DOI:
10.1039/D0RA08815C
(Paper)
RSC Adv., 2021,
11, 3071-3078
Density functional theory design of double donor dyes and electron transfer on dye/TiO2(101) composite systems for dye-sensitized solar cells†
Received
16th October 2020
, Accepted 17th December 2020
First published on 13th January 2021
Abstract
In this work, we designed a series of double donor organic dyes, named ME101–ME106, based on experimentally synthesized dye WD8, and further investigated their electronic structure, the stability of the dye/TiO2 (101) systems, density of states (DOS) and absorption spectra using density functional theory (DFT) and time-dependent DFT (TDDFT). The molar extinction coefficients of all designed dyes are higher than WD8. It's fascinating that ME106 exhibits a smallest energy gap and 75 nm redshifts compared to WD8. The results of calculations reveal that ME101–ME106/TiO2(101) surfaces are more stable than WD8, double donor dyes have sufficient electron injection driving force and have very strong transfer electron ability. It is expected that the design of double donors can provide a new understanding and guidance for the investigation of high efficiency dye-sensitized devices.
1. Introduction
Dye sensitized solar cells (DSSCs) have attracted significant interest owing to their high efficiency, easy manufacturing process and their low-cost, and have become one of the most promising photovoltaic devices.1–7
DSSCs have a typical sandwich structure, mainly including dye molecules, a metal oxide layer, an electrolyte and a counter electrode. A pivotal component “photosensitizer” is excited by harvesting light and then the conductor band (CB) of TiO2 accepts the photo-induced electrons with electricity generation. Especially, the sensitizers play a critical role in influencing the photoelectric conversion efficiency (PCE).8,9 Till now, ruthenium-polypyridyl complexes and metal-free organic dyes have been investigated commonly.10–13 Conventional Ru-complexes, such as N3/N719 have shown the recorded PCE exceeding 11%,14–16 and the PCE of zinc porphyrin sensitizers has reached 13%.17,18 At the same time, more efforts have been turned to design and explore metal-free organic dyes. P. Wang synthesized a new pure organic dye, C281–286, which exhibits high PCE of exceed 13.0%.19 There are also photosensitizers for colorful and semitransparent dye-sensitized solar cells, which increase its aesthetics while ensuring efficiency.20–22 And DSSC has effective output power under various lighting conditions, and even has excellent performance under dim conditions, thus paving way for the self-powered indoor light harvesting Internet of Things (IoT) applications.23–25 These give us more attention to develop more potential organic sensitizers for DSSCs. Up to now, various structures organic dyes are composed of electron donors (D), π-bridges (π) and acceptor moieties (A). At present, there are methods to obtain the PCE of DSSCs with D–π–A structure using only the results from density functional theory (DFT) and time-dependent DFT calculations.26 In 2019, Zhang et al. further explored the molecular structure and internal electron transfer mechanism of TH series dyes (D–A–π–A).27 In 2008, Ning et al. firstly proposed a new D–D–π–A configuration for improving the performance of a DSSC.28 The additional electron donor in D–D–π–A structure is beneficial not only enhance light absorption capacity, but also inhibit the dye aggregation.29–31 For example, Dai et al. incorporated the M45 sensitizer based on D–D–π–A form with overall power conversion efficiency of 9.02%.32 These suggest that D–D–π–A sensitizers will have more brilliant advantages in DSSCs fields.
Dye sensitizers and semiconductor photoanodes are the core of dye-sensitized solar cells. It is important that the individual dye molecule has excellent performance. However, after the dye is adsorbed on TiO2 surface, the anchoring group will interact with the semiconductor. The excited state electron can be effectively injected into the semiconductor, which is a key process regulating the PCE of the DSSCs. Dye-sensitized solar cells have made great progress, but it is still a difficult problem to accurately investigate the internal electron transfer mechanism of DSSCs. In fact, it turns out to be exertive for giving an in-depth interpretation of the experimental method from the atomic level. Therefore, we use theoretical simulation methods to analyze the electron transfer mechanism. In 2012, Wan et al. newly synthesized double electron donor type organic dye WD8 using phenothiazine as donor group, furan as π-bridge and carboxylic acid as acceptor group.33 In this work, we designed a range of organic dye ME101–ME106 by replacing the WD8's donor group in order to seek more promising organic dyes, as shown in Fig. 1. Moreover, we present a systematic investigation on the influence of the dye/TiO2 on their electronic and optical properties. The geometry of the dye/TiO2 complex system, electron injection, absorption spectra, and lifetime of the excited state were studied in order to evaluate the performance of the adsorbed systems in DSSCs.
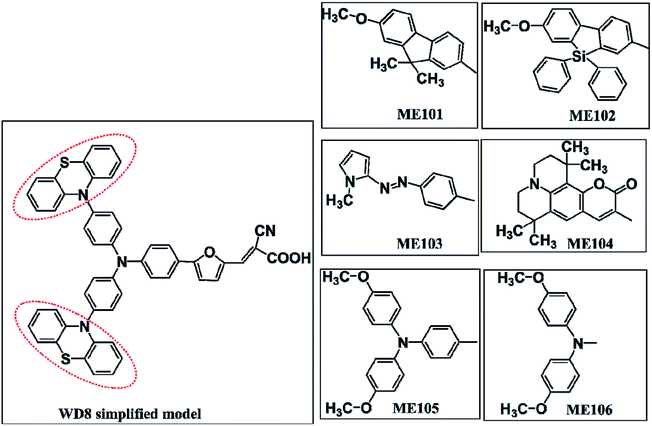 |
| Fig. 1 Molecular structures of dyes WD8 and ME101–ME106. | |
2. Computational methods
All the ground-state geometries were optimized by DFT combined with B3LYP functional in acetonitrile solution with Gaussian 09.34–37 The excitation states were calculated by CAM-B3LYP/6-31+G*/LanL2DZ for Ti atoms in acetonitrile using conductor like polarizable continuum model (CPCM).38,39 DFT/DFT+U calculations of dye/TiO2 were operated on this model using the Vienna Ab initio Simulation Package (VASP).40,41 The Perdew–Burke–Ernzerh (PBE) methods based on generalized gradient-approximation (GGA) were are carried out in all calculations. DFT+U correction is for titanium on the 3d orbital, and the band gap and lattice parameters of TiO2 were applied: U = 6 eV and J = 0.5 eV. All methods were believed to access the reliable results compared to the experimental values.42–44
3. Results and discussion
3.1 Electronic structure and stability of the dye/TiO2 (101) system
The excellent performance of the dye molecules depends on individual dye molecules and stability of the dye/TiO2, which improve photon-to-current conversion efficiency. The structures of WD8 and ME101–ME106 were optimized (Fig. S1†), and further energy levels and absorption spectra for WD8 and ME101–ME106 were calculated, as shown in Fig. 2 and 3. We can clearly see that the LUMOs of all dyes are higher than the conduction band edge (CBE) of TiO2 (about −4.0 eV) and HOMOs are lower than the potential (about −4.8 eV) of the I−/I3− redox shuttle.45 That is to say, these dyes possess enough injection driving force and driving force, so as to ensure the transfer of electrons from the double electron donor to the acceptor (Fig. S2†). In addition, the energy gaps decrease in the sequence of WD8 > ME101 > ME102> ME103 > ME104 > ME105 > ME106, which has a favorable characteristic lead to harvest longer light. From Fig. 3(a), it is obvious that the absorption spectra of the four dyes, ME101, ME102, ME105, and ME106, have red-shifts compared to WD8, especially maximum redshifts of ME106, which is consistent with the smallest HOMO–LUMO energy gap. From Fig. 3(b), the absorption spectra of ME103 and ME104 show a blue shift. The main reason is that the ME103 molecule contains a nitrogen–nitrogen double bond, which mainly undergoes n–π* transitions, and blue shifts easily occur in the polar solution of acetonitrile. The ME104 molecule contains –OCO– group, which is also prone to blue shift. Although the ME103 and ME104 molecules have only one absorption peak (perhaps because the other absorption peak is weaker, only one absorption peak is displayed), but their molar extinction coefficient increase significantly, the light absorption area of ME103 and ME104 are also much larger than that for WD8.
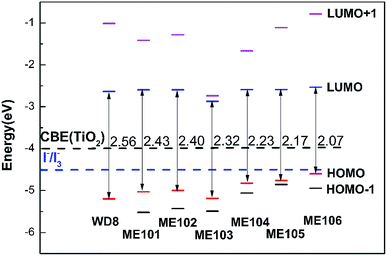 |
| Fig. 2 The energy levels of WD8 and ME101–ME106. | |
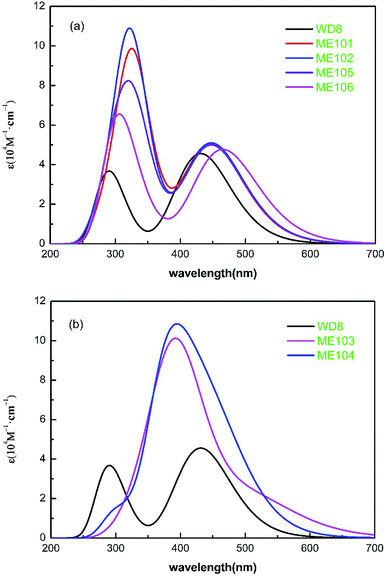 |
| Fig. 3 The calculated UV-visible absorption spectra of all dyes. (a) WD8, ME101, ME102, ME105 and ME106 (b) WD8, ME103 and ME104. | |
After calculation of the isolated dyes, a bidentate chemisorption configuration of the carboxylate of the dye attached to the TiO2 has been adopted. In other words, the two O atoms of the carboxylic acid in the dye molecules are combined with the Ti atom with a 5-bond to form an O–Ti bond, respectively. The remaining H atoms are attached to the O atom on the corresponding TiO2 substrate, and the optimized structure of ME101–ME106/TiO2(101) are shown in Fig. 4. As can be seen from the figure, all the dyes are double-donor dye sensitizers and the different electron donor, but all the dye molecules are “T” structures with good verticality. These dyes have a very good planar structure, which facilitates the injection of electrons from the donor to the TiO2 substrate. We calculated the binding energies of dyes/TiO2 and the O–Ti bond lengths of the bidentate bridges. The adsorption energy is estimated as: Eads = Eslab + Edye − Edye/slab, where Eads presents the adsorption energy of the composite system, Eslab is the energy of the slab supercell of the TiO2 anatase (101) surface, Edye is the energy of the sensitizer, and Edye/slab represents the total energy of the dye/TiO2 (101) composite system. The Eads value is greater than zero, indicating that the bidentate adsorption structure is stable, the larger the Eads value, the more stable adsorption.
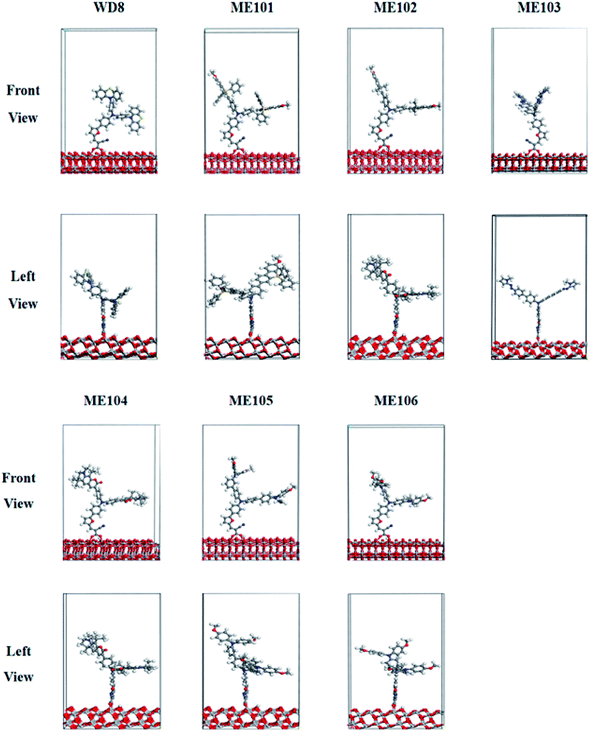 |
| Fig. 4 The configurations of WD8 and ME101–ME106 on TiO2 anatase (101). | |
3.2 Energy level alignment structure of the dye/TiO2 (101) system
We theoretically calculated the projected density of states (DOS) for ME101–ME106/TiO2, given in Fig. 5 and S3.† The Fermi level is the energy equaling to zero and other energies are relative to Ef. It can be seen that the calculated HOMO energy levels of all dyes are between the semiconductor conduction band and the valence band (VB), which helps to reduce the band gap of dye-sensitized solar. The narrow band gap promotes the absorption of more long-wavelength light and increases the electron transfer rate at the interface. However, the low energy level of TiO2 is not beneficial to improve Voc. The LUMO levels of the dyes are all located in the CB of the TiO2. It is noted that energy level alignment ensures ultrafast interfacial electron injection from the dye to TiO2 conduction band and reduces the chance of recombination between the injected electrons in CB of TiO2 and the oxidized dyes. As depicted in Fig. 5 and S3,† the LUMO orbital energy level of ME101–ME106 dye molecules is higher than that of WD8, which the newly designed dye molecules are coupled with TiO2 conduction band, and also implies the larger injection driving of ME101–ME106. Therefore, the dye/semiconductor composite system can effectively transfer and inject electrons, which is expected to improve the performance of DSSCs.
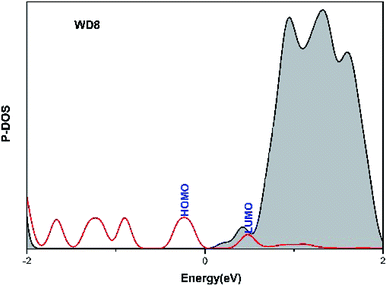 |
| Fig. 5 Calculated projected density of states for WD8. Red curves are for the dyes and the black lines are for the TiO2 substrate. | |
The calculated the energy gaps of isolated dyes WD8 and ME101–ME106 are 2.56, 2.43, 2.40, 2.32, 2.23, 2.17, and 2.07 eV, respectively. It is worth noting that when dyes were adsorbed on the surface of TiO2, the energy gaps of all dye molecules decreased to 1.25, 1.13, 1.10, 1.02, 0.94, 0.87, and 0.77 eV. The results show that when the dyes have been adsorbed, which indicate the same tendency the energy gaps of dyes decrease with the absorption range for the molecules is increased. These findings suggest that changes in the donor group of dye molecules can affect the charge transfer mechanism from the dye to the TiO2 surface and the energy-level arrangement of the dye/TiO2 (101) system.
3.3 Absorption spectra of the dye/TiO2 system
The UV-visible absorption spectra of isolated dye molecules reveal that the newly designed dual-donor dyes have wider absorption spectrum than WD8 dye, but the HOMO orbital of the dye molecules adsorbed on the TiO2 substrate changes significantly, which indicates that the dye/TiO2 system has a certain impact on UV-visible absorption spectra. In order to verify that the absorption spectrum of adsorbed dyes will be very different from the absorption spectrum of isolated dyes. According to Peng et al.19 recently proposed the model, we consider as a first approximation that the dye is connected to one TiO2 unit. The binding model is in perfect accordance with the recent results obtained which have demonstrated from infrared spectroscopy analysis in CH2Cl2 solution. Therefore, in order to save computing costs, we use the one TiO2 unit instead of the entire larger TiO2 substrate to simulate the absorption spectrum of the dye/TiO2 system. The two O atoms of the anchor group are linked to one Ti atom by a bidentate bonding bridge, with one hydrogen being isolated as one proton on the surface 2c-O atom. The optimized geometry of the dyes ME106 and WD8 adsorbing TiO2 units are shown in Fig. 6. Based on the optimized structure, the excited state of the adsorbed dye was calculated by TD-CAM-B3LYP, are presented in Fig. 7. It can be noticed that the absorption spectrum of the adsorbed dye is basically consistent with the absorption spectrum of the isolated dye. There are two absorption peaks, and the main absorption peak of the dye/TiO2 is superior to isolated dye's, which may be mainly related to the strong interaction between TiO2 and anchor group of the dye. However, its secondary peak has decreased, which agrees with the transition from π to π*. The UV-visible absorption spectrum of the dye/TiO2 is overall red-shifted, owing to the smaller energy gap of the dye/TiO2, and at the same time, the oscillator strength is also significantly increased. This is a key factor for assessing the photophysical properties of dyes. Therefore, the ME106 may be theoretically a good candidate as sensitizer of DSSC device.
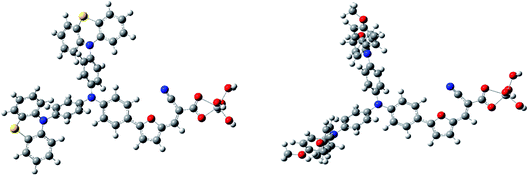 |
| Fig. 6 The geometries of dye WD8 and ME106 binding with the minimal TiO2 cluster. | |
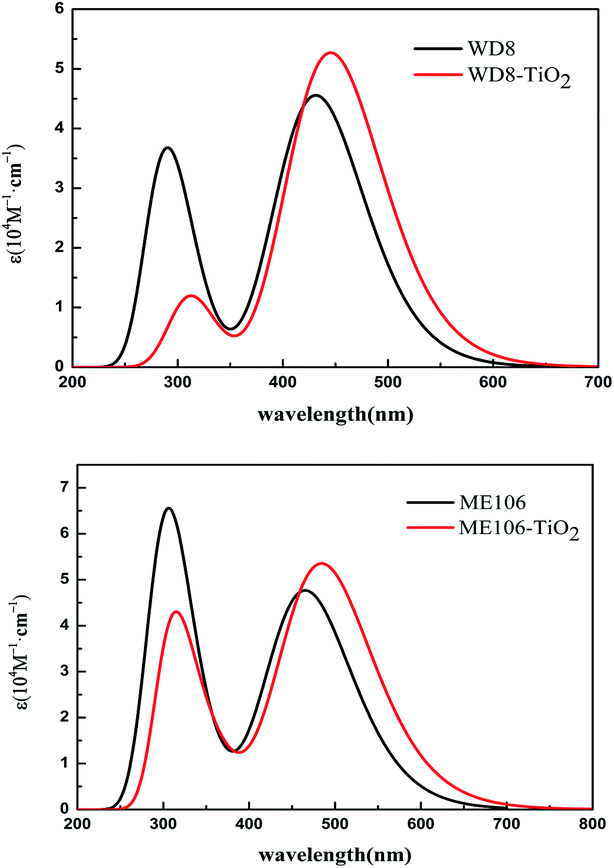 |
| Fig. 7 The UV-visible absorption spectra for isolated dyes and the adsorbed dyes. | |
4. Conclusions
In this work, we present a systematic theoretical study on a new double electron donor organic dyes ME101–ME106 based on the experimental synthesis organic dye WD8. We find that the isolated dyes ME101–ME106 have the smaller energy gap and the broaden absorption spectrum compared to WD8. The calculated results show that ME101–ME106 possessed more stable adsorption on the TiO2 surface compared to WD8. In addition, the density of states and the absorption spectra of the dye/TiO2 were calculated by first-principles calculations. Series of characterizations support the roles of the dye/TiO2 can effectively transfer and inject electrons. The HOMO–LUMO energy gap is reduced and the absorption spectra overall red shift. In theory, ME101–ME106 may have greater short-circuit photocurrent and open-circuit photovoltage. It is expected that the design of double donors can provide a new strategy and guidance for the investigation in high efficiency dye-sensitized devices.
Conflicts of interest
There are no conflicts to declare.
Acknowledgements
This work is supported by Shanxi Province Science and Technology Major Project (20181101013), the China Scholarship Council Fund (201506445024) and the Higher Education Young Elite Teacher Project (2462015YQ0603) from CUP (China University of Petroleum-Beijing).
References
- F. Bella, C. Gerbaldi, C. Barolo and M. Grätzel, Aqueous dye-sensitized solar cells, Chem. Soc. Rev., 2015, 44(44), 3431–3473 RSC.
- A. Hafeldt, G. Boschloo, L. C. Sun, L. Kloo and H. Pettersson, Dye-sensitized solar cells, Chem. Rev., 2010, 110(110), 6595–6663 CrossRef.
- B. O'Regan and M. Grätzel, A low-cost, high-efficiency solar cell based on dye-sensitized colloidal TiO2 films, Nature, 1991, 353, 737–740 CrossRef.
- J. Baxter and E. Aydil, Nanowire-based dye-sensitized solar cells, Appl. Phys. Lett., 2005, 86(86), 053114 CrossRef.
- Y. J. Chang and T. J. Chow, Highly efficient triarylene conjugated dyes for sensitized solar cells, J. Mater. Chem., 2011, 21(26), 9523–9531 RSC.
- C. H. Huang and Y. J. Chang, Dyes for sensitized solar cells by using [2.2]paracyclophane as a bridging unit, Tetrahedron Lett., 2014, 35(55), 4938–4942 CrossRef.
- N. Duvva, Y. K. Eom, G. Reddy, K. S. Schanze and L. Giribabu, Bulky Phenanthroimidazole–Phenothiazine D–π–A Based Organic Sensitizers for Application in Efficient Dye-Sensitized Solar Cells, ACS Appl. Energy Mater., 2020, 3(7), 6758–6767 CrossRef CAS.
- A. Mishra, M. K. R. Fischer and P. Bäuerle, Metal-free organic dyes for dye-sensitized solar cells: form structure: property relationships to design rules, Angew. Chem., Int. Ed., 2009, 48(48), 2474–2499 CrossRef CAS.
- C. Kim, T. W. Kim, S. Kim, I. Oh, H. Wonneberger, K. Yoon, M. Kwak, J. Kim, J. Kim, C. Li, K. Müllen and H. Ihee, Molecular-Level Understanding of Excited States of N-Annulated Rylene Dye for Dye-Sensitized Solar Cells, J. Phys. Chem. C, 2020, 124(42), 22993–23003 CrossRef CAS.
- K. Pei, Y. Wu, A. Islam, Q. Zhang, L. Han, H. Tian and W. Zhu, Constructing high-efficiency D–A–π–A-featured solar cell sensitizers: a promising building block of 2,3-diphenylquinoxaline for antiaggregation and photostability, ACS Appl. Mater. Interfaces, 2013, 5(5), 4986–4995 CrossRef CAS.
- T. Sudyoadsuk, J. Khunchalee, S. Pansay, P. Tongkasee, S. Morada, T. Kaewin, S. Jungsuttiwong and V. Promarak, An organic dye using N-dodecyl-3-(3,6-di-tert-butylcarbazol-N-yl)carbazol-6-yl as a donor moiety for efficient dye-sensitized solar cells, Tetrahedron Lett., 2013, 54(54), 4903–4907 CrossRef CAS.
- C. L. Gray, P. Xu, A. J. Rothenberger, S. J. Koehler, E. Elacqua, B. H. Milosavljevic and T. E. Mallouk, Oligomeric Ruthenium Polypyridyl Dye for Improved Stability of Aqueous Photoelectrochemical Cells, J. Phys. Chem. C, 2020, 124(6), 3542–3550 CrossRef CAS.
- K. T. Mukaddem, P. A. Chater, L. R. Devereux, O. K. Al Bahri, A. Jain and J. M. Cole, Dye-Anchoring Modes at the Dye⋯TiO2 Interface of N3- and N749-Sensitized Solar Cells Revealed by Glancing-Angle Pair Distribution Function Analysis, J. Phys. Chem. C, 2020, 124(22), 11935–11945 CrossRef CAS.
- M. K. Nazeeruddin, P. Pechy and M. Grätzel, Efficient panchromatic sensitization of nanocrystalline TiO2 films by a black dye based on a trithiocyanato-ruthenium complex, Chem. Commun., 1997, 1705–1706 RSC.
- M. K. Nazeeruddin, A. Kay, I. Rodicio, R. Humphry-Baker, E. Mueller, P. Liska, N. Vlachopoulos and M. Grätzel, Conversion of light to electricity by cis-X2bis (2,2′-bipyridyl-4,4′-dicarboxylate)ruthenium(II) charge-transfer sensitizers (X = Cl-, Br-, I-, CN-, and SCN-) on nanocrystalline titanium dioxide electrodes, J. Am. Chem. Soc., 1993, 115(115), 6382–6390 CrossRef CAS.
- K. Portillo-Cortez, et al., N719 Derivatives for Application in a Dye-Sensitized Solar Cell (DSSC): A Theoretical Study, J. Phys. Chem.
A, 2019, 123(51), 10930–10939 CrossRef CAS.
- S. Mathew, A. Yella, P. Gao, R. Humphry-Baker, B. F. Curchod, N. Ashari-Astani, I. Tavernelli, U. Rothlisberger, M. K. Nazeeruddin and M. Gratzel, Dye-sensitized solar cells with 13% efficiency achieved through the molecular engineering of porphyrin sensitizers, Nat. Chem., 2014, 6(6), 242–247 CrossRef CAS.
- J.-J. Fu, Y.-A. Duan, J.-Z. Zhang, M.-S. Guo and Y. Liao, Theoretical investigation of novel phenothiazine-based D–π–A conjugated organic dyes as dye-sensitizer in dye-sensitized solar cells, Theor. Chem., 2014, 1045, 145–153 CAS.
- Z. Yao, H. Wu, Y. Li, J. Wang, J. Zhang, M. Zhang, Y. Guo and P. Wang, Dithienopicenocarbazole as the kernel module of low-energy-gap organic dyes for efficient conversion of sunlight to electricity, Energy Environ. Sci., 2015, 8(8), 3192–3197 RSC.
- X. R. Xie, D. Y. Sun, Y. F. Wei, Y. Yuan, J. Zhang, Y. T. Ren and P. Wang, Thienochrysenocarbazole based organic dyes for transparent solar cells with over 10% efficiency, J. Mater. Chem. A, 2019, 7(18), 11338–11346 RSC.
- Y. Ren, Y. Cao, D. Zhang, S. M. Zakeeruddin, A. Hagfeldt, P. Wang and M. Grätzel, A Blue Photosensitizer Realizing Efficient and Stable Green Solar Cells via Color Tuning by the Electrolyte, Adv. Mater., 2020, 32(17), 2000193 CrossRef CAS.
- H. Wu, J. Zhang, Y. Ren, Y. Zhang, Y. Yuan, Z. Shen, S. Li and P. Wang, Tuning the Color Palette of Semi-Transparent Solar Cells via Lateral π-Extension of Polycyclic Heteroaromatics of Donor–Acceptor Dyes, ACS Appl. Energy Mater., 2020, 3(5), 4549–4558 CrossRef CAS.
- A. Aslam, et al., Dye-sensitized solar cells (DSSCs) as a potential photovoltaic technology for the self-powered internet of things (IoTs) applications, Sol. Energy, 2020, 207, 874–892 CrossRef CAS.
- M. L. Parisi, et al., Prospective life cycle assessment of third-generation photovoltaics at the pre-industrial scale: a long-term scenario approach, Renewable Sustainable Energy Rev., 2020, 121, 109703 CrossRef.
- G. Gokul, S. C. Pradhan and S. Soman, Dye-Sensitized Solar Cells as Potential Candidate for Indoor/Diffused Light Harvesting Applications: From BIPV to Self-powered IoTs, in Advances in Solar Energy Research, ed. H. Tyagi, A. K. Agarwal, P. R. Chakraborty and S. Powar, Springer Singapore, Singapore, 2019, pp. 281–316 Search PubMed.
- Z. Yang, K. Li, C. Lin, L. R. Devereux, W. Zhang, C. Shao, J. M. Cole and D. Cao, Predicting Device Parameters for Dye-Sensitized Solar Cells from Electronic Structure Calculations to Reproduce Experiment, ACS Appl. Energy Mater., 2020, 3(5), 4367–4376 CrossRef CAS.
- J. Jie, Q. Xu, G. Yang, Y. Feng and B. Zhang, Porphyrin sensitizers involving a fluorine-substituted benzothiadiazole as auxiliary acceptor and thiophene as π bridge for use in dye-sensitized solar cells (DSSCs), Dyes Pigm., 2020, 174, 107984 CrossRef CAS.
- Z. Ning, Q. Zhang, W. Wu, H. Pei, B. Liu and H. Tian, Starburst triarylamine based dyes for efficient dye-sensitized solar cells, J. Org. Chem., 2008, 73(73), 3791–3797 CrossRef CAS.
- S. Wang, H. R. Wang, J. C. Guo, H. B. Tang and J. Z. Zhao, Influence of the terminal electron donor in D–D–π–A phenothiazine dyes for dye-sensitized solar cells, Dyes Pigm., 2014, 109(109), 96–104 CrossRef CAS.
- T. Sudyoadsuk, S. Pansay, S. Morada, R. Rattanawan and S. Namuangruk, Synthesis and characterization of D–D–π–A-type organic dyes bearing carbazole–carbazole as a donor moiety (D–D) for efficient dye-sensitized solar, Eur. J. Org. Chem., 2013, 23(23), 5051–5063 CrossRef.
- J. K. Roy, S. Kar and J. Leszczynski, Revealing the Photophysical Mechanism of N,N′-Diphenyl-aniline Based Sensitizers with the D–D–π–A Framework: Theoretical Insights, ACS Sustainable Chem. Eng., 2020, 8(35), 13328–13341 CrossRef CAS.
- P. Dai, L. Yang, M. Liang, H. Dong, P. Wang, C. Zhang, Z. Sun and S. Xue, Influence of the terminal electron donor in D–D–π–A organic dye-sensitized solar cells: dithieno[3,2-b:2′,3′-d]pyrrole versus bis(amine), ACS Appl. Mater. Interfaces, 2015, 7(7), 22436–22447 CrossRef CAS.
- Z. Wan, C. Jia, Y. Duan, L. Zhou, Y. Lin and Y. Shi, Phenothiazine–triphenylamine based organic dyes containing various conjugated linkers for efficient dye-sensitized solar cells, J. Mater. Chem., 2012, 22(22), 25140–25147 RSC.
- R. G. Parr, Density-functional theory of atoms and molecule, Oxford University Press, 1989, pp. 2592–2598 Search PubMed.
- V. A. Rassolov, M. A. Ratner, J. A. Pople, P. C. Redfern and L. A. Curtiss, 6-31G* basis set for third-row atoms, J. Comput. Chem., 2001, 22(22), 976–984 CrossRef CAS.
- M. J. Frisch, G. W. Trucks, H. B. Schlegel, G. E. Scuseria, M. A. Robb, J. R. Cheeseman, G. Scalmani, V. Barone, B. Mennucci, G. A. Petersson, H. Nakatsuji, M. Caricato, X. Li, H. P. Hratchian, A. F. Izmaylov, J. Bloino, G. Zheng, J. L. Sonnenberg, M. Hada, M. Ehara, K. Toyota, R. Fukuda, J. Hasegawa, M. Ishida, T. Nakajima, Y. Honda, O. Kitao, H. Nakai, T. Vreven, J. A. Montgomery Jr, J. E. Peralta, F. Ogliaro, M. Bearpark, J. J. Heyd, E. Brothers, K. N. Kudin, V. N. Staroverov, R. Kobayashi, J. Normand, K. Raghavachari, A. Rendell, J. C. Burant, S. S. Iyengar, J. Tomasi, M. Cossi, N. Rega, J. M. Millam, M. Klene, J. E. Knox, J. B. Cross, V. Bakken, C. Adamo, J. Jaramillo, R. Gomperts, R. E. Stratmann, O. Yazyev, A. J. Austin, R. Cammi, C. Pomelli, J. W. Ochterski, R. L. Martin, K. Morokuma, V. G. Zakrzewski, G. A. Voth, P. Salvador, J. J. Dannenberg, S. Dapprich, A. D. Daniels, O. Farkas, J. B. Foresman, J. V. Ortiz, J. Cioslowski and D. J. Fox, Gaussian 09, Revision A.1, Gaussian Inc., Wallingford, CT, 2009 Search PubMed.
- V. Mohankumar, P. Pounraj, M. S. Pandian and P. Ramasamy, Theoretical Investigation on Flavones and Isoflavones-Added Triphenylamine-Based Sensitizers for DSSC Application, Braz. J. Phys., 2019, 49(1), 103–112 CrossRef CAS.
- S. Jungsuttiwong, R. Tarsang, T. Sudyoadsuk, V. Promarak, P. Khongpracha and S. Namuangruk, Theoretical study on novel double donor-based dyes used in high efficient dye-sensitized solar cells: the application of TDDFT study to the electron injection process, Org. Electron., 2013, 14(3), 711–722 CrossRef CAS.
- R. Venkatraman, S. V. K. Panneer, E. Varathan and V. Subramanian, Aromaticity–Photovoltaic Property Relationship of Triphenylamine-Based D–π–A Dyes: Leads from DFT Calculations, J. Phys. Chem. A, 2020, 124(17), 3374–3385 CrossRef CAS.
- S. L. Dudarev, G. A. Botton, S. Y. Savrasov, C. J. Humphreys and A. P. Sutton, Electron energy loss spectra and the structural stability of nickel oxide: an LSDA+U study, Phys. Rev. B: Condens. Matter Mater. Phys., 1998, 57(9), 1505–1509 CrossRef CAS.
- G. Kresse and J. Furthmtiller, Efficient iterative schemes for ab initio total-energy calculations using a plane-wave basis set, Phys. Rev. B: Condens. Matter Mater. Phys., 1996, 54(16), 11169–11186 CrossRef CAS.
- M. Lazzeri, A. Vittadini and A. Selloni, Erratum: Structure and energetics of stoichiometric TiO2 anatase surfaces, Phys. Rev. B: Condens. Matter Mater. Phys., 2002, 65, 119901 CrossRef.
- W.-L. Ding, D.-M. Wang, Z.-Y. Geng, X.-L. Zhao and Y.-F. Yan, Density functional theory characterization and verification of high-performance indoline dyes with D–A–π–A architecture for dye-sensitized solar cells, J. Phys. Chem. C, 2013, 117, 17382–17398 CrossRef CAS.
- K. Chaitanya, X.-H. Ju and B. M. Heron, Can elongation of the p-system in triarylamine derived sensitizers with either benzothiadiazole and/or ortho-fluorophenyl moieties enrich their light harvesting efficiency? – a theoretical study, RSC Adv., 2014, 4, 26621–26634 RSC.
- G. L. Zhang, Y. Bai, R. Z. Li, D. Shi, S. Wenger, S. M. Zakeeruddin, M. Gratzel and P. Wang, Employ a bisthienothiophene linker to construct an organic chromophore for efficient and stable dye-sensitized solar cells, Energy Environ. Sci., 2009, 2(1), 92–95 RSC.
Footnote |
† Electronic supplementary information (ESI) available: Optimized geometry structures of ME101–ME106, the frontier molecular orbitals of ME101–ME106 and density of states for ME101–ME106. See DOI: 10.1039/d0ra08815c |
|
This journal is © The Royal Society of Chemistry 2021 |