DOI:
10.1039/D0RA08325A
(Paper)
RSC Adv., 2021,
11, 543-554
Synthetic- and DFT modelling studies on regioselective modified Mannich reactions of hydroxy-KYNA derivatives†
Received
29th September 2020
, Accepted 6th December 2020
First published on 24th December 2020
Abstract
The syntheses of hydroxy-substituted kynurenic acid (KYNA) derivatives have been achieved by an optimised Conrad–Limpach procedure. The derivatives were then reacted with morpholine and paraformaldehyde, as a representative amine and aldehyde, in a modified Mannich reaction. The newly introduced substituents altered the preferred reaction centre of the KYNA skeleton. A systematic investigation of substitutions was carried out, using different reaction conditions, resulting in mono- or disubstituted derivatives. Product selectivity and regioselectivity were rationalised by DFT calculations disclosing HOMO distribution and NBO charges on the potential nucleophilic centres in the anion of the appropriate KYNA ester assumed to be active components towards the iminium ion intermediate.
Introduction
Kynurenic acid (KYNA) or 4-hydroxyquinoline-2-carboxylic acid is an endogenous substance, produced during the metabolism of tryptophan (TRP), via a pathway that is also responsible for the production of nicotinamide adenine dinucleotide (NAD+) and NAD phosphate (NADP+).1,2 This metabolism is involved in the generation of a variety of other compounds and also leads to the formation of L-kynurenine (L-KYN), which can be further metabolised in two separate ways. One of them constructs KYNA, while the other gives 3-hydroxykynurenine (3-OH-KYN) and quinolinic acid (QUIN), the latter being the precursor of NAD.3,4
KYNA is one of the few endogenous excitatory amino acid receptor blockers with a broad spectrum of antagonistic properties in supraphysiological concentrations. It is well established that KYNA has high affinity towards N-methyl-D-aspartate (NMDA) receptors. Moreover, it has recently been disclosed that KYNA shows an even higher affinity towards positive modulatory binding site at α-amino-3-hydroxy-5-methyl-4-isoxazolepropionic acid (AMPA) receptor.5
Recently there has been increasing interest in the synthesis and pharmacological studies of KYNA derivatives. This is due to its neuroprotective ability, as it can prevent neuronal loss following excitotoxic, ischemia-induced and infectious neuronal injuries.6,7 Several papers have been published on different alterations of the KYNA skeleton. Substitutions at positions 5–8 were achieved by starting from the corresponding aniline via the modified Conrad–Limpach method.8–10 The hydroxy group at position 4 was transformed to ether10–12 or amine functions,13 while the carboxyl group at position 2 was mostly modified into esters10–12 or amides.14–16
Based on the evaluations of previous KYNA amides, a tertiary nitrogen is needed for biological activity towards the central nervous system.17–20 Derivatives bearing such functional groups can be synthesised by various methods, such as carboxyl amidation14–16 or by the transformation of the 4-hydroxy group.13 The substitution of KYNA in the modified Mannich reaction (mMr) also emerges as a straightforward version of functionalisation. In this one-pot reaction KYNA, used as a CH acid, can be reacted with an array of amines and aldehydes giving the corresponding targeted aminoalkylated derivatives with the desired cationic centre.21
Our present aim was to carry out a systematic series of investigations with respect to the aminoalkylation of 5-, 6-, 7- and 8-hydroxykynurenic acid ethyl ester derivatives. According to our hypothesis, the newly introduced hydroxy groups may override the effect of the pre-existing 4-hydroxy function, allowing the substitution to take place on ring B. The experimental results of the systematic investigation were planned to be rationalised by comparative DFT modelling studies.
Results and discussion
Synthesis of 5-, 6- and 7-hydroxykynurenic acid derivatives
In order to compare the results of the present study with those of previous mMr investigations, the esters of hydroxykynurenic acids were selected as substrates for the reactions. Although the syntheses of the methyl esters of hydroxy derivatives are described in the literature,22,23 the ethyl esters were chosen for several reasons. By carrying out their mMr, they provide a better basis for comparison with previous derivatives. Furthermore, using diethyl acetylenedicarboxylate (DEAD), faster formation of the enamine intermediate was observed. The desired derivatives (2a–c) were synthesised with the Conrad–Limpach method applying the optimisations refined in previous investigations (Scheme 1).21
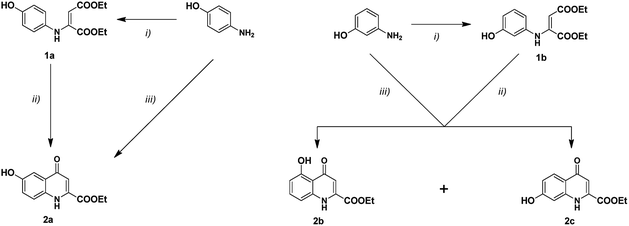 |
| Scheme 1 Synthesis of hydroxykynurenic acid derivatives; (i) DEAD, EtOH, reflux; (ii) DCB, 180 °C; 2a (55%), 2b (16%), 2c (39%); (iii) DEAD, p-TsOH, DCB, 190 °C, MW; 2a (62%), 2b (20%), 2c (43%). | |
As a last step, an additional column chromatographic purification of the esters was also required. The reason is that during the syntheses, even with the application of DEAD instead of the methyl derivative, maleimide side-products were formed in high yields. The literature describes the formation of these compounds as side-products during certain Conrad–Limpach reactions.24–26 However, in our case, probably due to the higher reactivity of hydroxyanilines, the syntheses were tilted toward the formation of the maleimides. After purification, the desired hydroxy esters were isolated in moderate yields. It is interesting to note that the synthesis starting from m-aminophenol allowed the isolation of the two possible regioisomers with a final ratio 1
:
2 (2b
:
2c).
Based on the work of Sutherland et al.,27 p-toluenesulfonic acid (p-TsOH) as catalyst was investigated in a one-pot version of the Conrad–Limpach procedure applying microwave irradiation (Scheme 1). The synthesis provided the appropriate hydroxy derivatives in increased yields with diminished maleimide formation and the work-up could be carried out without time-consuming chromatography.
6-Hydroxykynurenic acid ethyl ester
Based on preceding works, 1,4-dioxane seemed to be the optimal media for the substitution reactions. However, after achieving only moderate conversion (Table 1, entry 4) during the mMr of 2a (Scheme 2), the reaction was conducted in other solvents including acetonitrile (MeCN) as aprotic polar solvent, EtOH as protic polar solvent and toluene as aprotic apolar solvent at 80 °C, using 1 equivalent of morpholine and 3 equivalents of paraformaldehyde (Table 1).
Table 1 Screening of solvents in the case of 2a
Entry |
Amine equiv. |
Solvent |
T (°C) |
t |
Conv.a |
3a : 3ba |
Determined from crude NMR spectra. |
1 |
1.0 |
EtOH |
80 |
30′ |
10% |
3a |
2 |
1.0 |
EtOH |
80 |
1 h |
50% |
3a |
3 |
1.0 |
EtOH |
80 |
2 h |
85% |
4 : 1 |
4 |
1.0 |
1,4-Dioxane |
80 |
2 h |
70% |
1 : 1 |
5 |
1.0 |
Toluene |
80 |
2 h |
∼1% |
— |
6 |
1.0 |
MeCN |
80 |
2 h |
70% |
1 : 2 |
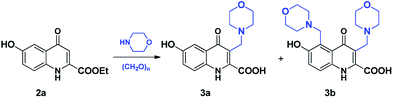 |
| Scheme 2 mMr of 2a using morpholine and paraformaldehyde. | |
We aimed to investigate the selectivity of the synthesis in ethanol, as this solvent enabled the formation of the most homogeneous reaction mixture presumably facilitating to achieve the highest yield. Although the higher temperature accelerated the reactions, conversions were maximised at around 85% (Table 2, entry 3 and 6). While higher temperature and longer reaction promoted the formation of 3b, its share in the isolated products remained low this showing high selectivity towards C-3 substitution.
Table 2 Screening of temperature and reagent equivalents in the case of 2a
Entry |
Amine equiv. |
Solvent |
T (°C) |
t |
Conv.a |
3a : 3ba |
Determined from crude NMR spectra. Work-up performed to isolate 3a. Work-up performed to isolate 3b. |
1 |
1.0 |
EtOH |
100 |
30′ |
45% |
4 : 1 |
2 |
1.0 |
EtOH |
100 |
1 h |
55% |
4 : 1 |
3 |
1.0 |
EtOH |
100 |
2 h |
85% |
7 : 2 |
4 |
1.0 |
EtOH |
150 |
30′ |
60% |
4 : 1 |
5 |
1.0 |
EtOH |
150 |
1 h |
65% |
7 : 2 |
6 |
1.0 |
EtOH |
150 |
2 h |
85% |
13 : 2 |
7 |
1.5 |
EtOH |
80 |
30′ |
60% |
4 : 1 |
8 |
1.5 |
EtOH |
80 |
1 h |
65% |
3 : 1 |
9 |
1.5 |
EtOH |
80 |
2 h |
90% |
2 : 1 |
10 |
2.0 |
EtOH |
80 |
30′ |
60% |
15 : 8 |
11 |
2.0 |
EtOH |
80 |
1 h |
70% |
10 : 9 |
12 |
2.0 |
EtOH |
80 |
2 h |
90% |
1 : 1 |
13b |
3.0 |
EtOH |
80 |
30′ |
99% |
3a |
14 |
3.0 |
EtOH |
80 |
1 h |
99% |
10 : 1 |
15c |
3.0 |
EtOH |
80 |
2 h |
99% |
1 : 1 |
To further investigate the reactivity of compound 2a, reactions using 2 and 3 equivalents of morpholine were carried out in EtOH. Faster reactions were observed in both cases, reaching a final ratio of 1
:
1 between 3a and 3b (Table 2, entry 12 and 15) further suggesting that the substitution at C-5 is much less preferred relative to that taking place at C-3.
It is worth mentioning that even though the reactions stopped after reaching a ratio of 1
:
1 after 2 hours, using increased amounts of amine halted the formation of 3b. This indicates a possible basic inhibition of the reaction preventing the formation of the reactive iminium ion. This view is in accord with the mechanism proposed for the Mannich-type condensation studied in this contribution. On the basis of solvent screening, the selectivity towards the formation of the disubstituted derivative can be increased by using aprotic solvents. Comparing the effect of MeCN to that of toluene and 1,4-dioxane suggests that an increased polarity might also increase this selectivity referring to the involvement of ionic species in the crucial regioselective coupling as discussed later (Table 3).
Table 3 Screening for solvent in the case of 2b
Entry |
Amine equiv. |
Solvent |
T (°C) |
t |
Conv.a |
4a : 4ba |
Determined from crude NMR spectra. |
1 |
1.0 |
EtOH |
80 |
30′ |
50% |
3 : 1 |
2 |
1.0 |
EtOH |
80 |
1 h |
70% |
2 : 1 |
3 |
1.0 |
EtOH |
80 |
2 h |
70% |
2 : 1 |
4 |
1.0 |
1,4-Dioxane |
80 |
2 h |
60% |
1 : 1 |
5 |
1.0 |
Toluene |
80 |
2 h |
55% |
5 : 3 |
6 |
1.0 |
MeCN |
80 |
2 h |
65% |
2 : 1 |
5-Hydroxykynurenic acid ethyl ester
On the basis of the results of the reactions performed with compound 2a, the first mMr starting from 2b was carried out in EtOH. As the reaction featured moderate conversion (Table 3, entry 3), the mMr was repeated using the other three solvents, showing lower conversion rates. Considering similarity in selectivity with 2a, a detailed investigation with EtOH as protic polar solvent was performed. During the reactions, C-6 aminoalkylated derivative 4a appeared to be the primary product with the C-3, C-6 disubstituted derivative formed only upon using prolonged reactions. It is interesting to note that in the case of 4a, the hydrolysis of the ester function did not take place, while in the case of compound 4b the free acid was isolated (Scheme 3).
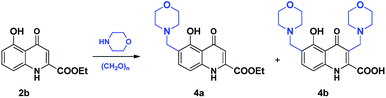 |
| Scheme 3 mMr of compound 2b using morpholine and paraformaldehyde. | |
The formation of 4a can be promoted by using higher temperature and shorter reaction, as a prolonged reaction led to the appearance of 4b, even using equivalent amounts of reagents. When using 3 equivalents of reagents, full conversion of 2b was achieved after 30 minutes with a 4a
:
4b distribution of 4
:
1 (Table 4, entry 13) that could be increased to 1
:
4 after 2 hours reaction time (Table 4, entry 15). Concluding the outcome of the reactions, 2b features higher reactivity at position C-6 than position C-3. However, similar to 2a, disubstitution of 2b can also be promoted by using longer reactions regardless of the polarity of the solvent.
Table 4 Screening of temperature and reagent equivalents in the case of 2b
Entry |
Amine equiv. |
Solvent |
T (°C) |
t |
Conv.a |
4a : 4ba |
Determined from crude NMR spectra. Work-up performed to isolate 4a. Work-up performed to isolate 4b. |
1 |
1.0 |
EtOH |
100 |
30′ |
40% |
4a |
2 |
1.0 |
EtOH |
100 |
1 h |
70% |
12 : 1 |
3 |
1.0 |
EtOH |
100 |
2 h |
70% |
6 : 1 |
4 |
1.0 |
EtOH |
150 |
30′ |
90% |
4a |
5b |
1.0 |
EtOH |
150 |
1 h |
95% |
4a |
6 |
1.0 |
EtOH |
150 |
2 h |
99% |
10 : 1 |
7 |
1.5 |
EtOH |
80 |
30′ |
40% |
10 : 3 |
8 |
1.5 |
EtOH |
80 |
1 h |
80% |
7 : 1 |
9 |
1.5 |
EtOH |
80 |
2 h |
90% |
2 : 1 |
10 |
2.0 |
EtOH |
80 |
30′ |
90% |
4 : 1 |
11 |
2.0 |
EtOH |
80 |
1 h |
95% |
2 : 1 |
12 |
2.0 |
EtOH |
80 |
2 h |
99% |
1 : 1 |
13 |
3.0 |
EtOH |
80 |
30′ |
99% |
4 : 1 |
14 |
3.0 |
EtOH |
80 |
1 h |
99% |
1 : 1 |
15c |
3.0 |
EtOH |
80 |
2 h |
99% |
1 : 4 |
7-Hydroxykynurenic acid ethyl ester
The modified Mannich reactions of 2c carried out in EtOH at 80 °C proved to be highly facile as indicated by the 80% conversion achieved even in 30 minutes (Table 5, entry 1). In this acidic phenol derivative, the reactivity of C-8 proved to be substantially higher than that of C-3 as indicated by the exclusive formation of 5a observed in all experiments using one equivalent of morpholine. It is of note that the hydrolysis of the ester function in 5a did not take place similar to that of 4a (Scheme 4).
Table 5 Screening of temperature and reagent equivalents in the case of 2c
Entry |
Amine equiv. |
Solvent |
T (°C) |
t |
Conv.a |
5a : 5ba |
Determined from crude NMR spectra. Work-up performed to isolate 5a. Multicomponent reaction, conversion could not be determined. Work-up performed to isolate 5b. |
1 |
1.0 |
EtOH |
80 |
30′ |
80% |
5a |
2 |
1.0 |
EtOH |
80 |
1 h |
95% |
5a |
3c |
1.0 |
EtOH |
80 |
1.5 h |
99% |
5a |
4 |
1.0 |
EtOH |
100 |
30′ |
75% |
5a |
5 |
1.0 |
EtOH |
100 |
1 h |
80% |
5a |
6 |
1.0 |
EtOH |
100 |
1.5 h |
90% |
5a |
7 |
1.0 |
EtOH |
150 |
30′ |
—b |
— |
10 |
1.5 |
EtOH |
80 |
30′ |
85% |
5a |
11 |
1.5 |
EtOH |
80 |
1 h |
95% |
5a |
12 |
1.5 |
EtOH |
80 |
1.5 h |
99% |
5a |
13 |
2.0 |
EtOH |
80 |
30′ |
85% |
5a |
14 |
2.0 |
EtOH |
80 |
1 h |
90% |
5a |
15 |
2.0 |
EtOH |
80 |
1.5 h |
99% |
18 : 1 |
16d |
2.0 |
EtOH |
80 |
56 h |
99% |
3 : 10 |
17 |
3.0 |
EtOH |
80 |
15′ |
99% |
5a |
18 |
3.0 |
EtOH |
80 |
1 h |
99% |
5a |
19 |
3.0 |
EtOH |
80 |
1.5 h |
99% |
18 : 1 |
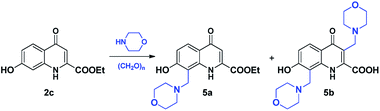 |
| Scheme 4 mMr of compound 2c using morpholine and paraformaldehyde. | |
During reactions carried out using higher equivalents of reagents, the formation of 5b was also observed. However, the formation of this product was slow, becoming detectable only after approximately 1.5–2 hours at 80 °C. As the formation of the products seemed to proceed under kinetic control, we attempted to perform a reaction using conventional heating for 56 hours that afforded 5b in sufficient amount (Table 5, entry 16).
As the formation of 5b was low even upon using higher temperature or increased amount of reagents, solvents tested previously were also investigated. In the case of 1,4-dioxane (as an aprotic solvent with moderate polarity), a selectivity towards the formation of disubstituted derivative 5b was observed (Table 6, entry 1), while in MeCN both 5a and 5b were formed with selectivity slightly tilted towards 5a (Table 6, entry 3).
Table 6 Screening for solvent in the case for 2c
Entry |
Amine equiv. |
Solvent |
T (°C) |
t |
Conv.a |
5a : 5ba |
determined from crude NMR spectra. |
1 |
1.0 |
1,4-Dioxane |
80 |
2 h |
45% |
1 : 5 |
2 |
1.0 |
Toulene |
80 |
2 h |
0% |
— |
3 |
1.0 |
MeCN |
80 |
2 h |
70% |
2 : 1 |
8-Hydroxykynurenic acid ethyl ester
Compound 8 was synthesised by the esterification of 8-hydroxykynurenic acid (xanthurenic acid, 6) as it was commercially available (Scheme 5). This step was needed to avoid the direct use of poorly soluble xanthurenic acid and to obtain information from the mMr comparable to that resulted from the experiments previously carried out with hydroxylated KYNA derivatives.
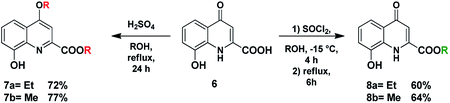 |
| Scheme 5 Esterification of xanthurenic acid (6). | |
It is interesting to mention that the esterification was first carried out according to a literature method used for the synthesis of the methyl ester.28 The use of EtOH as solvent led to the isolation of 4-ethoxy-substituted ethyl ester 7a. Since methanol was used for the synthesis described in the aforecited literature, the reaction was repeated in this solvent. In a similar manner, under these conditions, methoxy-substituted methyl ester 7b could be isolated. As suggested also by the literature, 7a and 7b should have been formed during the esterification by thionyl chloride in EtOH or MeOH.29 In our hands, however employing these conditions both esters (8a,b) could be obtained in good yields, without being contaminated by the corresponding 4-alkoxyquinoline.
Compound 9a has already been described in the literature, synthesised by employing benzyl protection of the 8-hydroxy function.30 As there was no further information about the reactivity of the 8-hydroxy derivative, we planned to explore the effect of the unprotected function on the course of the reaction (Scheme 6).
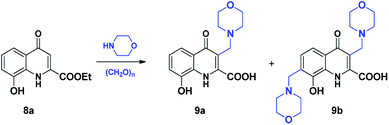 |
| Scheme 6 Synthesis of aminoalkylated xanthurenic acid derivatives. | |
Based on our experiences on the synthesis of the previously discussed hydroxy derivatives, aminoalkylations were first carried out in EtOH. All attempts at different temperatures yielded complex mixtures, but formation of 9a could not be detected. The reactions, conducted in the presence of increased equivalents of reagents, also provided complex mixtures, only traces of 9b could be detected in the crude product by 1H-NMR (Table 7).
Table 7 Screening of temperature and reagent equivalents in the case of 8a
Entry |
Amine equiv. |
Solvent |
T (°C) |
t |
Conv.a |
9a : 9ba |
Determined from crude NMR spectra. Conversion could not be determined. No traces of 9a or 9b was detected. Minimal amounts, could not be isolated. |
1 |
1.0 |
EtOH |
80 |
1 h |
—b |
—c |
2 |
1.0 |
EtOH |
100 |
1 h |
—b |
—c |
3 |
1.0 |
EtOH |
150 |
1 h |
—b |
—c |
4 |
1.5 |
EtOH |
80 |
1 h |
—b |
9bd |
5 |
2.0 |
EtOH |
80 |
1 h |
—b |
9bd |
6 |
3.0 |
EtOH |
80 |
1 h |
—b |
9bd |
After these unsuccessful experiments carried out in EtOH, solvents tested previously were again investigated. In toluene and MeCN the sole formation of 9b was observed, while in 1,4-dioxane the reaction yielded the C-3 substituted derivative (9a) as single product (Table 8).
Table 8 Screening for solvent in the case for 8a
Entry |
Amine equiv. |
Solvent |
T (°C) |
t |
Conv.a |
9a : 9ba |
Determined from crude NMR spectra. Work-up performed to isolate 9a. Work-up performed to isolate 9b. |
1 |
1.0 |
1,4-Dioxane |
80 |
2 h |
80% |
9a |
2 |
1.0 |
1,4-Dioxane |
100 |
2 h |
90% |
9a |
3b |
1.0 |
1,4-Dioxane |
150 |
2 h |
99% |
9a |
4 |
2.0 |
1,4-Dioxane |
150 |
2 h |
99% |
9a |
5c |
2.0 |
Toluene |
80 |
1 h |
99% |
9b |
6 |
2.0 |
MeCN |
80 |
2 h |
99% |
9b |
DFT calculations
In order to rationalise the marked regioselectivity patterns observed in the modified Mannich reactions of the systematic selection of kynurenic acid esters, we undertook a series of comparative DFT calculations carried out with B3LYP functional31–33 using 6–31 + G(d,p) basis set.34 The computations were supported by IEFPCM solvent model35 with dielectric constant ε = 24.5 to represent the polarity of ethanol employed as solvent in most experiments. However, the following characteristic features of the studied transformations seemed to be worth for primary consideration to create reliable models that will be analysed by theoretical methods: (i) no additional acidic component was added to the reaction mixtures; (ii) depending on the substitution pattern of the substrate, the Mannich-type coupling reactions might be accompanied by facile ester hydrolysis. Addressing the first point it can be assumed that the iminium ion and the amidate or phenolate anion derived from the condensation of morpholine, formaldehyde and the appropriate substrate of acidic character, are the actual coupling components involved in the Mannich reactions. It seems to be reasonable to assume that the formation of the major product can be interpreted by the coupling of iminium ion 10 to the most nucleophilic carbon centre of kynurenic anion derived from the deprotonation of the most acidic site of the neutral substrate. The possible sequences of the competitive Mannich condensations accompanied by the hydrolysis of the ester residue are exemplified by the conversions of 6-hydroxykynurenic acid ethyl ester 2a (Fig. 1). Since 2a/I, featuring the most pronounced nucleophilicity on C3, is more stable by 0.42 kcal mol−1 than 2a/II with preferred nucleophilic site at position 5, the dominant coupling of iminium ion 10 must lead to intermediate 11 capable of undergoing either equilibrium isomerisation to quinolone ester 12 or transannular ethanol elimination to generate quinoidal ketene intermediate 14. (The details of modelling studies disclosing relative energetics and local reactivity are discussed later.) Thus, carboxylic acid 3a, identified as major product, can be formed by the addition of water to 14 or by the neighbouring amine-assisted ester hydrolysis proceeding via spirocyclic zwitterionic intermediate 13. The regioselective iminium-mediated coupling of the alternative anion 2a/II is supposed to construct ester 15, which undergoes tautomerisation followed by transannular ethanol elimination (15 ↔ 16 ↔ 17). Subsequent hydration of the ketene intermediate might generate carboxylic acid 18 prone to undergo a further Mannich-type substitution affording minor product 3b. On the other hand, this polyfunctionalised kynurenic acid can also be derived from a subsequent coupling of 3a.
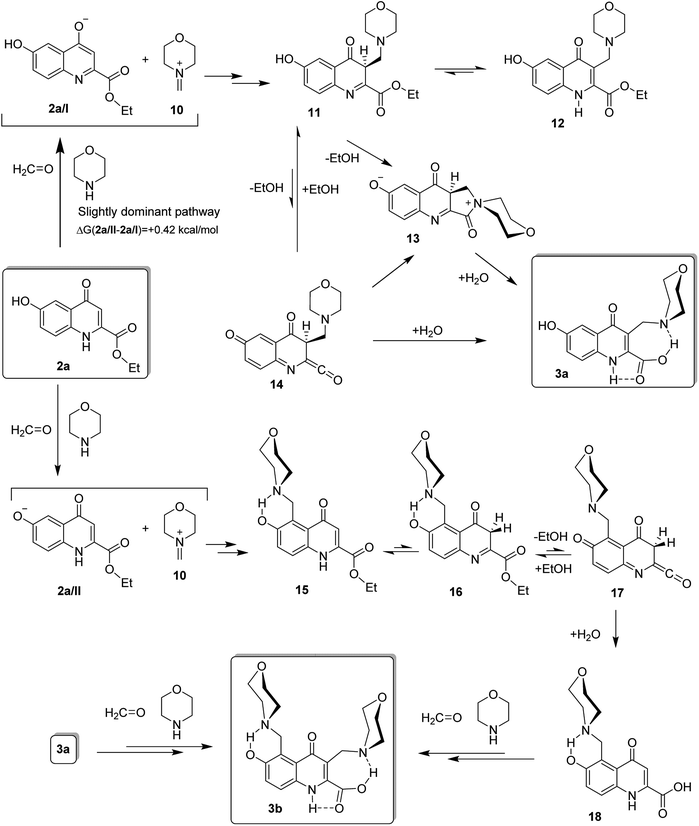 |
| Fig. 1 The possible sequences of the competitive Mannich condensations. | |
The mechanistic picture, accounting for the experimentally observed regioselectivity and ester hydrolysis, represented by the reaction pathways applied for the modified Mannich reactions of 2a, can be extended to analogous multistep transformations of the other kynurenic esters investigated in this work. However, searching for a reliable interpretation of the characteristic dependence of the regioselectivity on the substitution pattern, besides the relative thermodynamic stability, the HOMO delocalisation and the local NBO charges36 were also disclosed for the corresponding anion pairs type 2/I-2/II as outlined on Fig. 2. Since the crucial coupling between iminium ion 10 and the appropriate anion presumably takes places under simultaneous controls of orbital overlap and the electrostatic interaction between the ionic coupling partners, it can be established that – in excellent correlation with the structures of the major products of primary Mannich-type coupling – the most nucleophilic regions in the optimised structures of the more stable anions (framed structures) can be considered as reliably identified reactive sites on the basis of NBO charge and HOMO distribution, if they are taken together into account. It must also be noted here that the positions of the OH group on the fused benzene ring in 4a and 5a do not allow the ester hydrolysis taking place through the corresponding quinoidal ketene intermediates. However, regardless of the position of the OH group, the neighbouring group assistance from the 3-morpholinomethyl substituent obviously promotes this hydrolysis as discussed above.
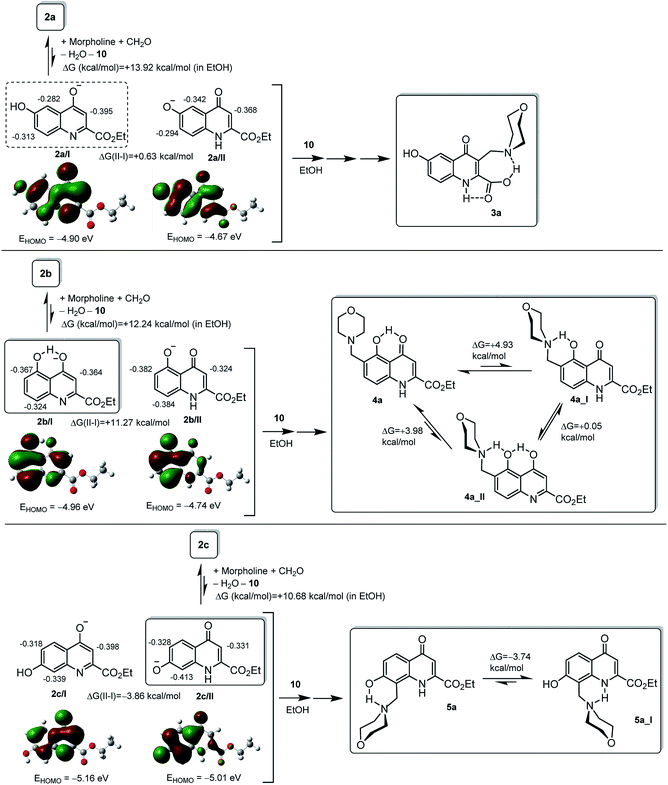 |
| Fig. 2 Rationalisation of the feasibility and regioselectivity observed in the Mannich reactions of 2a–c in terms of the relative thermodynamics, HOMO-energy, HOMO-delocalisation and the local NBO charges of the possible alternative anions generated by morpholine and formaldehyde along with the relative thermodynamics of the chelate-stabilized rotamer products. | |
Since it is reasonable to assume that besides the electronic properties of the active intermediates the overall tendency of isomers 2a–c to undergo Mannich-type reactions must also be strongly influenced by the concentration of the actual coupling partners, the changes in the free energy accompanying the generation of the appropriate ion pairs (types 2/I-10 and 2/II-10) were calculated by the DFT method used for the structural analysis of the anions (Fig. 2). In this regard, conversion 2c → 5a proceeding via 2c/II seems to be partly facilitated by the enhanced OH-acidity of the kynurenic precursor as reflected from the ΔG value calculated for ion-pair generation 2c + morpholine + CH2O → 2c/II+10 + H2O which is substantially smaller than those calculated for the other related processes studied in this work. It is of note that the relatively low HOMO energy level disclosed for anion 2c/II might suggest its decreased tendency to participate in orbital controlled interactions however, the significant electron density in position 8 (ρNBO = −0.413) refers to an increased coulombic contribution in promoting the coupling with iminium ion 10.
Comparative DFT calculations were also performed for two sets of alternative chelate-stabilized rotamers of Mannich products with the morpholinomethyl group attached on the fused benzene ring (4a/4a_I/4a_II and 5a/5a_I: Fig. 2) that identified 4a and 5a, respectively, as the preferred isomers in ethanolic solution.
The sluggish reactivity of 8a experienced in EtOH can be associated with the HOMO energy level of 8a/III found to be the lowest one in the series of the modelled nucleophilic anions and with the electron density in position 3 (ρNBO = −0.382) decreased relative to that identified in position 8 for anion 2c/II, the model with somewhat lowered HOMO energy level that presented an increased reactivity in EtOH (Fig. 3).
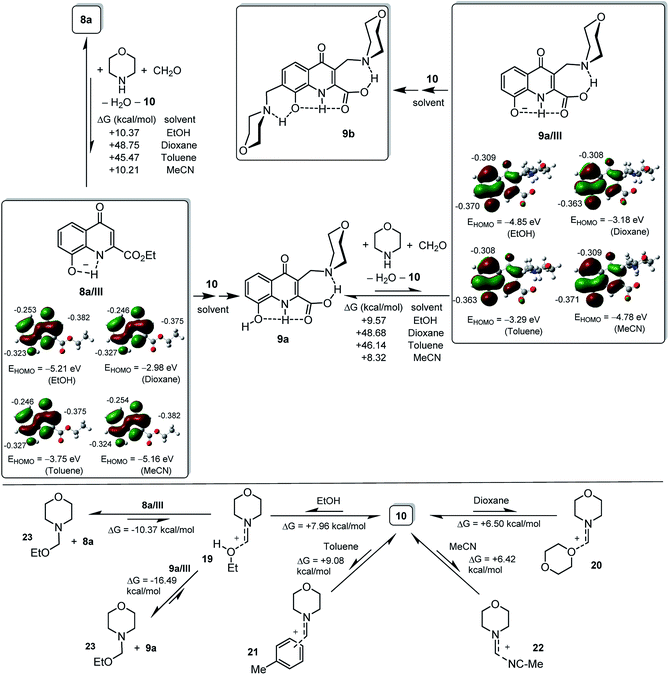 |
| Fig. 3 Rationalisation of the marked solvent effect observed for the regioselective sequential Mannich reactions of 8a in terms of: (i) the solvent-dependent changes in free energy accompanying the conversions leading active ion pairs 8a/III-10 and 9a/III-10, respectively; (ii) HOMO-energy of the anions; (iii) interactions of the iminium ion 10 with the solvent molecules. | |
Finally, we assume that the spectacular solvent–dependence observed in the transformations of 8a can be rationalized by the polarity-controlled feasibility of the ion pairs 8a/III-10 and 9a/III-10, the active coupling components of the first and second Mannich-like reactions, respectively. Accordingly, by means of a series of further B3LYP/6-31+G(d,p) calculations supported by IEFPCM method with the dielectric constants of the solvents used in the experiments, we assessed the changes in free energy associated with the primary ion-pair generating equilibrium condensation steps that involve 8a and 9a, respectively (Fig. 3). In the course of the modelling studies both the geometry optimisation and the subsequent frequency calculation were performed using IEFPCM solvent model. In accord with the general expectations the results (Fig. 3) indicate that in the relatively polar ethanol and acetonitrile the formation of reactive ion pairs 8a/III-10 and 9a/III-10 is substantially more favourable than in the much less polar dioxane and toluene. Although this view is in accord with the failure of the second coupling step of the sequential Mannich reactions attempted in dioxane affording 9a and with the ready formation of 9b via 9a in acetonitrile, the ΔG values calculated for the ion pair forming condensations taking place in ethanol and toluene apparently contradict to the experimental results referring to a definite inhibitory effect of the more polar solvent and to the facilitating effect of the less polar one. This apparent contradiction can partly be resolved by taking donor–acceptor interactions of iminium cation 10 with the solvent molecules into account (Fig. 3). Such interactions simply modelled by the involvement of one solvent molecule are presumably responsible for at least a partial deactivation of the electrophilic component, as reflected from the comparison of the ΔG values calculated for the formation of bimolecular complexes. Thus, among the solvents tested toluene seems to be the one with the most decreased capability to deactivate iminium ion 10 allowing a relatively facile Mannich-like couplings of the ion pairs. It is also noteworthy that in both anions the HOMO delocalisation and the local NBO charges are practically invariant to the polarity of the environment, while the HOMO energy level is substantially higher in the less polar solvents than in the more polar ones suggesting that these species, present even in low concentration in dioxane and toluene, display significant nucleophilicity enhanced relative to that predictable in ethanol and acetonitrile. Highlighting the significance of HOMO level influencing anion reactivity and consequent chemoselectivity, the HOMO energy calculated in dioxane for 8a_III is markedly higher compared to that disclosed for 9a_III (−2.98 eV and −3.18 eV, respectively: Fig. 3). In accord with the outcome of the reaction conducted in dioxane selectively affording 9a, this finding refers to a decreased nucleophilicity of 9a_III relative to that of 8a_III, while in toluene the reversed order of HOMO levels was disclosed for these anions (−3.75 eV and −3.29 eV for 8a_III and 9a_III, respectively) plausibly reasoning the facile formation of 9b when the reaction was carried out in this solvent. In acetonitrile the relatively high concentration of ion pairs 8a/III-10 and 9a/III-10 might be responsible for the facilitation of the sequential coupling steps finally constructing 9b, as the HOMO levels of anions calculated in this polar solvent are lower (−5.16 eV and −4.78 eV for 8a_III and 9a_III, respectively) referring to their decreased donor character. Finally, the highly decreased reactivity of the ion pairs in ethanol can also be attributed to the deactivation of anions 8a/III and 9a/III by hydrogen bonds with the solvent molecules and to the favoured formation of 23 (Fig. 3).
Conclusions
Through an optimised Conrad–Limpach procedure, the syntheses of hydroxy-substituted kynurenic acid derivatives were achieved. In order to investigate the substitution affecting effect of the new hydroxy groups in modified Mannich reaction, a systematic investigation was carried out on the synthesised derivatives. Hydroxykynurenic acids were reacted with morpholine and paraformaldehyde – as representative amine and aldehyde – under different reaction conditions. These reactions resulted in varied substitution positions.
Employing 5-, 6- and 7-hydroxy derivatives as substrates, higher conversions and, consequently, higher yields could be achieved in EtOH. It is hypothesised that this protic, polar solvent can contribute to higher intermediate stability by solvating the iminium ion formed during the reaction. Higher temperatures allowed higher conversions, while giving appearance to disubstituted derivatives. Decreasing the solvent polarity resulted in increased amounts of disubstituted derivatives. This may be attributed to a decreased stability and enhanced reactivity of the iminium ion capable of aminoalkylating the less reactive positions as well. In the case of 8-hydroxykynurenic acid, comparative DFT calculations suggested that the overall low reactivity might be due to the low energy level of HOMO in the chelate-stabilised anion.
By means of DFT calculations it was also disclosed that the regioselectivity of the modified Mannich reactions of hydroxykynurenic acids can reasonably be rationalised on the basis of the relative acidity of the potential nucleophilic sites as well as the HOMO delocalisation and the local NBO charges in the resulting anions. The spectacular solvent-dependent reactivity of 8-hydroxykynureic acid ester was also rationalised by a series of comparative DFT calculations revealing the relative thermodynamics of a polarity-controlled formation of the ion pair involved in the crucial C_C coupling, the iminium-deactivation by the interaction with the solvent used and the polarity-dependent HOMO energy level of the anion component.
Experimental
General
The 1H and 13C-NMR spectra were recorded in DMSO d6, CDCl3 and D2O solutions in 5 mm tubes at room temperature (RT), on a Bruker DRX-500 spectrometer (Bruker Biospin, Karlsruhe, Baden Württemberg, Germany) at 500 (1H) and 125 (13C) MHz, with the deuterium signal of the solvent as the lock and TMS as internal standard (1H, 13C).
The HRMS flow injection analysis was performed with Thermo Scientific Q Exactive Plus hybrid quadrupole-Orbitrap (Thermo Fisher Scientific, Waltham, MA, USA) mass spectrometer coupled to a Waters Acquity I-Class UPLC™ (Waters, Manchester, UK).
Melting points were determined on a Hinotek X-4 melting point apparatus. Merck Kieselgel 60F254 plates were used for TLC.
All calculations were carried out by using the Gaussian 09 software (Gaussian Incorporation, Pittsburgh, U.S.) package.37 The optimised structures are available from the authors.
General procedure for the synthesis of aminoalkylated 6-hydroxykynurenic acid derivatives (3a,b)
Ethyl 6-hydroxy-4-oxo-1,4-dihydroquinoline-2-carboxylate (2a, 117 mg, 0.5 mmol), morpholine (131 mg, 1.5 mmol) and paraformaldehyde (70 mg, 2.3 mmol) were placed in a pressure-resistant 10 mL vessel with EtOH (5 mL). The mixture was kept at 80 °C for the corresponding reaction time in a microwave reactor (300 W). Following the removal of the solvent, the residue was crystallised as detailed below.
6-Hydroxy-3-(morpholinomethyl)-4-oxo-1,4-dihydroquinoline-2-carboxylic acid (3a). Reaction time in microwave reactor: 30 min. Crystallisation from DCM (5 mL). Yield: 123 mg (81%); M.p. 220–225 °C. 1H NMR (DMSO-d6); 2.99–3.15 (2H, m); 3.16–3.27 (2H, m); 3.53–3.74 (2H, m); 3.81–4.04 (2H, m); 4.38 (2H, s); 7.17 (1H, d, J = Hz); 7.41 (1H, s); 7.87 (1H, d, J = Hz); 9.74 (1H, brs); 11.70 (1H, brs); 12.87 (1H, brs); 13C NMR (DMSO-d6); 49.8; 50.8; 64.3; 106.4; 107.8; 121.6; 123.0; 126.0; 132.9; 134.4; 147.0; 154.7; 165.0; 176.8; HRMS calcd for [M + H+] m/z = 305.1132, found m/z = 305.1138.
6-Hydroxy-3,5-bis(morpholinomethyl)-4-oxo-1,4-dihydroquinoline-2-carboxylic acid (3b). Reaction time in microwave reactor: 2 h. Crystallisation from DCM (5 mL) – removal of 3a; evaporation of DCM from the mother liquor, crystallisation from EtOAc (5 mL). Yield: 59 mg (39%); M.p. >325 °C. 1H NMR (DMSO-d6); 2.50–2.58 (4H, m); 3.09–3.19 (4H, m); 3.59–3.66 (4H, m); 3.68–3.87 (4H, m); 4.34 (2H, s); 4.77 (2H, m); 7.10 (1H, d, J = 8.4 Hz); 7.85 (1H, d, J = 8.9 Hz); 13C NMR (DMSO-d6); 49.9; 50.9; 52.9; 64.3; 66.4; 108.0; 120.8; 123.0; 123.8; 134.4; 145.9; 155.6; 164.8; 179.3; HRMS calcd for [M + H+] m/z = 404.1816, found m/z = 404.1810.
Ethyl 5-hydroxy-3-(morpholinomethyl)-4-oxo-1,4-dihydroquinoline-2-carboxylate (4a)
Ethyl 5-hydroxy-4-oxo-1,4-dihydroquinoline-2-carboxylate (2b, 117 mg, 0.5 mmol), morpholine (48 mg, 0.5 mmol) and paraformaldehyde (45 mg, 1.5 mmol) were placed in a pressure-resistant vessel of 10 mL with 5 mL EtOH. The mixture was kept at 150 °C for 1 h in a microwave reactor. Following the removal of the solvent, the residue was crystallised from EtOAc (5 mL). Yield: 132 mg (80%); M.p. 160–164 °C. 1H NMR (DMSO-d6); 1.37 (3H, t, J = 7.4 Hz); 2.37–2.47 (4H, m); 3.48–3.61 (6H, m); 4.44 (2H, q, J = 7.1 Hz); 6.65 (1H, s); 7.35 (1H, d, J = 8.7 Hz); 7.62 (1H, d, J = 8.6 Hz); 12.46 (1H, brs); 14.45 (1H, brs); 13C NMR (DMSO-d6); 14.3; 53.5; 54.9; 108.1; 108.9; 114.0; 136.6; 139.5; 161.9; 183.3; HRMS calcd for [M + H+] m/z = 333.1445, found m/z = 333.1444.
5-Hydroxy-3,6-bis(morpholinomethyl)-4-oxo-1,4-dihydroquinoline-2-carboxylic acid (4b)
Ethyl 5-hydroxy-4-oxo-1,4-dihydroquinoline-2-carboxylate (2b, 117 mg, 0.5 mmol), morpholine (131 mg, 1.5 mmol) and paraformaldehyde (70 mg, 2.3 mmol) were placed in a pressure-resistant vessel of 10 mL with 5 mL EtOH. The mixture was kept at 80 °C for 2 h in a microwave reactor. Following the removal of the solvent, the residue was crystallised from EtOAc (5 mL) and was recrystallised from EtOAc (10 mL). Yield: 137 mg (68%); M.p. 218–220 °C. 1H NMR (DMSO-d6); 2.40 (4H, s); 3.18 (2H, m); 3.50 (2H, s); 3.56 (4H, m); 3.59–4.05 (4H, m); 4.37 (2H, s); 7.37 (1H, d, J = 8.7 Hz); 7.55 (1H, d, J = 8.5 Hz); 12.16 (1H, brs); 12.48 (1H, brs); 14.62 (1H, brs); 13C NMR (DMSO-d6); 49.7; 50.1; 53.6; 55.1; 64.3; 65.8; 66.7; 107.9; 108.0; 108.1; 112.1; 122.5; 136.1; 143.8; 149.8; 163.9; 182.2; HRMS calcd for [M + H+] m/z = 404.1816, found m/z = 404.1817.
Ethyl 7-hydroxy-8-(morpholinomethyl)-4-oxo-1,4-dihydroquinoline-2-carboxylate (5a)
Ethyl 7-hydroxy-4-oxo-1,4-dihydroquinoline-2-carboxylate (2c, 117 mg, 0.5 mmol), morpholine (48 mg, 0.5 mmol) and paraformaldehyde (45 mg 1.5 mmol) were placed in a pressure-resistant vessel of 10 mL with 5 mL EtOH. The mixture was kept at 80 °C for 1.5 h in a microwave reactor. Following the removal of the solvent, the residue was crystallised from EtOH (5 mL). Yield: 144 mg (87%); M.p. 228–231 °C. 1H NMR (DMSO-d6); 1.37 (3H, t, J = 7.2 Hz); 2.45–2.50 (4H, m); 3.65–3.75 (4H, m); 3.94 (2H, s); 4.45 (2H, q, J = 7.1 Hz); 6.55 (1H, s); 6.95 (1H, d, J = 8.5 Hz); 7.88 (1H, d, J = 8.8 Hz); 10.55 (1H, brs); 13.13 (1H, brs); 13C NMR (DMSO-d6); 14.9; 53.6; 54.0; 63.5; 66.8; 107.8; 110.5; 115.1; 120.7; 126.2; 137.7; 142.4; 159.8; 163.3; 178.1; HRMS calcd for [M + H+] m/z = 333.1445, found m/z = 333.1445.
7-Hydroxy-3,8-bis(morpholinomethyl)-4-oxo-1,4-dihydroquinoline-2-carboxylic acid (5b)
Ethyl 7-hydroxy-4-oxo-1,4-dihydroquinoline-2-carboxylate (2c, 233 mg, 1.0 mmol), morpholine (174 mg, 2.0 mmol) and paraformaldehyde (120 mg, 4.0 mmol) were placed in a 50 mL round-bottom flask with 20 mL EtOH. The mixture was let stir for 56 h at reflux temperature using conventional heating. Following the evaporation of the solvent, 5a was removed by crystallizing the residue from EtOH (10 mL). The solvent from the mother liquor was evaporated and the residue was crystallised from EtOAc (10 mL) yielding 5b. Yield: 242 mg (60%); M.p. 239–241 °C. 1H NMR (DMSO-d6); 2.40–2.48 (4H, m); 2.97–3.11 (2H, m); 3.15–3.25 (2H, m); 3.55–3.63 (2H, m); 3.71 (4H, t, J = 4.5 Hz); 3.87 (2H, s); 3.90–4.03 (2H, m); 4.39 (2H, s); 6.91 (1H, d, J = 8.8 Hz); 7.88 (1H, d, J = 8.8 Hz); 10.39 (1H, brs); 12.89 (1H, brs); 13.13 (1H, brs); 13C NMR (DMSO-d6); 50.0; 50.3; 53.4; 53.4; 64.3; 66.1; 107.7; 108.0; 114.4; 118.4; 126.2; 140.6; 146.4; 159.2; 164.8; 177.3; HRMS calcd for [M + H+] m/z = 404.1816, found m/z = 404.1812.
General procedure for the synthesis of methyl and ethyl esters of 4-alkoxy substituted xanthurenic acid derivatives (7a,b)
Xanthurenic acid (6, 1 g, 4.9 mmol) was put in a 100 mL round bottom flask with 40 mL MeOH or EtOH and 1 mL cm3 H2SO4. The reaction mixture was treated at reflux temperature for 24 h. The H2SO4 was neutralised using NaHCO3 (using litmus paper as indicator). After the evaporation of the solvent, the mixture was dissolved in H2O (30 mL) and was extracted with DCM (3 × 25 mL). The collected organic phases were dried (Na2SO4), the solvent was evaporated and the residue was crystallised from n-hexane
:
EtOAc (1
:
9) mixture (15 mL).
Ethyl 4-ethoxy-8-hydroxyquinoline-2-carboxylate (7a). Solvent: EtOH. Yield: 685 mg (60%); M.p. 144–147 °C. 1H NMR (DMSO-d6); 1.11 (3H, t, J = 7.1 Hz); 1.38 (3H, t, J = 7.2 Hz); 3.75 (2H, q, J = 7.1 Hz); 4.43 (2H, q, J = 7.1 Hz); 6.81 (1H, s); 7.20 (1H, d, J = 7.7 Hz); 7.30 (1H, t, J = 8.0 Hz); 7.57 (1H, d, J = 8.3 Hz); 13C NMR (DMSO-d6); 14.8; 63.7; 109.1; 114.9; 116.4; 126.2; 126.7; 131.8; 139.8; 140.7; 162.9; 175.5.
Methyl 8-hydroxy-4-methoxyquinoline-2-carboxylate (7b). Solvent: MeOH. Yield: 687 mg (64%); M.p. 275–277 °C. 1H NMR (DMSO-d6); 3.96 (3H, s); 4.11 (1H, s); 7.17 (1H, d, J = 7.7 Hz); 7.50 (1H, t, J = 8.2 Hz); 7.53 (1H, s); 7.59 (1H, d, J = 8.4 Hz); 9.73 (1H, brs); 13C NMR (DMSO-d6); 53.1; 56.9; 101.0; 111.8; 113.3; 123.0; 129.2; 139.3; 147.5; 148.2; 154.5; 163.2.
8-Hydroxy-3-(morpholinomethyl)-4-oxo-1,4-dihydroquinoline-2-carboxylic acid (9a)
Ethyl 8-hydroxy-4-oxo-1,4-dihydroquinoline-2-carboxylate (8a, 117 mg, 0.5 mmol), morpholine (48 mg, 0.5 mmol) and paraformaldehyde (45 mg, 1.5 mmol) were placed in a pressure-resistant vessel of 10 mL with 5 mL 1,4-dioxane. The mixture was kept at 150 °C for 2 h in a microwave reactor. Following the removal of the solvent, the residue was crystallised from EtOAc (5 mL). Yield: 53 mg (35%); M.p. 290–295 °C (decomposition). 1H NMR (DMSO-d6); 3.03–3.23 (2H, m); 3.25–3.43 (2H, m); 3.54–3.74 (2H, m); 3.85–4.05 (2H, m); 4.51 (2H, s); 7.12 (1H, d, J = 7.5 Hz); 7.19 (1H, t, J = 8.0 Hz); 7.55 (1H, d, J = 8.2 Hz); 10.27 (1H, brs); 12.13 (1H, brs); 13C NMR (DMSO-d6); 50.3; 50.6; 64.1; 108.9; 115.6; 115.7; 124.6; 125.5; 128.9; 145.0; 147.0; 163.9; 178.0; HRMS calcd for [M + H+] m/z = 305.1132, found m/z = 305.1139.
8-Hydroxy-3,7-bis(morpholinomethyl)-4-oxo-1,4-dihydroquinoline-2-carboxylic acid (9b)
Ethyl 8-hydroxy-4-oxo-1,4-dihydroquinoline-2-carboxylate (8a, 117 mg, 0.5 mmol), morpholine (87 mg, 1.0 mmol) and paraformaldehyde (60 mg, 2.0 mmol) were placed in a pressure-resistant vessel of 10 mL with 5 mL toluene. The mixture was kept at 80 °C for 1 h in a microwave reactor. Following the removal of the solvent, the residue was crystallised from toluene (5 mL). Yield: 168 mg (83%); M.p. 218–224 °C. 1H NMR (DMSO-d6); 2.53–2.58 (4H, m); 3.03–3.20 (2H, m); 3.20–3.37 (2H, m); 3.64–3.69 (4H, m); 3.65–3.75 (2H, m) 3.89 (2H, s); 3.91–4.01 (2H, m); 4.50 (2H, s); 7.10 (1H, d, J = 8.5 Hz); 7.52 (1H, d, J = 8.5 Hz); 10.25 (1H, brs); 12.08 (1H, brs); 13C NMR (DMSO-d6); 24.0; 50.3; 50.5; 52.9; 60.0; 64.1; 66.4; 108.9; 115.3; 115.4; 124.4; 124.9; 145.0; 146.5; 159.6; 163.9; 177.9; HRMS calcd for [M + H+] m/z = 404.1816, found m/z = 404.1817.
Conflicts of interest
The authors declare no conflict of interest. The funders had no role in the design of the study; in the collection, analyses, or interpretation of data, in the writing of the manuscript, or in the decision to publish the results.
Acknowledgements
The authors' thanks are due to the Hungarian Research Foundation (OTKA No. K-129037); the Ministry of National Economy, National Research Development and Innovation Office [GINOP-2.3.2-15-2016-00034]; the EU-funded Hungarian Grant [EFOP-3.6.1-16-2016-00008]; the Ministry of Human Capacities, Hungary grant, TKP-2020; ÚNKP-20-3-SZTE-319 - New National Excellence Program of the Ministry for Innovation and Technology from the source of the National Research, Development and Innovation Fund and to the Gedeon Richter Plc. Centenarial Foundation.
Notes and references
- É. Rózsa, H. Robotka, L. Vécsei and J. Toldi, J. Neural Transm., 2008, 115, 1087–1091 CrossRef.
- T. W. Stone, Expert Opin. Invest. Drugs, 2001, 10, 633–645 CrossRef CAS.
- H. Németh, J. Toldi and L. Vécsei, Curr. Neurovasc. Res., 2005, 2, 249–260 CrossRef.
- H. Németh, J. Toldi and L. Vécsei, J. Neural Transm., Suppl., 2006, 70, 285–304 Search PubMed.
- K. Sas, H. Robotka, J. Toldi and L. Vécsei, J. Neurol. Sci., 2007, 257, 221–239 CrossRef CAS.
- G. Gigler, G. Szénási, A. Simó, G. Lévay, L. G. Hársing Jr, K. Sas, L. Vécsei and J. Toldi, Eur. J. Pharmacol., 2007, 564, 116–122 CrossRef CAS.
- E. Luchowska, P. Luchowski, A. Sarnowska, M. Wielosz, W. A. Turski and E. M. Urbańska, Pol. J. Pharmacol., 2003, 55, 443–447 CrossRef CAS.
- B. L. Harrison, B. M. Baron, D. M. Cousino and I. A. McDonald, J. Med. Chem., 1990, 12, 3130–3132 CrossRef.
- D. Edmont, R. Rocher, C. Plisson and J. Chenault, Bioorg. Med. Chem. Lett., 2000, 16, 1831–1834 CrossRef.
- F. P. Bonina, L. Arenare, R. Ippolito, G. Boatto, G. Battaglia, V. Bruno and P. de Caprariis, Int. J. Pharm., 2000, 202, 79–88 CrossRef CAS.
- S. Manfredini, B. Pavan, S. Vertuani, M. Scaglianti, D. Compagnone, C. Biondi, A. Scatturin, S. Tanganelli, L. Ferraro, P. Prasad and A. Dalpiaz, J. Med. Chem., 2002, 45, 559–562 CrossRef CAS.
- S. Manfredini, S. Vertuani, B. Pavan, F. Vitali, M. Scaglianti, F. Bortolotti, C. Biondi, A. Scatturin, P. Prasad and A. Dalpiaz, Bioorg. Med. Chem., 2004, 12, 5453–5463 CrossRef CAS.
- A. C. Nichols and K. L. Yielding, Mol. Chem. Neuropathol., 1998, 35, 1–12 CrossRef CAS.
- A. Brik, Y. C. Lin, J. Elder and C. H. Wong, Chem. Biol., 2002, 9, 891–896 CrossRef CAS.
- E. Knyihár-Csillik, A. Mihály, B. Krisztin-Péva, H. Robotka, I. Szatmári, F. Fülöp, J. Toldi, B. Csillik and L. Vécsei, Neurosci. Res., 2008, 61, 429–432 CrossRef.
- F. Fülöp, I. Szatmári, E. Vámos, D. Zádori, J. Toldi and L. Vécsei, Curr. Med. Chem., 2009, 16, 4828–4842 CrossRef.
- D. Zádori, G. Nyíri, A. Szőnyi, I. Szatmári, F. Fülöp, J. Toldi, T. F. Freund, L. Vécsei and P. Klivényi, J. Neural Transm., 2011, 118, 865–875 CrossRef.
- D. Zádori, G. Veres, L. Szalárdy, P. Klivényi, J. Toldi and L. Vécsei, J. Alzheimer's Dis., 2014, 42, S177–S187 Search PubMed.
- R. Greco, C. Demartini, A. M. Zanaboni, E. Redavide, S. Pampalone, J. Toldi, F. Fülöp, F. Blandini, G. Nappi, G. Sandrini, L. Vécsei and C. Tassorelli, Cephalalgia, 2017, 37, 1272–1284 CrossRef.
- A. Fejes-Szabó, Z. Bohár, E. Vámos, G. Nagy-Grócz, L. Tar, G. Veres, D. Zádori, M. Szentirmai, J. Tajti, I. Szatmári, F. Fülöp, J. Toldi, A. Paardutz and L. Vecsei, J. Neural. Transm., 2014, 121, 725–738 CrossRef.
- B. Lőrinczi, A. Csámpai, F. Fülöp and I. Szatmári, Molecules, 2020, 25, 937 CrossRef.
- M. C. Hall, G. H. Johnson and B. J. Wright, J. Med. Chem., 1974, 17, 685–690 CrossRef.
- J. A. Bryant, B. O. Buckman, I. Islam, R. Mohan, M. M. Morrissey, G. P. Wei, X. Wei and S. Yuan, US Pat., 16374202A, 2002 Search PubMed.
- N. D. Heindel, J. Org. Chem., 1970, 35, 3138–3140 CrossRef CAS.
- K. Kizaki, H. Imoto, T. Kato and K. Naka, Tetrahedron, 2015, 71, 643–647 CrossRef CAS.
- M. Boominathan, V. Sathish, M. Nagaraj, N. Bhuvanesh, S. Muthusubramanian and S. Rajagopal, RSC Adv., 2013, 3, 22246–22252 RSC.
- N. L. Sloan, S. K. Luthra, G. McRobbie, S. L. Pimlott and A. Sutherland, RSC Adv., 2017, 7, 54881–54891 RSC.
- B. H. Aktas, M. Chorev, J. A. Halperin and G. Wagner, US Pat., 2011042139W, 2011 Search PubMed.
- H. Kikuchi, T. Suzuki, M. Ogura, M. K. Homma, Y. Homma and Y. Oshima, Bioorg. Med. Chem., 2015, 23, 66–72 CrossRef CAS.
- M. Abarghaz, J.-J. Bourguignon, E. Klotz, J.-P. Macher, G. Ronsin, M. Schmitt and P. Wagner, US Pat., 49940305A, 2005 Search PubMed.
- A. D. Becke, J. Chem. Phys., 1998, 98, 5648–5652 CrossRef.
- C. Lee, W. Yang and R. G. Parr, Phys. Rev. B: Condens. Matter Mater. Phys., 1988, 37, 785–789 CrossRef CAS.
- P. J. Stephens, F. J. Devlin, C. F. Chahalowsky and M. J. Frisch, J. Phys. Chem., 1994, 98, 11623–11627 CrossRef CAS.
- J. W. Hehre, L. Radom, P. V. R. Schleyer and J. A. Pople, Ab initio molecular orbital theory, Wiley, New York, NY, USA, 1986 Search PubMed.
- J. Tomasi, B. Mennucci and É. Cancés, J. Mol. Struct., 1999, 464, 211–216 CrossRef CAS.
- F. Weinhold and C. R. Landis, Valency and Bonding: A Natural Bond Orbital Donor-Acceptor Perspective, Cambridge University Press, 2005 Search PubMed.
- Gaussian 09, Revision A.02, ed. M. J. Frisch, G. W. Trucks, H. B. Schlegel, G. E. Scuseria, M. A. Robb, J. R. Cheeseman, G. Scalmani, V. Barone, G. A. Petersson and H. Nakatsuji, et al., Gaussian, Inc., Wallingford, CT, USA, 2016 Search PubMed.
Footnote |
† Electronic supplementary information (ESI) available. See DOI: 10.1039/d0ra08325a |
|
This journal is © The Royal Society of Chemistry 2021 |