouda, H. Tokoro, S.-I. Ohkoshi and E. Freysz, Chem. Phys. Lett., 2014, 608, 106–112 CrossRef CAS.
- Y. Li, H. Bai, J. Zhai, W. Yi, J. Li, H. Yang and G. Xi, Anal. Chem., 2019, 91, 4496–4503 CrossRef CAS.
- L. Mohan and C. Anandan, Appl. Surf. Sci., 2013, 282, 281–290 CrossRef CAS.
- L. Mohan and C. Anandan, Appl. Surf. Sci., 2013, 268, 288–296 CrossRef CAS.
- C. Anandan and L. Mohan, J. Mater. Eng. Perform., 2013, 22, 3507–3516 CrossRef CAS.
- V. V Atuchin, V. G. Kesler, N. V Pervukhina and Z. Zhang, J. Electron Spectrosc. Relat. Phenom., 2006, 152, 18–24 CrossRef.
- J. Yan, Z. Lin, C. Ma, Z. Zheng, P. Liu and G. Yang, Nanoscale, 2016, 8, 15001–15007 RSC.
- H. Hernandez-Arriaga, E. Lopez-Luna, E. Martinez-Guerra, M. M. Turrubiartes, A. G. Rodriguez and M. A. Vidal, J. Appl. Phys., 2017, 121, 64302 CrossRef.
- B. Bharti, S. Kumar, H.-N. Lee and R. Kumar, Sci. Rep., 2016, 6, 32355 CrossRef CAS.
- K. R. Goodman, J. Wang, Y. Ma, X. Tong, D. J. Stacchiola and M. G. White, J. Chem. Phys., 2020, 152(5), 054714 CrossRef CAS.
- R. Liu, J. X. Shang and F. H. Wang, Comput. Mater. Sci., 2014, 81, 158–162 CrossRef CAS.
- G. Chang, F. Sun, J. Duan, Z. Che, X. Wang, J. Wang, M. J. Kim and H. Zhang, Acta Mater., 2018, 160, 235–246 CrossRef CAS.
- H. Tokoro, M. Yoshikiyo, K. Imoto, A. Namai, T. Nasu, K. Nakagawa, N. Ozaki, F. Hakoe, K. Tanaka, K. Chiba, R. Makiura, K. Prassides and S. I. Ohkoshi, Nat. Commun., 2015, 6, 1–8 Search PubMed.
- J. Lombard, T. Biben and S. Merabia, J. Phys. Chem. C, 2017, 121, 15402–15415 CrossRef CAS.
- T. S. Santra, C.-W. Chen, H.-Y. Chang and F.-G. Tseng, RSC Adv., 2016, 6, 10979–10986 RSC.
- L. Mohan, S. Kar, R. Hattori, M. Ishii-Teshima, P. Bera, S. Roy, T. Subhra Santra, T. Shibata and M. Nagai, Appl. Surf. Sci., 2021, 543, 148815 CrossRef CAS.
|
This journal is © The Royal Society of Chemistry 2021 |