DOI:
10.1039/D0RA07458F
(Paper)
RSC Adv., 2021,
11, 8963-8969
A highly sensitive and selective on–off fluorescent sensor based on a complex of polySchiff-Fe2+ for Cr(VI) detection in an aqueous medium†
Received
30th August 2020
, Accepted 14th February 2021
First published on 1st March 2021
Abstract
Designing exceptional probes to detect minute quantities of chromium(VI) is of huge importance for the safety and health of the human race. In this study, a green fluorescence emission probe (polyethylene Schiff base-chelated Fe2+ complex) was synthesized by a one-pot synthesis method for the highly selective and sensitive detection of Cr(VI) in an aqueous solution. The complex of polyethylene Schiff-Fe2+ was fully characterized, and it displayed satisfactory stability in the aqueous solution. The fluorescence emission could be quenched specifically by the introduction of Cr(VI) via the oxidation of the Fe2+-centered polyethylene Schiff base complex. The fluorescence intensity decreases linearly with the concentration of Cr(VI), and the corresponding detection limit was calculated to be 0.18 μM. Thus, the obtained fluorescence detection system could be used for Cr(VI) detection in tap water. These features provide potential uses of the as-prepared polyethylene Schiff-Fe2+ complex as a sensor for environmental applications.
Introduction
Chromium mainly exists in the form of trivalent chromium (Cr(III)) and hexavalent chromium (Cr(VI)) in nature.1,2 Cr(III) is an essential trace element for human body to assist the normal metabolism of protein; however, Cr(VI) is highly toxic and is a pollutant in nature.3,4 The state stipulates that the content of Cr(VI) in sewage and drinking water shall not exceed 1.5 mg L−1 and 0.05 mg L−1, respectively. Excess Cr(VI) will not only inhibit the plant growth, leading to stunting,5,6 but also denatures the protein or enzyme in the human body after internalization, which may further increase the risk of cancer.7,8 Therefore, the monitoring of Cr(VI) content is of great significance.
The importance of Cr(VI) determination has promoted the prosperous development of strategies in the past several decades. Representatively, the diphenylcarbazide spectrophotometry-based national standard method for Cr(VI) detection has been used for over 30 years in China. This method displayed satisfactory detection limits (0.004 mg L−1) with colorimetric responses.9,10 To date, various analytical methods, including electrochemical assays,11,12 chromatography13 and atomic absorption spectrometry,14 have been successfully developed for the determination of Cr(VI). For these methods, disadvantages such as expensive cost, time-consuming, and complicated pretreatment processes limit their practical applications. In recent years, fluorescent probes for Cr(VI) detection have attracted considerable attention because of their potential features including high sensitivity, convenience and accessible instrument requirement.15,16 Up to now, the reported fluorimetric methods are mainly based on organic chromophores, carbon quantum dots and metal nanoclusters.17–20 For these fluorophores, however, their photostability is the key point that interferes with the quantificational evaluation of Cr(VI).21,22 New fluorometric methods to fulfill the long-term target analysis is urgently needed. Schiff base compounds containing an imine group can easily bond with metal ions to form complexes supported by the lone pair electrons of N.23–25 In our previous study, we found that the Schiff base synthesized with polymine (PEI) and 2.4-dihydroxybenzaldehyde (DHBFA) had weak fluorescence, which was enhanced when complexed with ferric divalent cation. Therefore, considering the strong oxidizing capacity of Cr(VI), we synthesized a new polyethylene Schiff base (PS)-chelated Fe2+ complex via an efficient microwave method. The six-membered Fe2+-based complex displayed strong and stabilized fluorescence emission at 505 nm, which could be quenched specifically by the introduction of Cr(VI) in an aqueous solution through the oxidation process. The on–off–on sensing property of PS-Fe2+ by adding Cr(VI) was designed (Fig. 1). The corresponding fluorescence intensity decreases linearly with the concentration of Cr(VI), which realizes the Cr(VI) detection quantificationally. Besides, the preparation of the polyethylene Schiff base-chelated Fe2+ complex (PS-Fe2+) was evaluated thoroughly, which presented an effective one-pot methodology to synthesis the new Cr(VI) fluorescent probe.
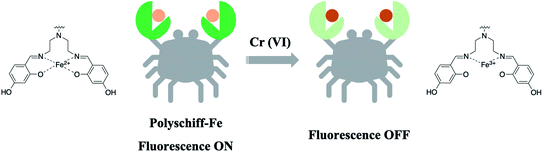 |
| Fig. 1 Schematic of the reaction of the PS-Fe2+ complex with Cr(VI). | |
Experimental
Reagents and instruments
All chemicals and solvents were of analytical grade and commercially available. Ferrous sulfate was purchased from Tianjin Fengchuan Chemical Reagent Technology Co., Ltd. PEI (1800), DHBFA, and Cr(VI) were bought from Shanghai Aladdin Biochemical Technology Co., Ltd. Double-distilled deionized water was used throughout all the experiments. All glassware was washed with aqua regia and rinsed with deionized water.
Discover SP (CEM, America) was used for the complex of PS-Fe2+ synthesis. Ultraviolet-visible (UV-vis) absorption spectroscopy was performed on a UV-2550 spectrophotometer (Shimadzu, Japan). An F-4600 fluorescence spectrophotometer (Shimadzu, Japan) was used for recording fluorescence spectra. The particle size was measured using a JSM-6700F transmission electron microscope (Japan). Fourier transform infrared spectroscopy (FTIR) was carried out using an FTIR-8400 Fourier transform infrared spectrometer (Shimadzu, Japan). X-ray photoelectron spectroscopy (XPS, Shimadzu Japan) was used to characterize PS-Fe2+.
Preparation of PS-Fe2+
PS-Fe2+ were synthesized through the microwave-assisted synthesis. Briefly, 1 mL of FeSO4 (0.1 mM), 4.0 mL of DHBFA (0.1 mM) and 4.0 mL of PEI (0.1 mM) were added into a flask, and the mixture was then diluted with water to 10 mL. The system was placed in a microwave generator with the microwave power of 325 W for 3 min, which was then cooled to room temperature and stored in dark at room temperature for further usage. Experiments were carried out with different reaction times, microwave power and composition of reactants to determine the optimum conditions.
Optical properties of PS-Fe2+ for Cr(VI) sensing
All the detection experiments were measured in aqueous solutions with appropriate pH values. The fluorescent spectra of the analyte added PS-Fe2+ solutions were recorded by a fluorescence spectrometer with the excitation at 400 nm for 30 min. Parameters including reaction temperature, time-dependent responses, and pH values during the detection process were measured to optimize the detection performance of PS-Fe2+ towards Cr(VI) detection. Further, fluorescent responses of PS-Fe2+ towards various analytes including Cr2O72−, Ca2+, Mg2+, Al3+, Na+, K+, CO32−, HCO3−, F−, Br−, NO3−, Cl−, OH− and Cr3+ were measured, respectively, to evaluate the specificity. Then, the sensitivity of PS-Fe2+ towards Cr(VI) was evaluated upon addition of numerous concentrations of Cr(VI) into the PS-Fe2+containing system and measured by the fluorescence spectrometer.
Results and discussion
Synthesis of the water-soluble PS-Fe2+
The microwave-assisted synthesis can effectively improve the complex synthetic efficiency with high yield and purity.26,27 Based on this strategy, we used DHBFA, PEI and ferrous sulfate as raw materials to synthesize PS-Fe2+ under microwave irradiation via a one-pot synthesis. BesidesFe2+, other ions including Fe3+, Zn2+, Ni2+, Co2+ were introduced to the reaction system, respectively. The corresponding fluorescence properties of the obtained products were tested and only the introduction of Fe2+ could induce green fluorescence emission (Fig. 2A). Then, we evaluated the different reaction conditions to optimize the preparation of the complex. As shown in Fig. 2B–D, the gradual elevation of the DHBFA, PEI or ferrous sulfate ratio induced distinct fluorescence intensity enhancement and then decreased, which obtained the best molar ratio (2
:
2
:
3) of ferrous sulfate, DHBFA and PEI. Further, reaction microwave power (Fig. 2E) and time (Fig. 2F) tests showed that 325 W and 3 min irradiation were the best conditions for the PS-Fe2+ synthesis, respectively.
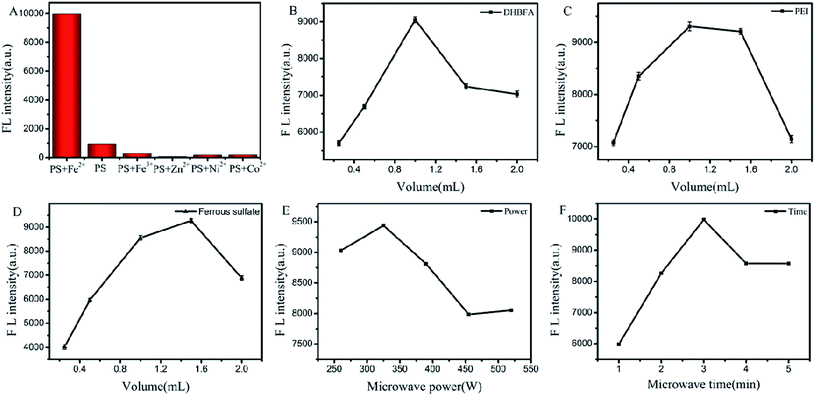 |
| Fig. 2 The optimization of the synthesis conditions of PS-Fe2+ upon control variable method. (A) Selection of the metal ions for Schiff base complexes (Fe2+, Fe3+, Zn2+, Ni2+, and Co2+); (B) the volume of the DHBFA solution (1 mM stock solution); (C) the volume of the PEI solution (1 mM stock solution); (D) the volume of the ferrous sulfate solution (1 mM stock solution); (E) the microwave power study; and (F) the irradiation time study. λex = 400 nm, slit: 10 nm/10 nm. | |
Characterization of PS-Fe2+
PS-Fe2+ was prepared under the optimized conditions. In order to prove the successful preparation of PS-Fe2+, fluorescence spectra of PS-Fe2+, PS, DGBFA, PEI, Fe2+, DGBFA + Fe2+, PEI + Fe2+ were recorded upon excitation at 400 nm. All configuration concentrations were 1 mM. Except for Fe2+ (1.5 mL), the quantity of all the others were 1 mL. As shown in Fig. 3A, only PS-Fe2+ shows a fluorescence maximum emission peak at approximately 505 nm. Corresponding to the fluorescence emission spectrum of the obtained PS-Fe2+, its UV-vis absorption spectrum displayed a distinct signal at 343 nm (Fig. 3B). The shape and size of PS-Fe2+ were then examined via TEM. As shown in Fig. 3C, the as-synthesized PS-Fe2+ appears as dendritic particles with good dispersity. The average diameter is about 4 nm. The FTIR spectrum was obtained to understand the structure of PS-Fe2+. In the FTIR spectrum of DHBFA, distinct absorption band displayed at 3000–3600 cm−1, which represented the vibration of the salicylaldehyde moiety with intramolecular hydrogen bonds (Fig. 3D). After the reaction, the peak in the corresponding region narrowed notably, which might be caused by the inhibition of the original intramolecular hydrogen bonds. In addition, DHBFA has two hydroxyl peaks, while PS-Fe2+ has only one redshifted hydroxyl peak, proving that a complex of ring conjugate was synthesized due to complexation of O and Fe2+. Simultaneously, the original signal at 1572 cm−1, which represented the vibration of C
O in DHBFA, disappeared, while a new signal at 1651 cm−1 appeared, which is the characteristic stretching vibration signal of C
N.28,29 In the XPS results (Fig. 3E), the binding energy of Fe 2p3/2 for PS-Fe2+ was 708.247 eV, which indicated the existence of Fe(II) in PS-Fe2+. The specific binding energies of elements including C, N and O appeared significantly (Fig. 3F). The above results demonstrated that fluorescent PS-Fe2+ was obtained utilizing the aforementioned method. The fluorescence quantum yield of the obtained PS-Fe2+ was about 55% using quinine sulfate (58% in 0.1 M H2SO4) as a standard.
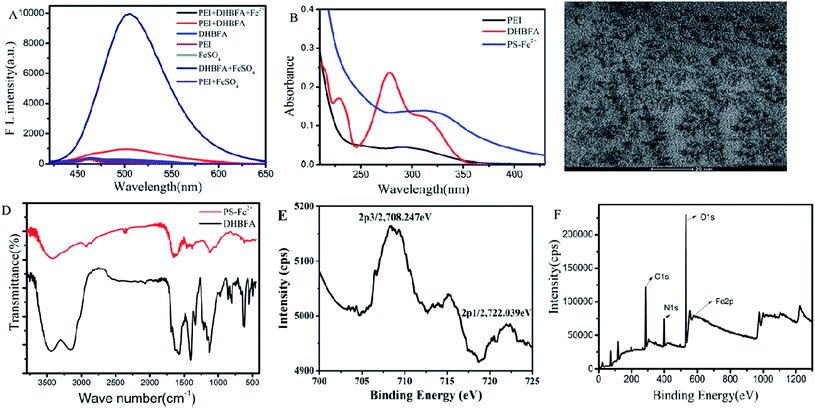 |
| Fig. 3 Characterization of PS-Fe2+. (A) Fluorescence emission of the synthesis system containing the following species, respectively: (1) PEI + DHBFA + Fe2+, (2) PEI and DHBFA, (3) PEI, (4) DHBFA, (5) Fe2+, (6) Fe2+ and PEI, (7) DHBFA and Fe2+. (B) UV-vis absorption of PEI, PS-Fe2+ and DHBFA; (C) TEM image of PS-Fe2+, scale bar: 20 nm; (D) FTIR spectrum of DHBFA (black line) and PS-Fe2+ (red line); (E) XPS spectrum of Fe 2p for PS-Fe2+; and (F) XPS spectrum of PS-Fe2+. | |
Results for the high-resolution spectra (Fig. S2†) indicated that the high-resolution C 1s spectrum could be deconvoluted into two peaks (285.08, 287.33 eV), corresponding to C–C/C
C, C–N/C–O bonds, respectively. Peaks were also recorded in the high-resolution N 1s spectrum (398.14 eV), corresponding to pyridinic C
N and amino N bonds, respectively. The presence of oxygenated carbon atoms (C–O/C–OH) can be verified from the spectrum peak (531.83 eV) recorded for high-resolution O 1s.30 Results from FT-IR and XPS analyses are consistent, suggesting that –NH2 and –OH groups are present on PS-Fe2+, enabling PS-Fe2+ to have excellent water solubility and potential further application prospects in organisms.
The structures of Schiff base, Schiff base-Fe2+ and Schiff base-Fe3+ were optimized and their corresponding molecular orbital energy levels were calculated at the PBE1PBE/def2tzvp level.31 As shown in Fig. 4, the chelation of Fe2+ lowered the HOMO energy level of the Schiff base. The results show that PS with Fe2+ generates a circular fluorescent probe. Simultaneously, after the oxidation of Fe2+ to Fe3+, the HOMO energy level of the complex further decreases, which supports the oxidation-based Cr(VI) detection process.
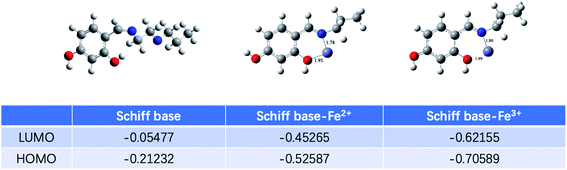 |
| Fig. 4 Optimized structures of the Schiff base, Schiff base-Fe2+ and Schiff base-Fe3+ and their corresponding molecular orbital energy levels calculated by Gaussian 09W at the PBE1PBE/def2tzvp level. | |
The stability of PS-Fe2+ was further evaluated via the monitoring of its fluorescent intensity at 505 nm upon temperature, standing time change and pH. As shown in Fig. 5, the solution of PS-Fe2+ is inert in the temperature range of 20–60 °C (Fig. 5A). The fluorescence intensity was stable within 90 min (Fig. 5B) and in the pH ranges from 6 to 9 (Fig. 5C). Besides, the appearance of redox substances such as Na2SO3 and H2O2 or NaCl did not interfere the fluorescent properties of PS-Fe2+ (Fig. S4†). The present results not only demonstrate the high stability of the detection system, but also supported the possibility to detect Cr(VI) with high specificity.
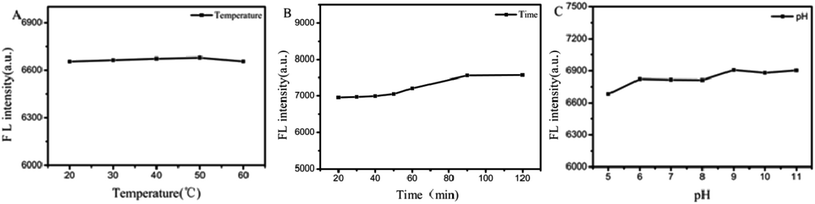 |
| Fig. 5 Temperature (A), time (B) and pH (C) dependent fluorescence intensity stability of the PS-Fe2+. λex = 400 nm, slit: 10 nm/10 nm. | |
Fluorescent responses of PS-Fe2+towards Cr(VI)
Supported by the aforementioned results, we then measured the possibility of PS-Fe2+ to detect Cr(VI) in aqueous solutions. Fig. 6A shows that there was an obvious decrease in the fluorescence intensity with the addition of Cr(VI) to the PS-Fe2+ solution, which provided the possibility to detect Cr(VI) quantitatively with PS-Fe2+ via a turn-off fluorescent method. To ensure the reliability of the detection process, we incubated the reaction mixture for different periods and the result showed that the reaction balanced within 15 min and the extended reaction time did not induce significant response change (Fig. 6B). Thus, the subsequent fluorescent data were all obtained 30 min after the analyte addition. Further, the reaction temperature-related response changes were also evaluated and increased temperature did not induce significant fluorescent quenching change (Fig. 6C). Besides, pH ranges from 6 to 8 did not interfere with the detection process, which permitted the application of PS-Fe2+ for Cr(VI) detection in numerous systems (Fig. 6D). The detection process displayed maximal fluorescence intensity quenching with 3.0 mL Tris–HCl buffer pH 6.0 (Fig. 6E and F). Thus, the Tris–HCl buffer was chosen as the detection system in the following experiments.
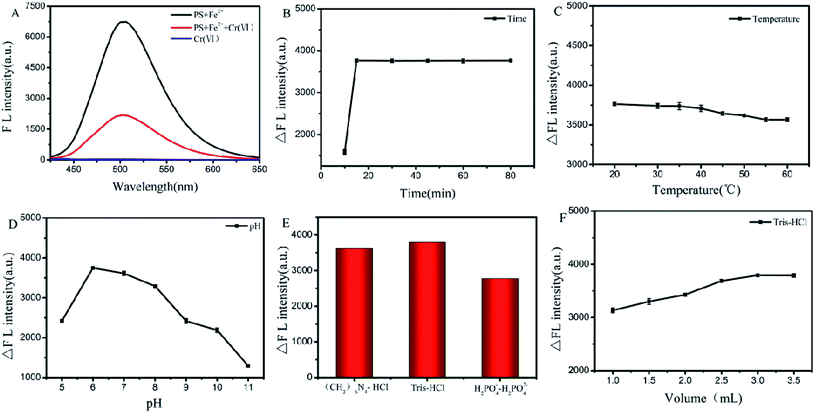 |
| Fig. 6 The optimization of the detection conditions of PS-Fe2+ for Cr(VI). (A) The fluorescence intensity of Cr(VI) to PS-Fe2+; (B) reaction period-induced response changes; (C) reaction temperature dependency response changes; (D) pH dependency response changes; (E) the buffer solution dependency response changes; and (F) selection of the amount of Tris–HCl. ΔF = F0 − F. λex = 400 nm, slit: 10 nm/10 nm. | |
The fluorescence spectra of the PS-Fe2+-containing solution for different concentrations of Cr2O72− were tested under the optimized reaction conditions. As shown in Fig. 7A, the inherent fluorescence intensity of PS-Fe2+ at 500 nm decreased gradually upon the addition of Cr2O72−. The fluorescence intensities of the detection system changed linearly with the concentrations of Cr2O72− ranging from 0.4 to 80 μM [ΔF = 52.2C + 839.7 (R2 = 0.9935)] (Fig. 7B). The corresponding detection limit based on the IUPAC definition (CDL = 3 sb per m) was 0.18 μM from 10 blank solutions. For a fluorescent probe, specificity is the most important character to support its applications. The fluorescent responses of PS-Fe2+ towards numerous potentially interfering substances (Cr2O72−, Ca2+, Mg2+, Al3+, Na+, K+, CO32−, HCO3−, F−, Br−, NO3−, Cl−, OH−, Cr3+, Cu2+, Ni2+, Mn2+, Pb2+, Zn2+, Ag+, Hg+, Co2+, Ba2+, Cd 2+,Cr3+, SO42−, NO2−, SCN−, PO43− and C2O42−, 2 equiv. over Cr(VI)) were then evaluated. As shown (some of the ions, Fig. S3†) in Fig. 7C, only Cr2O72− induced distinct fluorescent intensity decrease. The above-mentioned results demonstrated that PS-Fe2+ could be used for Cr(VI) detection quantificationally with high specificity.
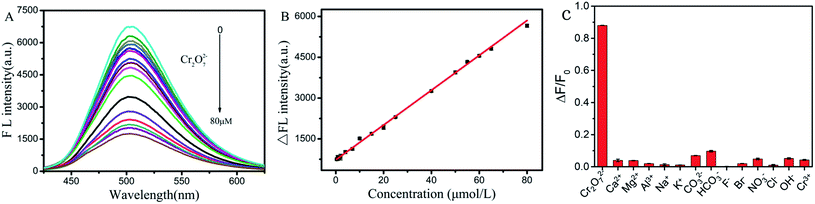 |
| Fig. 7 Quantification of the detection of Cr(VI) using PS-Fe2+. (A) Fluorescence quenching of the PS-Fe2+-containing system upon different concentrations of Cr2O72− (0, 0.2, 0.4, 0.6, 0.8, 1, 2, 4, 6, 8, 10, 12, 20, 30, 40, 50, 60, 70, and 80 μM) addition; (B) working curve of PS-Fe2+ to detect Cr2O72−; (C) relative emission intensity decrease (ΔF/F0) of the PS-Fe2+ in the presence of 0.1 mM of numerous analytes including Cr2O72−, Ca2+, Mg2+, Al3+, Na+, K+, CO32−, HCO3−, F−, Br−, NO3−, Cl−, OH−, Cr3+, respectively. F0 and F are the fluorescent intensities of the solutions at 500 nm without and with the addition of analytes, respectively. ΔF = F0 − F. λex = 400 nm, slit: 10 nm/10 nm. | |
Detecting Cr(VI) in tap water
In order to evaluate the practical utility of PS-Fe2+ to determine Cr(VI) in a real scenario, experiments were carried out to detect Cr(VI) in tap water by the recovery of standard addition. In a typical experiment, filtrated tap water (pH 6.0 adjusted by the addition of Tris–HCl) samples were spiked with different concentrations of Cr2O72− (5 μM, 10 μM, and 20 μM) in the presence of PS-Fe2+, and the concentration of Cr2O72− was determined using the calibration curve shown in Table 1. Recoveries ranged from 99.8% to 103.8% which demonstrated the applicability of PS-Fe2+ for Cr(VI) detection in real water samples. Therefore, the method can replace other methods to determine trace Cr(VI) in water.
Table 1 Detection of Cr(VI) in tap water
Sample |
Cr2O72− added (μM) |
Cr2O72− recovered (μM) |
Recovery (%) |
RSD (%) |
Tap water |
5 |
4.99 |
99.8% |
2.52 |
10 |
10.38 |
103.8% |
3.41 |
20 |
20.44 |
102.2% |
1.83 |
The linear range and detection limit of our study were compared with other fluorescence methods for Cr(VI) detection (Table 2). It can be seen that the detection limit of our study was relatively lower.
Table 2 Comparison of methods for Cr(VI) determination
Methods |
Linear range (μM) |
Detection limit (μM) |
Reference |
g-C3N4 fluorescence probe |
0.6–300 |
0.15 |
6 |
SiNP fluorescence sensor |
0.1–200 |
0.028 |
32 |
GQD-modified membranes |
1–500 |
0.19 |
33 |
CD fluorescence sensor |
2–180 |
2.10 |
34 |
CQD fluorescence sensor |
2.0–71 |
0.003 |
35 |
P,N-CD fluorescence sensor |
1.5–30 |
0.023 |
36 |
1,8-Naphthalimide fluorescence sensor |
2–90 |
0.36 |
37 |
PS-Fe2+ fluorescence sensor |
0.4–80 |
0.18 |
This work |
Conclusion
In summary, the preparation of water dispersible PS-Fe2+ was reported via a facile, environmentally friendly one-pot microwave-assisted synthesis methodology. The PS with weak fluorescence emission was synthesized by PEI and DHBFA under microwave heating, and PS could chelate ferrous ions to obtain a new iron-based complex with strong fluorescence emission at 500 nm and excellent stability in aqueous solutions. The fluorescence emission could be quenched specifically by the redox reaction of Cr(VI) and Fe2+. Simultaneously, we further investigated the on–off–on sensing property of the PS-Fe2+ by adding Cr(VI) to the PS-Fe2+ fluorescent probe. By the redox reaction of Fe2+ and Cr(VI), the concentration of the complex decreased, resulting in reduced fluorescence. The corresponding fluorescence intensity decreases are linear related to the concentration of Cr(VI) to realize Cr(VI) detection and the corresponding detection limit was calculated to be 0.18 μM. In addition, the proposed fluorescence method was successfully applied to the real sample analysis for the detection of Cr(VI) in drinking water samples with acceptable precision. The current synthesis method and the detection strategies for Cr(VI) may provide an easier and inexpensive process for the development of sensors for both environmental, biological applications, and domestic drinking water.
Conflicts of interest
There are no conflicts of interest to declare.
Acknowledgements
This work is supported by Physical chemistry key discipline project (Discipline team building and talent training) and sponsored by the Fund for Shanxi “1331 Project” Key Subjects Construction Plan.
References
- Y. H. Zhang, X. Fang, H. Zhao and Z. X. Li, Talanta, 2018, 181, 318–325 CrossRef CAS.
- S. I. Ohira, K. Nakamura, C. P. Shelor, P. K. Dasgupta and K. Toda, Anal. Chem., 2015, 87, 11575–11580 CrossRef CAS.
- S. Huang, H. N. Qiu, F. W. Zhu, S. Y. Lu and Q. Xiao, Microchim. Acta, 2015, 182, 1723–1731 CrossRef CAS.
- S. K. Tammina and Y. L. Yang, J. Photochem. Photobiol., A, 2020, 387, 112–134 CrossRef.
- A. Drinčić, T. Zuliani, J. Ščančar and R. Milačič, Sci. Total Environ., 2018, 638, 1286–1294 CrossRef.
- F. Petrucci and O. Senofonte, Anal. Methods, 2015, 7, 5269–5274 RSC.
- J. F. Guo, C. J. Hou, M. Yang, D. Q. Huo and H. B. Fa, RSC Adv., 2016, 6, 104693–104698 RSC.
- H. Y. Li, D. Li, Y. Guo, Y. Yang, W. Wei and B. Xie, Sens. Actuators, B, 2018, 277, 30–38 CrossRef CAS.
- R. Lv, J. Y. Wang, Y. P. Zhang, H. Li, L. Y. Yang, S. Y. Liao, W. Gu and X. Liu, J. Mater. Chem. A, 2016, 4, 15494–15500 RSC.
- M. C. Rong, L. P. Lin, X. H. Song, Y. R. Wang, Y. X. Zhong, J. W. Yan, Y. F. Feng, X. Y. Zeng and X. Chen, Biosens. Bioelectron., 2015, 68, 210–217 CrossRef CAS.
- N. S. Lawrence, R. P. Deo and J. Wang, Anal. Chim. Acta, 2004, 517, 131–137 CrossRef CAS.
- T. N. Ravishankar, Anal. Methods, 2015, 7, 3493–3499 RSC.
- V. Arancibia, M. Valderrama, K. Silva and T. Tapia, J. Chromatogr. B: Anal. Technol. Biomed. Life Sci., 2003, 785, 303–309 CrossRef CAS.
- A. N. Anthemidis, G. A. Zachariadis, J. S. Kougoulis and J. A. Stratis, Talanta, 2002, 57, 15–22 CrossRef CAS.
- M. Wang, R. Shi, M. J. Gao, K. L. Zhang, L. L. Deng, Q. F. Fu, L. J. Wang and D. Gao, Food Chem., 2020, 318, 126506 CrossRef CAS.
- L. Y. Li, F. F. Chen, J. f. Pan, S. h. Zhong, L. y. Li and Y. Yu, J. Lumin., 2020, 226, 117440 CrossRef CAS.
- Z. Q. Han, L. Qi, G. Y. Shen, W. Liu and Y. Chen, Anal. Chem., 2007, 79, 5862–5868 CrossRef CAS.
- W. Jin, G. S. Wu and A. C. Chen, Analyst, 2014, 139, 235–241 RSC.
- X. Chen, T. Pradhan, F. Wang, J. S. Kim and J. Yoon, Chem. Rev., 2012, 112, 1910–1956 CrossRef CAS.
- A. P. Rawat and D. P. Singh, Ecotoxicol. Environ. Saf., 2019, 176, 27–33 CrossRef CAS.
- D. Wu, A. C. Sedgwick, T. Gunnlaugsson, E. U. Akkaya, J. Yoon and T. D. James, Chem. Soc. Rev., 2017, 46, 7097–7472 RSC.
- J. H. M. van der Velde, J. Oelerich, J. Huang, J. H. Smit, A. Aminian Jazi, S. Galiani, K. Kolmakov, G. Gouridis, C. Eggeling, A. Herrmann, G. Roelfes and T. Cordes, Nat. Commun., 2016, 7, 10137–10144 Search PubMed.
- H. D. Li, L. L. Li and B. Z. Yin, Inorg. Chem. Commun., 2014, 42, 1–4 CrossRef CAS.
- H. M. Wu, Y. Guo and J. F. Cao, Chin. J. Anal. Chem., 2018, 46, 379–385 CAS.
- X. G. Li, X. M. Wu, F. Zhang, B. Zhao and Y. Li, Talanta, 2019, 195, 372–380 CrossRef CAS.
- G. Kumar, K. Paul and V. Luxami, Sens. Actuators, B, 2018, 263, 585–593 CrossRef CAS.
- J. A. Gerbec, D. Magana, A. Washington and G. F. Strouse, J. Am. Chem. Soc., 2005, 127, 15791–15800 CrossRef CAS.
- A. B. Panda, G. Glaspell and M. S. El-Shall, J. Am. Chem. Soc., 2006, 128, 2790–2791 CrossRef CAS.
- P. J. Li, Y. Y. Hong, H. T. Feng and S. F. Li, J. Mater. Chem. B, 2017, 5, 2979–2988 RSC.
- S. R. Zhang, L. X. Jin, J. Liu, Q. Wang and L. Jiao, Mater. Chem. Phys., 2020, 248, 122912 CrossRef CAS.
- X. Q. Lu, Q. Chen, X. X. Tian, Y. W. Mu, H. G. Lu and S. D. Li, Nanoscale, 2019, 11, 21311–21316 RSC.
- P. M. Carrasco, I. Garcia, L. Yate, R. T. Zaera, G. Cabanero, H. J. Grande and V. Ruiz, Carbon, 2016, 109, 658–665 CrossRef.
- L. Zhu, X. Peng, H. Li, Y. Zhang and S. Yao, Sens. Actuators, B, 2017, 238, 196–203 CrossRef CAS.
- C. Li, W. J. Liu, X. B. Sun, W. Pan and J. P. Wang, Sens. Actuators, B, 2017, 252, 544–553 CrossRef CAS.
- T. T. Zhou, Z. Z. Wang, R. X. Ma, Y. Wang, L. J. Gao and X. H. Sun, Metall. Anal., 2016, 36, 59–64 Search PubMed.
- X. J. Gong, Y. Liu, Z. H. Yang, S. M. Shuang, Z. Y. Zhang and C. Dong, Anal. Chim. Acta, 2017, 968, 85–96 CrossRef CAS.
- Z. Y. Zhang, C. M. Sha, A. F. Liu, Z. Y. Zhang and D. M. Xu, J. Fluoresc., 2015, 25, 335–340 CrossRef CAS.
Footnote |
† Electronic supplementary information (ESI) available. See DOI: 10.1039/d0ra07458f |
|
This journal is © The Royal Society of Chemistry 2021 |
Click here to see how this site uses Cookies. View our privacy policy here.