DOI:
10.1039/D0QM00509F
(Review Article)
Mater. Chem. Front., 2021,
5, 76-96
Recent progress in quinoidal semiconducting polymers: structural evolution and insight
Received
18th July 2020
, Accepted 4th September 2020
First published on 7th September 2020
Abstract
The past decade has witnessed a visual explosion in the discovery of high-performance semiconducting polymers. A few representative materials among them have been subjected to the evaluation of their applicability in daily use. Although many design concepts have been extracted from a diverse array of such polymers, the structural aspects are still evolving and continue to fuel the development of flexible electronics. Among the newly developed systems, quinoid-containing polymers have shown promise in low-gap semiconductors as novel electronic materials. This review provides an overview of the recent progress in quinoidal polymer semiconductors for use in electronics, focusing on the construction of these quinoidal frameworks and their interesting electronic structures. We discuss the origin of the narrow gap and how this feature is appropriately leveraged to meet the requirements of high-mobility materials design. Also included is the derivatization for analog preparation to disclose the chemical space of such quinoid-based polymers.
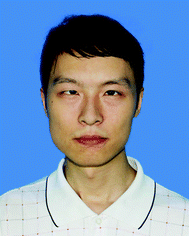
Jianyao Huang
| Jianyao Huang received his PhD degree in organic chemistry from the Institute of Chemistry, Chinese Academy of Sciences in 2015. His research interests include organic electronics and functional organic field-effect transistors. |
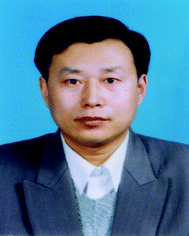
Gui Yu
| Gui Yu is currently a professor at the Institute of Chemistry, Chinese Academy of Science. He graduated from Jilin University in 1998 and received his MS (1993) and PhD (1997) degrees from the Changchun Institute of Physics, Chinese Academy of Science. After obtaining his PhD, he worked as a postdoctoral fellow at the Institute of Chemistry. Thereafter, he was appointed to the position of Associate Professor in 1999 and promoted to Professor in 2008. His current research mainly focuses on the synthesis of organic/polymers, graphene, and covalent organic frameworks. Simultaneously, the optoelectronic and spintronic devices of these materials are discussed. |
1. Introduction
Modern electronics, which requires biocompatibility, flexibility, and mechanical robustness has driven the rapid development of organic semiconductors.1–5 Solution-processable polymeric semiconductors serve as good candidates for large-area fabrication. In addition, their custom-tailored functionality and unparalleled chemical space promise a host of applications responsible for transformative technologies, including active matrix organic light-emitting diode displays, stretchable skin sensors, and e-papers, which bode well for our daily life.6–10 The fine-tuning of the charge transport property of the semiconducting layer is crucial for realizing their potential. In particular, the improvement in the charge transport behavior depends on the ability to control the structural aspects across a wide range of length scales. Materials chemistry endeavors have been attempted using a set of tools to probe into the structure–property relationship on and beyond the molecular formula, especially since the advent of polymer semiconductors with alternating donor–acceptor motifs. This paradigm is still evolving, with more interesting structural families being incorporated.
Semiconducting polymers with quinoidal building blocks are such an emerging class of materials. The term “quinoid” originates from quinone, a typical oxidative state of aromatic compounds obtained by the conversion to cyclic diones and the rearrangement of the bond types in rings. In contrast to their aromatic counterparts, quinoids show significant bond length alternations that break the aromaticity, reduce the highest occupied molecular orbital (HOMO)–lowest unoccupied molecular orbital (LUMO) gaps that potentially enable injections of both electrons and holes, different chemical reactivities that require synthetic efforts, and low-energy and low-symmetry spectroscopic features that guarantee new functionalities. For small-molecule semiconductors, this structure enjoys widespread use in n-type materials because of the ease of electron injection leveraging the combined contribution of small energy gap and low-lying LUMO energy levels. Examples include tetracyanoquinodimethane analogues and indenofluorenes.11–14 Sophisticated analyses have been employed to showcase their ability for excellent electron transport. By contrast, a polymeric framework with quinoidal subunits is still in its infancy. To establish a paradigm for the rational design of quinoid-containing polymers, the daunting synthetic challenge is needed to be overcome and disclose the latent theoretical underpinnings. These efforts potentially expand the scope of this class of materials.
Notably, quinoidal structures are often associated with diradicaloid or antiaromaticity in a manner reminiscent of their shared 4nπ electronic structures and diradical resonance forms. Another oft-encountered concept in semiconductor design is the quinoidal character. This terminology pervades the literature but is typically referred to as the quinoidal resonance forms of redox active species, such as thienoacene and polythiophene derivatives.15–18 Although such features contribute in part to the opto-electronic properties, their structures are different from those with inherent ground-state quinoidal subunits demonstrated of late. Fig. 1 depicts the difference between the aromatic and quinoidal compounds, as well as those with quinoidal characters in the resonance forms. From a synthetic perspective, a ground-state quinoidal building block holds a conceptual appeal for the modification of polymer backbones. We highlight that such materials containing inherently quinoidal structures are newcomers in the polymeric semiconductor family. In this context, this class of materials is attractive in terms of their physical properties and relatively unexplored chemical space. Introducing quinoidal structures to a fully aromatic backbone can tune the frontier orbital energetics owing to the hybridization and add more sites for chemical functionalization. Simply put, studies on these materials open avenues for the formulation of design rationales for polymeric semiconductors.
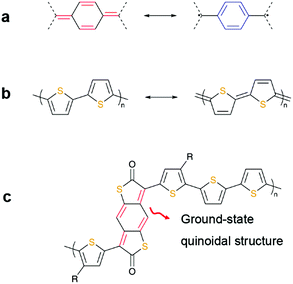 |
| Fig. 1 Quinoid structures. (a) Resonance effect of quinoid structures. (b) Quinoidal character in polythiophene (not a ground-state quinoid-containing material). (c) A typical quinoid-containing semiconducting polymer. | |
In this review, we aim to focus on quinoidal structures introduced into polymer semiconductors and to compare their features with other conventional building blocks. Although a few early reports have reviewed quinoidal semiconductors for use in transistors, the major concern is small-molecule materials or polymers with typical quinoidal characters.19,20 We highlight the quinoidal family of polymer semiconductors in this review and give an overview about how the structural difference affects the electronic structure and hence the performance. The following part focuses on the design strategy towards these inherently quinoidal substructures. Then we show case studies about the quinoid semiconducting polymers by the modification of different core structures. We note that many semiconducting polymers were not recognized as quinoidal materials at the stage when they were developed for the first time. Some quinoidal subunits were simply considered as electron-accepting building blocks. Detailed structural information involving the rational design is still limited for these materials. We discuss the synthesis, electronic structures, and corresponding charge transport behaviors of quinoidal polymers, and compare them with structural analogs. Well-developed small-molecule quinoidal semiconductors (e.g., tetracyanoquinodimethane congeners and structural analogs thereof) and polymers with quinoidal character (e.g. polythiophene) are beyond the scope of this review, despite the important concepts referred to in these materials.
2. General structural parameters towards designing polymer semiconductors
The pursuit of high-mobility polymer semiconductors has inspired design strategies far beyond the simple permutation of comonomers. An increasing demand for understanding the structural aspects has driven cutting-edge studies focusing on the tuning of electronic structures, conformations, and side-chain organizations.21–27 To date, the most mentioned structural descriptors for polymer semiconductors include conjugation, coplanarity, side-chain geometry, monomer symmetry, regioregularity, molecular weight, and defects. Conjugation is perhaps the most important parameter for polymer semiconductors. Studies about this parameter can be traced back to the early stage of development,28 specifically the structural isomers of poly-p-phenylene vinylene with linear- or cross-conjugation. Cross-conjugated materials show significant frontier orbital localization, which is an impediment to intrachain transport; on the other hand, linear-conjugation triggers this charge transport pathway.29–32 Coplanarity involves conformational control over single-bonded subunits. Enhanced coplanarity can simultaneously increase the effective conjugation and aggregation tendency. Side-chain geometry is a deterministic factor for intermolecular interdigitation, a feature that is associated with self-organization nature and crystallinity.33 Monomer symmetry influences backbone orientation, adding extra control over the aggregation behavior and long-range ordering.34 Similar effects are present for the regioregularity of the polymer.35–37 Molecular weight and defects are responsible for the batch-to-batch variations in charge transport performance; the reaction conditions should therefore be optimized to balance the solubility and self-assembly behaviors.38–42 Subtle changes in all these factors can lead to pronounced differences in the macroscopic observables, emphasizing the key role in establishing bridges from atomistic descriptors to the macroscale. Therefore, an appropriate balance between these factors is essential for optimizing the performance.
The design strategy towards polymer quinoids manifests itself in the tuning of electronic structures through the synergetic effect of bond-length alternation and equalization. Quinoidal heterocycles feature polarized structures and thus serve as embedded chromophores to provide structural modifiers. These modifications illustrate six important design purposes towards polymer semiconductors (Fig. 2) as follows:
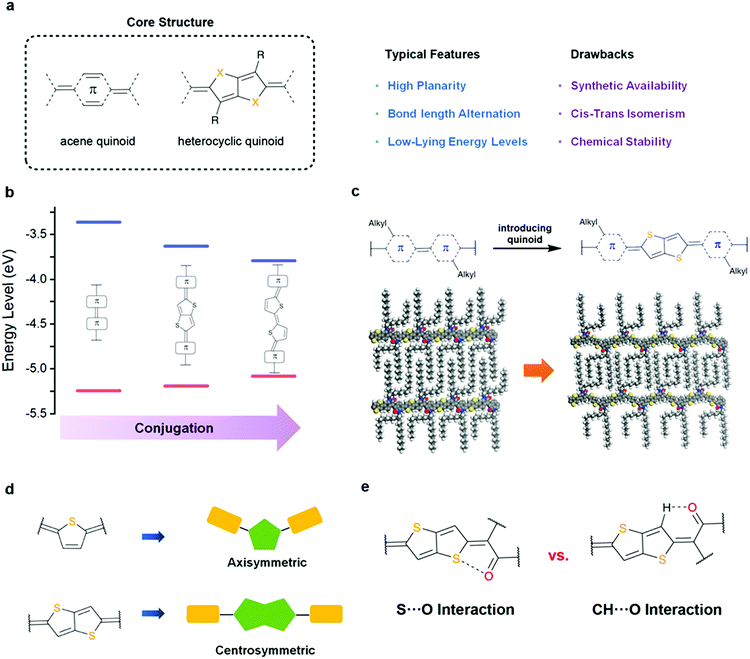 |
| Fig. 2 Schematic diagrams for the design of quinoidal semiconducting polymers. (a) Different core structures for quinoidal building blocks. (b) Energetic tuning by introducing quinoids with different conjugation length. (c) Varied packing motifs after the introduction of quinoid subunits into the building block. (d) Different molecular symmetries of heterocyclic quinoids. (e) Competitive non-covalent interactions and configurations after the introduction of quinoids. | |
(1) A typical method for introducing quinoids is π-extension. This strategy, which is conceptually straightforward for target-oriented quinoid synthesis, streamlines the design effort and is compatible for further functionalization. Unlike aromatic units, quinoids either displace the double bonds or are inserted into the original cyclic compound in a fused fashion, both of which retain the molecular planarity and avoid conformational ambiguity. In some cases, however, this strategy allows for the emergence of cis–trans isomers, thereby leading to chemoselectivity in configurational control.43 Moreover, as the attachment sites for side chains move away from each other to varying degrees after π-extension, the relevant packing motif of the polymer chains can be substantially changed, with a propensity for densely packed, altered interdigitation.44 This modification leads to conceivable self-organization variants that are difficult for predicting their effects on the charge transport properties.
(2) Introducing a heterocyclic quinone as an electron-deficient moiety is another method for backbone engineering. This method is more precisely referred to as the reconstruction of the core rather than the modification of the original scaffold, in view of the significant change in the electronic structures. Few, if any, semiconducting characteristics prove to be predictable by the introduction of heterocyclic quinone subunits; however, multiple embedded or substituted heteroatoms provide an appealing platform for conformational control, which is essential for the planarization of the polymer backbone. In these cases, the flanking substituents with respect to the heterocyclic quinone, have a pronounced effect on the overall conformation and orientation, especially through the formation of Coulombic carbonyl oxygen-heteroatom attractions.45 Such conformational preference is also vital for rational design.
(3) The resulting reactivity of quinoid-embedded substructures is different from those of the aromatic rings. Due to its inherent electron deficiency, a quinoid-containing unit is used to build n-type or ambipolar polymer backbones. Currently, the polymerization of electronically active polymers depends on palladium-catalyzed reactions including the Stille and Suzuki coupling reactions. According to the reaction mechanisms, a progressive strengthening of the electron affinity may hamper the electrophilic transmetalation for the carbon–tin or carbon–boron bond cleavage, posing synthetic challenges in obtaining high molecular weight materials.46–48 Moreover, the double bond in quinoid is susceptible to addition reactions such that an explicitly designed route different from simple condensation should be taken into account for the preparation and further functionalization. In addition, quinoids themselves function as oxidants, which likely limit the scope of the reactions utilized.
(4) The attachment sites for side chains depend highly on the type and chemical properties of heterocyclic quinoids. For example, pyrrolidone- or oxindole-based quinoids possess N-alkylation sites, whereas thienoquinoids have no positions for side-chain substituents. This structural difference can affect the intermolecular interactions involving microstructural and morphological optimization. Yet, the fundamental understanding of the modification of side-chain attachment remains unclarified; the optimal side chain is linked to the molecular geometry of the co-repeating unit and necessitates a balance between the functional criteria that are sometimes orthogonal.
(5) The molecular symmetry of the building block can be altered by the exchange of the quinoid subunits. Although a perfect stack of polymer chains favors centrosymmetric structures in an antiparallel fashion, axisymmetric units bring about new functionalities in the building block and occasionally do not affect the linearity of the backbone.49 To this end, the installation of axisymmetric heteroquinoids is also an approach for modifying the geometric and electronic structures.
(6) A judicious compromise of the other comonomer is needed to prevent excessively low-lying LUMO energy levels after quinoid introduction. This empiricism-driven structural evolution involves trial-and-error procedures to explore the chemical space for a given core of interest because the quantitative prediction of the charge transport behaviors is still lacking at present.
3. Categories of quinoidal polymer semiconductors
The design of a quinoidal molecular framework is typically based on a core structure. In this section, we discuss the synthesis, electronic structure, and physical properties of different quinoidal polymer semiconductors, in view of their structural features in parent cores. Given that various ring fusion and redox processes are involved, a one-size-fits-all synthetic route for such quinoid building blocks is elusive. We categorize these quinoid polymers into three distinct groups in terms of their prominent structural features, each of which represents an intriguing core. These core structures include oxindole-capped quinoids, azaquinoids, thienoquinoids, and pyrazinoquinoids.
3.1 Semiconducting polymers based on oxindole-capped quinoids
As a conventional building block in the field of organic electronics, isoindigo represents the state-of-the-art in ready preparation, easy-to-manipulate complexity, and functional versatility.51 Isoindigo is routinely synthesized via the acid-catalyzed condensation of oxindole with isatin,52 or the reductive dimerization of individual isatin.53 To introduce a quinoidal structure into this symmetric molecule, bond disconnection conventionally takes place in the central double bond. Given that the retrosynthetic plan points to the final step of the double-bond forming event, the quinoidization of this molecule capitalizes on the simple derivatization of accessible precursors oxindole or isatin (Fig. 3). Replacing the internal carbon–carbon double bond with inserted quinoid structures leads to π-extension as well as a reduced HOMO–LUMO gap and enhanced planarity. Theoretical implications offer access to diradical resonance forms, rationalizing the open-shell tendency of these quinoids. The contribution of diradicals to the ground state is typically described by the diradical character index (y0), a parameter that reflects the occupation number of the lowest unoccupied natural orbital (LUNO).54 If a benzoquinoid spacer is introduced, the calculated y0 is 0.33, which is larger than that of the thienoquinoid counterpart (0.19).50 The greater y0 for benzoquinoid-containing compounds indicates a significant stabilization of the benzene-containing resonance forms. On the other hand, the corresponding quinoidal structure is chemically less stable, which validates its synthetic difficulty. The conjugation length is another factor affecting the diradical character. The bithiophene quinoid analog has a larger y0 than that of the thienothiophene quinoid structure, indicating that the extended conjugation of aromatic species is beneficial for the localization of the unpaired electrons in open-shell diradicaloids.
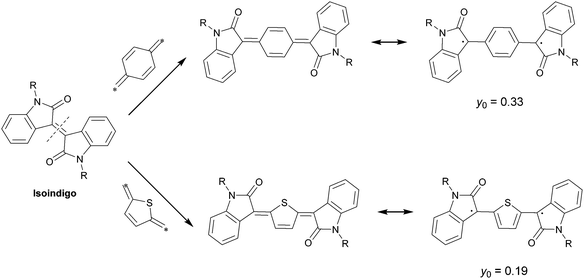 |
| Fig. 3 Resonance effect of oxindole-capped quinoidal structures. The diradical character index y0 indicates the different stability of the quinoidal compound. The values of diradical character indices are reported in ref. 50, American Chemical Society. | |
3.1.1 Quinoidal thiophene embedded structures.
A first example of oxindole-capped quinoid materials was reported by Kim's group.55,56 They used an alkylated indophenine, which has a quinoidal bithiophene spacer, as the semiconductor for field-effect transistor applications (Fig. 4). Indophenine was reported as a blue pigment in 1879 for the first time.57 This compound was an unexpected condensation product of the treatment of isatin with impure coal-tar benzene in sulfuric acid. The reactive impurity was later discovered as thiophene, a compound that participates in the condensation with protonated isatin. Six cis–trans isomers were detected by the nuclear Overhauser effect (NOE) studies.58 With this precedent, Kim et al. prepared a dodecyl-substituted indophenine with embedded quinoidal bithiophene (QBT) and a biselenophene derivative (QBS). They discussed the chalcogen effect, by which a small contribution to the frontier molecular orbital energies and a pronounced difference in crystallinity were found. Both molecules showed ambipolar transport behaviors with hole and electron mobilities of 0.031 and 0.005 cm2 V−1 s−1 for QBT, and 0.055 and 0.021 cm2 V−1 s−1 for QBS, respectively. Following these encouraging results, they further studied the polymeric system.56 They synthesized the 2-decyltetradecyl-substituted, brominated monomer using a similar protocol and coupled it with the vinylene moiety to assemble the polymer backbone (Fig. 5). This polymer P1 features a highly planar backbone, a low gap, as well as delocalized HOMO and LUMO, implying its ambipolar semiconducting transport behaviors. Despite the cis–trans isomerism, the fabricated field-effect transistors based on P1 exhibited hole and electron mobilities of up to 0.52 and 0.53 cm2 V−1 s−1. This work emphasizes the promise of quinoidal building blocks in polymer semiconductors.
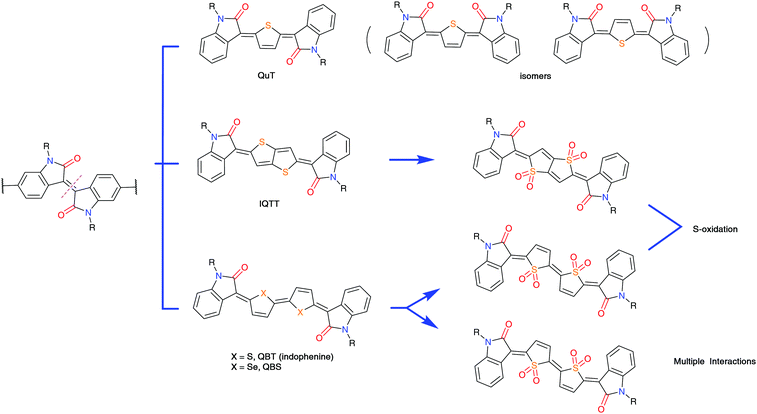 |
| Fig. 4 Structural evolution of quinoid building blocks with end-capped oxindole. | |
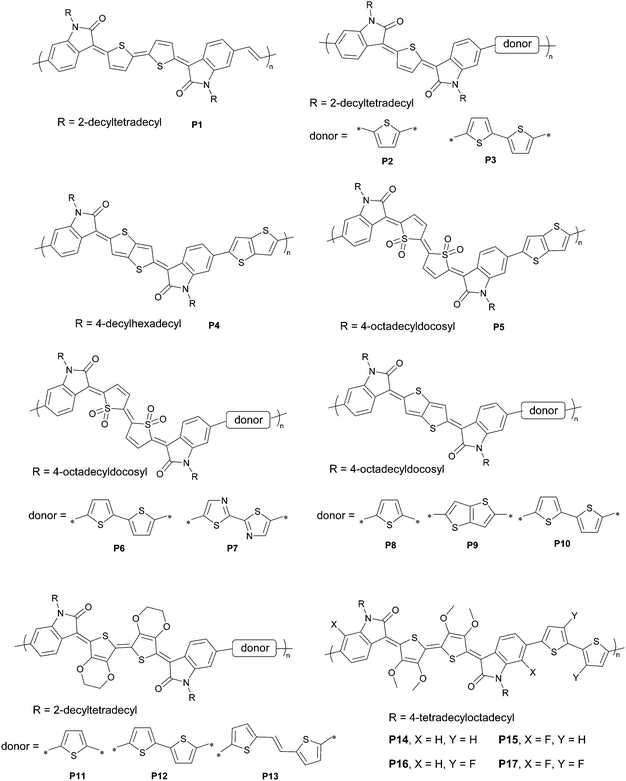 |
| Fig. 5 Chemical structures of quinoidal end-capped oxindole-based semiconducting polymers. | |
During the preparation of indophenine, a byproduct containing quinoidal monothiophene (QuT) was also separated. This compound was deemed as the product of 2-electron reduction involving a dication intermediate (Fig. 4).58 Interestingly, although this molecule possesses neither a center nor a plane symmetry, its electronic structure still holds promises for transistor applications. Similar to that in the bithiophene quinoid, the double-bonded linkage ensures the planarity of the backbone; as a result, the conformational freedom is restricted at the expense of the introduced configurational isomerism. Of the three isomers detected during the synthesis, only one was found to be the major product and could be isolated by repeated column chromatography. This asymmetric structure was further confirmed as the Z,E-isomer by 2D correlation spectroscopy and 2D nuclear Overhauser effect spectroscopy. The maximum hole mobilities of the copolymer P2 and P3 were 3.60 and 2.77 cm2 V−1 s−1 after thermal treatment at 250 °C, which increased to 4.82 and 8.09 cm2 V−1 s−1 using the off-center spin-casting technique, respectively. Although there is an overestimation to some extent, the study on high-mobility quinoidal polymers is still important in a practical sense.59
To explore the chemical space of these materials, a thienothiophene quinoid linkage was developed. In contrast to thiophene and bithiophene counterparts, a quinoidal thienothiophene spacer leverages its innate symmetric molecular geometry and reduced configurational disorder to build a polymer backbone. Unlike the indophenine synthesis, Reichmanis et al. prepared the quinoidal thienothiophene-based building block (IQTT) through a two-step sequence involving organolithium-mediated addition and SnCl2-mediated reduction (Fig. 4). Of particular interest has been the isolation of the final product. The major product showed cross-talk between the hydrogen atoms of the internal quinoidal thienothiophene and the peripheral oxindole by two-dimensional 1H–1H nuclear Overhauser effect spectroscopy, confirming its centrosymmetric geometry. This compound was copolymerized with the thienothiophene monomer to introduce both quinoidal and aromatic substructures. Bond-length alternation in the quinoidal structure is a typical feature proven by theoretical modeling. This alternation motif results in a lowered HOMO–LUMO gap and a different frontier orbital distribution in comparison with that of the isoindigo-based polymers. The thin-film transistor based on the IQTT-thienothiophene copolymer P4 showed a hole mobility of 0.13 cm2 V−1 s−1, which is slightly lower than that of the isoindigo-thienothiophene counterpart. This result implies a gap-narrowing strategy by the insertion of thienothiophene quinoids and expands the chemical space of thienoquinoid-embedded materials for organic electronics.
3.1.2 Configurational and conformational control in oxindole-capped structures.
The aforementioned reports focused on the structural development of quinoidal polymers. However, the introduced configurational isomerism is a detrimental factor for long-range ordering. The polymer backbone comprising isomeric substructures causes inevitable energetic disorder that functions as the trapping of carriers. In addition, the asymmetric substructure leads to uncontrollable side-chain organization that harms the formation of crystallites. To mitigate this undesirable property, Deng et al. developed a thiophene-S,S-dioxidized indophenine preparation wherein the most stable isomer could be accessed via mild thermal treatment.60 The original indophenine was a mixture of six isomers, whereas the oxidized product contains only (E,E,E), (Z,E,E), and (Z,E,Z) isomers that can be separated by column chromatography (Fig. 6). The latter two isomers were converted into the first one after storing at 0 °C for a few days. The authors then heated the mixture in boiling toluene to exclusively afford the stereoselective (E,E,E) isomer. Thermal isomerization is dominated by the innate bias of the chemical stability because the (Z,E,E) and (Z,E,Z) isomers have unfavorable Coulombic repulsions between the unshared electron pairs of oxygen atoms, whereas the (E,E,E) isomer is stabilized by the intramolecular H–O interactions. Single-crystal X-ray diffraction analysis further confirmed this stable isomer. Because of the significant lowering of the frontier orbital energy levels by sulfone incorporation, the HOMO and LUMO energy levels of the polymer were estimated to be −5.92 and −3.98 eV, respectively. This result envisioned a predominant electron transport behavior. They fabricated field-effect transistors with a bottom-gate/bottom-contact (BGBC) configuration to test the mobility. As expected, only the n-channel semiconductor characteristics of the polymer P5 were exhibited, with the highest electron mobility of 0.14 cm2 V−1 s−1. This report illustrates an example of quinoidal sulfone-embedded building blocks and the polymers thereof, highlighting the effect of the planar backbone on mobility improvement. The combination of sulfone and quinoid substructures, both of which are of high electron affinity, is a strategic design towards unipolar n-type polymer materials. In a following work, the authors replaced the comonomer with bithiophene (P6) and bithiazole (P7). The resulting polymers provided similar unipolar n-type transport with electron mobilities up to 0.18 cm2 V−1 s−1, thus diversifying the monomer scope for this building block.61
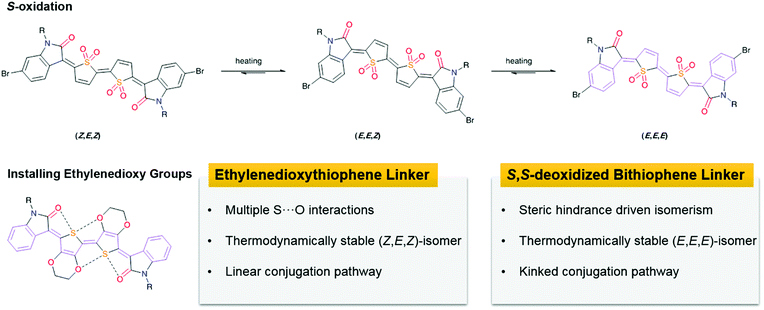 |
| Fig. 6
S-Oxidation of quinoidal thiophene and the installation of ethylenedioxy groups. | |
Inspired by these works, thienothiophene sulfone was then introduced to develop n-type materials.62 Isatin was first converted into quinoidal thienothiophene-oxindole monomer in a similar route and then oxidized to the sulfone mixture. Heating the mixture also led to thermal isomerization to the (E,E) isomer in high yields. A series of polymers were prepared using the Stille coupling reaction, with thiophene (P8), thienothiophene (P9), and bithiophene (P10) as the donor moieties. All these polymers showed unipolar n-type transport behaviors with the highest mobility of 0.45 cm2 V−1 s−1. The authors also discussed the effect of backbone curvature on the charge transport properties. This structural parameter is particularly important for this class of materials because the S,S-dioxidized monomer has a different configurational preference compared with those of the thienoquinoid-containing counterparts. The π-extension direction of the internal sulfone quinoids deviates from that of the donor moiety, potentially leading to reduced effective conjugation length and non-linear backbone geometry. Indeed, the pronounced backbone curvature is unfavorable for obtaining highly stacked aggregates because of its sensitivity to transverse displacement between neighboring polymer strains. The angle between the rigid substructures can be used to describe the linearity of the backbone. For the thienothiophene-sulfone quinoids, the angle was calculated as 143.4°, indicating its preference towards a linear polymer backbone compared with the bithiophene-sulfone quinoids, in which an angle of 131.8° was present. This influencing factor exemplifies the relationship between the backbone linearity and carrier mobility.
Using conformational control has proven to be an efficient method in semiconductor design. To maximize the configurational uniformity of the quinoidal core, additional functional groups were introduced in later reports.63 Such an emerging structural control was achieved by through-space weak interactions. Despite the prevalence of hydrogen bonds in conformational control, the chalcogen bond, especially the S–O interaction, also dictates the molecular geometry in semiconductor design. Hwang and co-workers replaced the thiophene with 3,4-ethylenedioxythiophene in the quinoidal spacer. This motif forms multiple S–O interactions, which constrain the rotation in the cation intermediate. This monomer has a symmetric (Z,E,Z)-configuration that is also driven by the steric repulsion of the oxygen-containing functionalities in the disfavored conformers. Organic field-effect transistors based on the copolymer P11 showed hole mobilities of up to 4.3 × 10−2 cm2 V−1 s−1. Likewise, Sun and co-workers capitalized on the steric control and intramolecular interactions of quinoidal dimethoxythiophene, a structure motif similar to that of ethylenedioxythiophene (P12–P17). By the introduction of fluorine substituents to further control the backbone conformation, the semiconducting polymers showed hole mobilities of up to 2.70 cm2 V−1 s−1.64 These experiments corroborate the established theory that non-covalent interactions have an impact on the structures of building blocks and accomplishes a molecular geometry different from that obtained through the S-oxidation strategy.
3.1.3 Comparison with isoindigo-based materials.
Structural analysis implies the influence of these molecular skeletons on side-chain interdigitation. Notably, the mobilities of the polymers with extended quinoidal units are lower than those of the isoindigo-based materials. This is due to the variation in the interdigitated motif, in which an increased distance between the attaching sites leads to a partially stacked pattern.44cis–trans isomerism also affects the overall backbone ordering, giving rise to a curved, stacking-disfavored aggregate. Mobility measurements emphasize that reduced configurational and conformational ambiguity is important for achieving high performance. In addition, these quinoidal polymers still have predominant hole transport behaviors owing to the moderately lowered LUMO energy levels. For S,S-dioxidized molecules, introducing additional electron-deficient functional groups can readily realize the conversion of charge transport polarity from p- to n-type. This strategy is applicable to other existing polymer semiconductors. The introduced sulfonyl group, however, protrudes out of the backbone plane and possibly affects the intermolecular packing to some extent. The experimental and theoretical underpinnings of these factors are still being studied in detail. Collectively, these newly developed materials are poised to transform the recognition of conventional donor–acceptor semiconducting polymers, providing new directions to functionalize benchmark materials or to construct new molecular scaffolds.
3.2 Semiconducting polymers based on azaquinoids and thienoquinoids
Quinoid derivatives of aromatic heterocycles are prevalent in organic chemistry. On the basis of the type of heteroatoms, azaquinoids and thienoquinoids are two typical classes of materials used in electronics. An azaquinoid typically features a bislactam structure, which is canonically defined as an amide-containing semiconductor and overlooks its innate quinoid character. The introduction of heteroatoms to quinoid frameworks polarizes the bonds for enhanced electronic effects and chromophoric matters. Herein, in this part, we discuss these materials in comparison to the isoelectronic thienoquinoids, and attempt to summarize the physical and chemical underpinnings of these materials (Fig. 7).
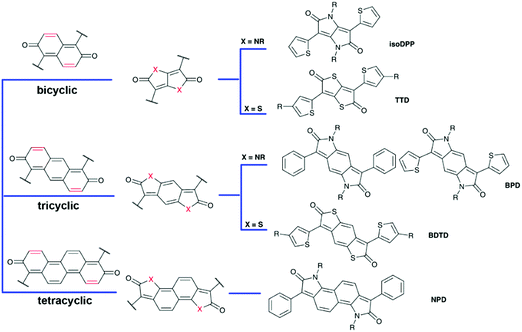 |
| Fig. 7 Bicyclic, tricyclic, and tetracyclic isoelectronic heteroquinone structures used as building blocks in semiconducting polymers. | |
As an isoelectronic analog of 2,6-naphthoquinone, pyrrolo[3,2-b]pyrrole-2,5-dione (hereafter referred to as isoDPP) and thieno[3,2-b]thiophene-2,5-dione (TTD) represent prototypical heteroquinoids. The unpaired electrons from nitrogen or sulfur atoms directly conjugate into the π-system to afford an isoelectronic structure similar to an aromatic sextet. The lactam and thiolactone structures for these units also provide a clue about the chemical functionality. These molecules can be substituted at 3,6-positions to build a backbone, in which the conjugation pathway resembles a butadiene in a fused manner. The carbonyl groups offer moderate-to-high electron-accepting capacities, together with a polarized structure, to fine-tune the frontier orbital energies. In this context, these moieties are promising candidates for electron-acceptors in polymeric semiconductors.
3.2.1 Bicyclic heteroquinoid-based polymers.
Bicyclic heteroquinoids can be traced back to diketopyrrolopyrrole derivatives. The diketopyrrolopyrrole family has emerged as extensively studied building blocks for organic electronics. The representative pyrrolo[3,4-c]pyrrole-1,4-dione (DPP) is used to tactically construct polymer semiconductors for photovoltaics and transistors. By analogy to the widely employed DPP units, the structural isomer, isoDPP, was then developed to expand the chemical space. Diphenyl isoDPP is a bislactam derivative of pulvinic acid, a natural pigment found in lichens. There are three different routes for obtaining substituted isoDPP. The amidation of pulvinic acid can afford diphenyl isoDPP under harsh conditions.65 Unfortunately, this approach is not viable for scale-up. Another one-pot synthesis of substituted N-aryl isoDPP was devised.66 Diaryloxalimide dichloride was treated with an aryl ester enolate to afford the condensation product isoDPP, accompanied by a pyrrolidenone byproduct due to the two-fold cyclization that forms enamine tautomers. Notably, this condensation reaction serves as a useful handle for isoDPP on the premise that N-aryl substrates were used. If an N,N′-dialkyl intermediate en route to the alkylated isoDPP was used, the inevitable tautomerism due to the presence of α-H led to poor yields, which renders this route futile. The most efficient route to alkylated isoDPP involves stepwise cyclization to forge lactam rings. Lu et al. synthesized a variety of alkylated dithienyl isoDPP monomers (isoDPPTR) via this route, and used them to construct semiconducting polymers for use in field-effect transistors and organic bulk-heterojunction photovoltaic devices.67 They compared the molecular structures of isoDPPTR and conventional DPP using theoretical methods and single-crystal analysis. The results indicated that isoDPPTR exhibited more pronounced bond-length alternation, a larger HOMO–LUMO gap, and a different conformational preference. The difference in the structures of DPP and isoDPP is an analogy to their isoelectronic naphthoquinone compounds: 1,5-naphthoquinone (isoelectronic to DPP) has a smaller bond length alternation and a narrower HOMO–LUMO gap than that of 2,6-naphthoquinone (isoelectronic to isoDPP).68 Another key aspect to note is that conformational control plays a critical role in facilitating charge transport properties. DPP adopts a hydrogen-bond predominant, planar conformation, which limits the rotational disorder of the backbone,45 whereas isoDPPTR consists of non-planar thiophene-isoDPP subunits with a calculated dihedral angle of 24°. The single-crystal analysis of methyl isoDPP indicates the coexistence of mixed syn–anti conformers with either O⋯S or O⋯H attractions, thus implying potential conformational disorder in the corresponding polymer backbone. All polymers based on isoDPP exhibited hole transport behaviors. A hole mobility of 3.4 × 10−2 cm2 V−1 s−1 and a power conversion efficiency of 5.1% were obtained for the silolodithiophene-isoDPP copolymer P18 (Fig. 8). Despite the lower opto-electronic performances compared with those of DPP-based counterparts from a practicality standpoint, the intriguing structural details provide new insights into the diversification of bislactam-containing semiconductors.
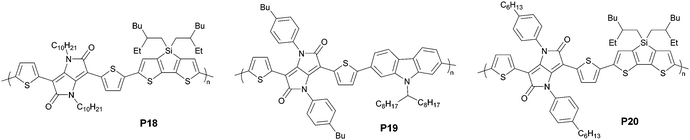 |
| Fig. 8 Typical isoDPP-based polymers used in transistors. | |
N-Aryl isoDPP was also extensively studied because of its synthetic accessibility in a one-pot, two-fold cyclization step.69–71 These materials (e.g., P19) showed moderate-to-low charge transport properties and fluorescence quantum yields. The N-aryl substituents are responsible for the variation due to the sizeable torsional angles between the aryl side chain and the backbone, thus leading to reduced π-stacking tendency. Zhang and co-workers further probed into this system through the thionation of isoDPP.72 The thionated building block, isoDTPP, gave a red-shifted absorption profile and a substantially reduced HOMO–LUMO gap, as evidenced by other thionated compounds.73 The empty 3d orbitals of sulfur atoms can accommodate π-electrons, thereby showing enforced electron affinity of the resultant molecule compared with that of the oxygen-containing counterpart. Moreover, the introduced S⋯S interactions promise intra- and inter-molecular ordering. The isoDTPP-based polymer P20 showed ambipolar transport behaviors with hole and electron mobilities of 0.49 and 0.26 cm2 V−1 s−1, respectively. This example illustrates the effect of thionation in controlling the charge injection process for heteroquinone compounds and provides a new method to enrich the semiconductor library. The substituent effect is noteworthy. Aside from thienyl-substituted monomers, isoDPP with phenyl-substituted at 3,6-positions was also exploited to gain insight into the physicochemical properties. These phenyl-substituted polymers showed narrow absorption and a large optical gap because of the large torsional angle, leading to a reduced effective conjugation length.70 Such phenomena pervade the literature, as exemplified by phenyl-DPP based polymers.74
By analogy to azaquinoid, thienoquinod serves as an electron acceptor for polymers. Tetracyano–thienoquinodimethane is a representative of thienoquioinds due to its electronic structure similar to that of 7,7,8,8-tetracyanoquinodimethane (TCNQ), a classical electron acceptor used in charge-transfer salts. In 1974, Gronowitz and co-workers reported the first cyanated thienoquinoid preparation through treatment with tetracyanoethylene oxide.75 This class of materials was then extensively studied from the late 1980s to the early 2010s for applications in n-type semiconductors.76 The deep-lying LUMO energy levels of these materials avoid oxygen and water doping such that air-stability of the device can be achieved. Notably, such deep-lying LUMO energy levels are ubiquitous for other thienoquinoidal compounds and can be inherited by the polymers. To this end, thienoquinoid-containing polymers are becoming cutting-edge research areas for n-type semiconductors.
A thieno[3,2-b]thiophene-2,5-dione (TTD)-based polymer was reported for the first time by Osaka and co-workers.77 This structure features a thiolactone-fused butadiene fragment that is highly polarized. The preparation of TTD involves the borylation of thienothiophene, followed by oxidative carbon-boron bond cleavage and in situ quinoidization. Considering the absence of attaching sites for the solubilizing groups, TTD requires the installation of alkylated substituents such as 2-octyldecyl thiophene. The authors synthesized brominated TTD monomers in four steps. An individual TTD unit has poor solubility that likely hinders coupling at later stages; thus, the alkylated coupling partner was introduced first to ensure processability. Notably, protection of the α-H on substituted thiophenes with trimethylsilyl groups is required to avoid side reactions in the borylation and oxidation steps. Next, Ir-catalyzed direct borylation proceeded in good yields and the product was treated with oxone to afford dithienyl TTD. The final step was the facile conversion of the trimethylsilyl protection groups into bromide. When this monomer was coupled with bithiophene, the delivered polymers showed narrow gaps with estimated HOMO and LUMO energy levels of −5.3 and −4.1 eV, respectively. These frontier molecular orbital energy levels enable ambipolar charge transport. Devices based on the 2-decyltetradecyl TTD polymer P21 exhibited a high hole mobility of 1.38 cm2 V−1 s−1 with a bottom-gate-top-contact configuration and hole/electron mobilities of 0.12/0.20 cm2 V−1 s−1 with a top-gate-bottom-contact configuration (Fig. 9). This work opens up the opportunity of use of thienoquinoid-containing semiconducting polymers for ambipolar transistor applications.
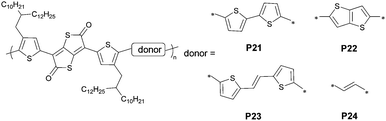 |
| Fig. 9 TTD-based polymers used in transistors. | |
In pursuit of developing an improved route to obtain TTD in terms of the overall yield and step count, the authors expedited the synthesis without resorting to protecting group chemistry.78 The chemoselectivity of the key intermediate 3,6-bis(alkylthienyl) thienothiophene indicates that the lithiation proceeds exclusively at the central thienothiophene core. To this end, they synthesized the resultant boronic acid using lithium tetramethylpiperidide and subjected the product to the following oxidation using hydrogen peroxide. This selectivity is due to the directing effect of sulfur's lone electron pairs on flanking thiophenes, and is also affected by the steric effects of the 5-position being hindered for lithiation. Thus, using a bulky lithium tetramethylpiperidide promotes the conversion. The overall yield of 42% in four steps offers access to the extensive exploration of TTD-based polymer semiconductors. The authors screened the monomer scope and demonstrated the influence of the donor moiety on the ambipolar charge transport properties. Four donor linkages, including vinylene, thienothiophene, bithiophene, and dithienylethene (P21–P24), were studied, showing that the ratio of the electron mobility to the hole mobility reduced with the lengthening of the donor conjugation. Polymers with thiophene derivative moieties showed mobilities greater than 0.1 cm2 V−1 s−1 and were further used in constructing complementary inverters. The synthetic scalability, electronic tunability, and high crystallinity of TTD-based polymers enable versatile functionalities in electronic applications.
3.2.2 Tricyclic and tetracyclic heteroquinoid-based polymers.
Studies on heteroquinoidal homologues by tactical conjugation extension underpin the development of narrow-gap semiconductors. From a theoretical point of view, introducing fused rings or vinylene linkages into heteroquinoids leads to an elevated HOMO energy level and a lowered LUMO energy level, both of which streamline the tuning of molecular electronic structures. Thanks to modern computational tools, one can predict the change by structural homologation using theoretical screening and can find the optimal target with potentially efficient injection of holes, electrons, or both. Perhaps the most daunting step during the design is the invention of the synthetic route. The lack of chemical feedstocks or mature synthetic methodology impedes the structural diversification, giving rise to only a few candidates for further evaluation. Nevertheless, these heteroquinoid derivatives hold great promises for use in electronics.
Benzodifuranone, which is a red to blue dye in textiles, has been developed over thirty years.79 Its aromatic form is a famous electron-deficiency enhancer for oxindole-based electron acceptors, providing a variety of air-stable n-channel polymer semiconductors such as bis(2-oxoindolin-3-ylidene)benzodifurandione derivatives.80,81 Unlike the aromatic linkage, quinoidal benzodifuranone is directly connected to the substituents via single bonds, resulting in a typical alternating aromatics-quinoids motif. The optical and electrochemical properties of this colorant based low-gap material was heavily studied in 2011 by Zhang and co-workers;82,83 however, their charge transport properties have seldom been studied.84 If nitrogen atoms are introduced to replace the oxygen atoms, benzodipyrrolidone (BPD) emerges as a new quinoidal chromophore with a quinodimethane substructure.85 This compound was synthesized through the condensation of p-phenylenediamine with mandelic acid derivatives, followed by a ring-closure and oxidation sequence (Fig. 10). BPD is readily alkylated under basic conditions to afford the electron-withdrawing monomer.86 The charge transport properties depended on the comonomers, giving unipolar electron transport to ambipolar transport behaviors with mobilities of the order of 10−3 cm2 V−1 s−1 (P25 and P26, Fig. 11). The relatively low mobility is likely ascribed to the torsional angle of 38° between the flanking phenyl groups and BPD being, as uncovered by the single-crystal analysis. The further optimization of the side chains and comonomers (P27 and P28) led to an increased hole mobility of up to 0.066 cm2 V−1 s−1; however, the performance is not competitive to those of other quinoid-based materials.87–89 To improve the semiconducting performance, the torsional disorder should be suppressed.
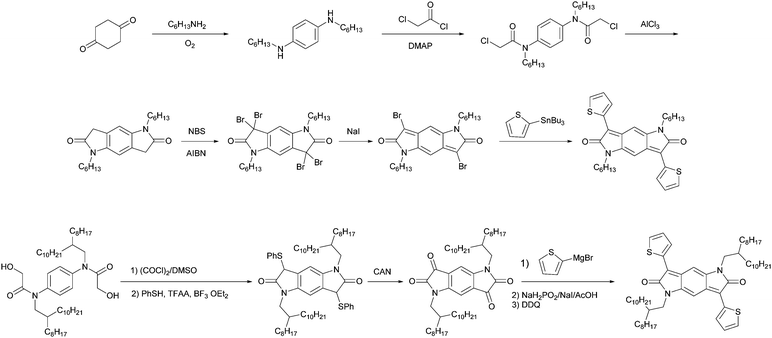 |
| Fig. 10 Two synthetic routes to obtain dithienyl benzodipyrroledione. | |
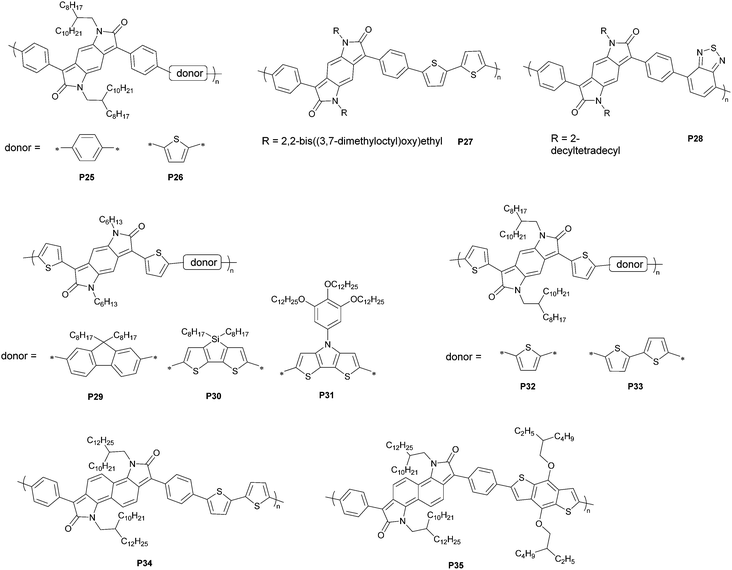 |
| Fig. 11 Benzodipyrrolidone and naphthodipyrrolidone-based semiconducting polymers. | |
Structural modification by introducing other less bulky substituents likely ameliorates the steric hindrance. Dithienylbenzodipyrrolidone (BPT) emerged as an alternative to diphenyl counterparts as the electron acceptor moiety in polymers. Because the condensation of thienyl glycolic acid with p-phenylenediamine gave an insoluble mixture that cannot be isolated, a newly designed synthetic route to BPT is required. Such a procedure differs in literatures. Cui and co-workers reported the synthesis of dialkyl dibromobenzodipyrrolidone, which was readily derived to deliver thienyl-substituted monomers.90 The route involved the condensation of 1,4-cyclohexanedione with hexylamine, amidation by chloroacetyl chloride, AlCl3-catalyzed intramolecular ring-closure, bromination with N-bromosuccinimide, and reductive debromination with sodium iodide. Thienyl flanks were installed using the Stille coupling reaction and the product was brominated to afford the monomer. When coupled with fluorene derivatives, BPT-based polymers (P29–P31) exhibited estimated LUMO energy levels as low as −3.80 eV, a decreased interring dihedral angle of 12.7°, and enhanced π–π interactions, rendering it a promising acceptor moiety used in solar cells and n-channel transistors. Rumer and co-workers developed another methodology involving a Pummerer-type cyclization method,91 a ceric(IV) ammonium nitrate-mediated oxidation, a Grignard-type addition, and subsequent redox reactions for quinoidization.92 The monomer was afforded by bromination, and copolymerized with thiophene and bithiophene donors. Hole and electron mobilities of 0.21 and 0.10 cm2 V−1 s−1 were achieved for the BPT–thiophene copolymer (P32)-based devices, respectively, whereas the BPT–bithophene copolymer P33 showed lower hole and electron mobilities of 0.08 and 0.01 cm2 V−1 s−1, respectively. This study illustrates the enhanced planarity of benzodipyrrolidone, which facilitates the charge transport behaviors.
Further extension of the quinoid framework leads to the exploration of the naphthodipyrrolidone (NPD)-based materials. By the exchange of benzoquinoid with naphthoquinoid, a narrower gap is expected to tune the energy levels, along with an altered geometric scaffold for reduced backbone curvature. Zhang and co-workers reported a route proceeding through an amidation–cyclization sequence.93 The resultant naphthodipyrrolidone-thiophene copolymer exhibited estimated LUMO and HOMO energy levels of −3.96 and −5.37 eV, respectively, implying its potential in optoelectronic applications. This example also showcases the effect of quinoid extension such that the dihedral angle of 24.9° between the phenyl flanks and the core structure is smaller than that in benzodipyrrolidone molecules (38°), and a shortened π-stacking distance of 3.38 Å further enhances the intermolecular interactions. In-depth exploitation of the substrates indicates the applications of naphthodipyrrolidone-based polymers in dyes, photovoltaics, or field-effect transistors.94–97 Among these works, an electron mobility of 0.67 cm2 V−1 s−1 (0.283 cm2 V−1 s−1 if the two-point method is used) was achieved for the naphthodipyrrolidone-bithiophene copolymer (P34)-based devices, which supports the hypothesis that naphthodipyrrolidone-based materials facilitate electron transport.96 In addition, the lower hole (0.021 cm2 V−1 s−1) and electron (0.227 cm2 V−1 s−1) mobilities for the naphthodipyrrolidone-benzodithiophene copolymer (P35) imply the importance of electronic and morphological attributes in monomer screening.
In contrast to the π-extended bislactam-based quinoids, thienoquinoids with an inserted vinylene linkage or a fused benzoquinoid require substituents with solubilizing side chains to ensure the solubility of monomers. This different attachment pattern leads to a distinct synthetic strategy for π-extended thienoquinoids (Fig. 12). The molecular scaffold was first forged and then converted to quinoid via oxidation reactions. The authors followed the optimized route via a coupling-borylation-oxidation sequence, and afforded 2,2′-bithiophene-5,5′-dione (BTD)- and benzo[1,2-b:4,5-b′]dithiophene-2,6-dione (BDTD)-based polymers.43 Notably, the BTD monomer containing a vinylene linker has two isomers, which are responsible for the visible-light-triggered reversible isomerization. The presence of isomers introduces disorders that possibly play a detrimental role in charge transport. The LUMO energy levels of these thienoquinoid-based polymers are lowered with the extension of quinoid conjugation, thus leading to a trend towards n-channel over p-channel transport behaviors. The ratio of electron mobility to hole mobility increased from 0.47 for the TTD-based polymer P36 to 1.00 for the BTD-based polymer P37 and finally to 1.17 for the BDTD-based polymer P38. A maximum electron mobility of 0.35 cm2 V−1 s−1 was obtained for P38. This example explains that the tuning of thienoquinoid conjugation effectively controls the HOMO–LUMO gap and charge transport properties. A narrow gap of less than 1 eV inspires the generation of materials with high NIR activity and visible transparency for use in optoelectronic devices.76 This class of π-extended thienoquinoid building blocks also includes recently developed dithienyl acenedithiophenediones.98 An additional fused ring can narrow the HOMO–LUMO gap by approximately 0.4 eV according to theoretical modeling (Fig. 13). The molecular orbital stabilization of these ladder-type heteroquinoids also corresponds to the fusion pattern that a geometrically linear molecule provides more stabilized frontier orbitals than a curved one, as an intuitive manifestation of enhanced effective conjugation. Such features promise to make new connections in materials chemistry.
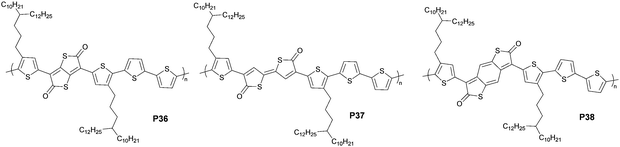 |
| Fig. 12 Thienoquinoid-based polymers with extended conjugation. | |
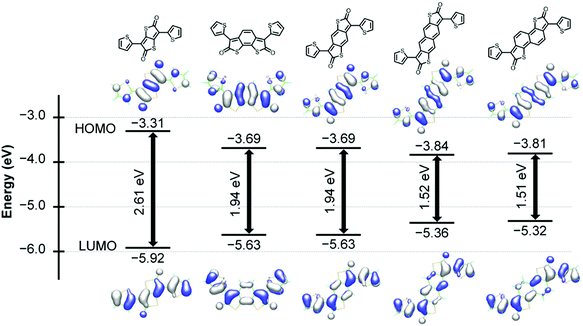 |
| Fig. 13 Density functional theory-calculated HOMO and LUMO energy levels of thienoquinoid structures. Reproduced with permission from ref. 98. Copyright 2017 Wiley-VCH. | |
3.2.3 Comparison of diverse heteroquinoid structures.
As a complement to conventional benzoquinone, heteroquinone features enhanced molecular polarization and structural diversity. Browsing the increasing enriched library of these materials, one can infer several important paradigms for the in silico design of new polymer semiconductors. First, a five-membered-ring heterquinone in lieu of a six-membered-ring benzoquinone substantially changes the main-chain geometry. The judicious incorporation of a centrosymmetric heteroquinone acceptor can favorably adjust the overall backbone linearity, which affects the persistence length, chain rigidity, absorption, and aggregation behavior.99 Second, the electronic effects by the introduction of heteroatoms effectively tune the frontier orbital energetics because either a nitrogen or a sulfur atom carries unshared electron pairs that conjugate into the π-systems. This feature leads to decreased electron-deficiency in the quinoid core, which is accompanied by intensive polarization that narrows the energy gap. These electronic structures are predictable using modern theoretical modeling. Third, structural isomers of quinone-containing building blocks offer potential chances to explore the effects of side-chain substituent patterns, electronic structures, and conformational differences. The inspiring comparison between DPP and isoDPP exemplified this rationale. We envisaged that heteroquinoids can stimulate the development of high-performance polymer semiconductors in the near future.
3.3 Semiconducting polymers based on pyrazinoquinoids
To afford an n-channel material, the limitations of many building blocks for p-dominant semiconductors necessitate strategies towards the use of more electron-deficient units. A representative method to lower the energy levels of the building blocks has been the introduction of electron-negative atoms such as pyridinic nitrogen atoms. By exchanging a methine group for a pyridinic nitrogen, the electronegativity of the sp2-N atoms dictates the lowered electron density in the scaffold. Meanwhile, the unshared electron pair provides more potential sites for constructing non-covalent bonds and relieves the steric interactions in some cases. In terms of small-molecule semiconductors, N-heteroacenes function as structural variants for the state-of-the-art acene materials, allowing in-depth studies focusing on the effect of N-substitution.100–102 As a symmetric heterocyclic alternative to benzoquinoid, pyrazinoquinoid has two pyridinic nitrogen atoms embedded in the molecular skeleton (Fig. 14). Their electronic structures are therefore akin to those of benzoquinoid analogues. Motivated by these precedents, Hong and co-workers developed a series of dipyrrolo[2,3-b:2′,3′-e]pyrazine-2,6-dione (PzDP)-based polymers, and studied the ambipolar field-effect performance arising from the strong electron-withdrawing tendency.88 The synthesis of the monomer began with the condensation reaction between ethyl-2-(4-bromophenyl)-2-oxoacetate and 1,4-diacetylpiperazine-2,5-dione to afford a mixture of cis–trans isomers. The intermediate was then treated with formamide to forge the dipyrrolopyrazine framework. They used a 2,2-bis((3,7-dimethyloctyl)oxy)ethyl side chain to overcome the strong self-aggregation of PzDP-based polymers. As a testament to the solubility improvement, this acetal-type side chain had been installed in the benzoquinoid counterparts.87 Two polymers were obtained using the Stille coupling reaction with thiophene or bithiophene as the donor. Both polymers showed typical narrow gaps with HOMO and LUMO energy levels suitable for ambipolar transport (Fig. 15). Specifically, the thiophene-PzDP copolymer P39 exhibited p-dominant transport behavior with a maximum hole mobility of 0.066 cm2 V−1 s−1 and the thienothiophene-PzDP copolymer P40-based transistor showed hole and electron mobilities of 0.053 and 0.021 cm2 V−1 s−1, respectively. Using the PzDP monomer as the starting material, the authors developed cyano-substituted unipolar semiconductors with the highest electron mobility of 0.16 cm2 V−1 s−1, further demonstrating the feasibility of PzDP-based n-channel materials.
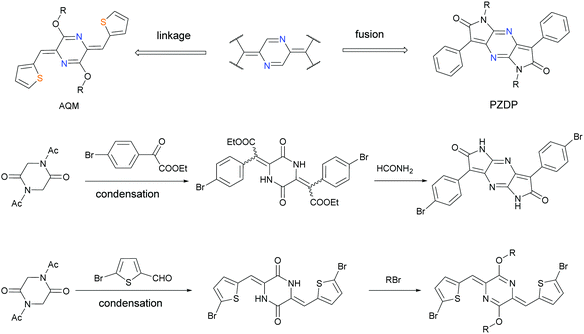 |
| Fig. 14 Chemical structures and syntheses of pyrazinoquinoid building blocks. | |
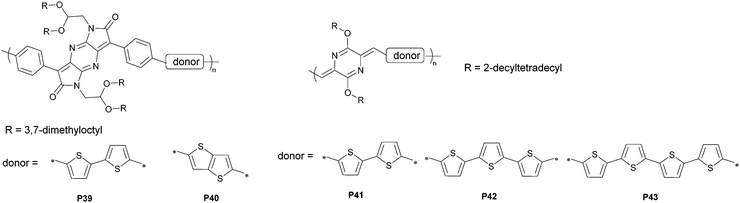 |
| Fig. 15 Pyrazinoquinoid-based semiconducting polymers. | |
Insights into the direct installation of a para-quinodimethane subunit can effectively uncover the underpinnings of such quinoidal polymers. When a quinoid structure is coupled with an aromatic unit, the bond length alternation is minimized owing to the resonance effect, leading to a lowered gap. While chemically unstable benzoquinoids have a limited substrate scope for derivatization, pyrazinoquinoids are readily accessible because of the nitrogen atoms that allow the use of a series of precursor settings. Liu and co-workers provided deep insights into this system using p-azaquinodimethane (AQM)-based polymers (P41–P43).103 They introduced nitrogen atoms to stabilize the π-extended quinodimethanes in the form of diarylidene-dialkoxydihydropyrazines, and systematically studied their optical and electronic properties. The condensation between 1,4-diacetyl-2,5-diketopiperazine and 2-formylthiophene afforded diarylidene piperazinedione, accompanied by the in situ cleavage of the acetyl-protecting groups. O-Alkylation proceeds predominantly under basic conditions because the formation of the metalated bis-lactim tautomer is thermodynamically preferable. This phenomenon is rational considering the energetic stabilization in the conjugated bis-lactim intermediate. This building block features an exclusive Z-configuration with intramolecular S⋯N interactions. The monomer was then used to build polymer chains with different lengths of the donor units. Bithiophene-, terthiophene-, and quaterthiophene-linked copolymers were obtained to illustrate the optical and electrochemical properties. As the length of the donor moiety increases, the optical gap also increases, with a slightly lowered HOMO energy level and a similar LUMO energy level estimated from cyclic voltammetry. This trend is typical for quinoids-aromatics alternating polymers but is distinctly different from that for all-aromatic conjugated systems. These AQM-based polymers showed ambipolar transport behaviors with the highest carrier mobility of 0.54 cm2 V−1 s−1 (P42). The direct introduction of azaquinodimethane into the polymer backbone shows the inherent electronic structures of quinoidal building blocks, thus providing new insights into quinoid-based materials for next-generation semiconductors. To improve the charge transport properties, the authors studied the monomer scope with different side chains and backbone structures.104 The authors observed a typical odd–even trend for branched side-chain spacers and a trend towards edge-on packing as the conjugation length increases. These effects play synergistic roles in thin-film crystallinity and chain orientation. A hole mobility of up to 1.05 cm2 V−1 s−1 was achieved after optimization. Furthermore, ionic AQM compounds were synthesized as active materials in photothermal therapy. The absence of fluorescence in ionic AQM-based polymers indicated non-radiative de-excitation pathways upon laser exposure. Follow-up antibacterial tests showed a 95% reduction in the bacterial colony count in the solution of an ionic AQM polymer upon laser irradiation for 5 min. This result is a proof of concept for these materials as promising candidates in photothermal therapy.105
The pyrazinoquinoid compound family is still in its infancy, whether they are constructed in a fused or in a bridged manner. This lack of understanding is correlated with the multiple roles of nitrogen atoms in electronic and steric effects, and partly with the limited scope of sp2-hybridized nitrogen-containing building blocks. From the viewpoint of their structural aspects, these N-heteroquinoids effectively tune the electronic effects and introduce modifications in the conjugation pathway by changing the C
C double bonds with short C
N bonds; however, whether this modification is positive for promoting charge transport is under debate. From a synthetic perspective, nitrogen-containing precursors, such as amides, can provide reactive sites for the installation of other groups through condensation reactions. This synthetic advantage, however, cannot always neutralize the accompanied shortages. Typical conceivable problems for N-heteroaromatic materials include their long-term chemical stability against oxidants or reductants, and their potential binding capability as competent ligands for metal catalysts to impede cross-coupling polymerization. Precedent studies on small molecules indicate that N-heteroaromatic compounds are sensitive to redox conditions under which variable oxidation forms of the molecules can be detected.106 Naturally-occurring compounds are replete with unstable N-containing molecules that readily undergo degradation upon radical-mediated photooxidation. The impurities involved in these processes can greatly affect the final electrical performances by introducing defects in the captured carriers.107,108 Failed attempts to incorporate pyridinic N-containing quinoids or long-term stability studies are generally not included in the bibliography; thus, one cannot obtain a comprehensive survey of such effects. Furthermore, the synergistic union of quinoids and pyridinic nitrogen atoms, both of which are underdeveloped, might add more complexities in material preparation and subsequent device optimization.
4. Conclusions and outlook
The quinoidal polymers shown in this review, while insightful, are by no means comprehensive. Browsing the typical quinoidal polymers, one can conclude that the energy gap engineering is their most valuable character. The inherently low energy gap of the quinoidal moiety in the polymer backbone provides access to a sufficiently low-lying LUMO energy level for electron transport and a suitable HOMO energy level to retain hole transport. Correspondingly, the optical characteristics of quinoidal polymers are also affected, showing a relatively broad absorption band that covers even a part of the near infrared region. When a quinoidal monomer is coupled with an aromatic moiety, the absorption profile shows a blue shift with the conjugation extension of the aromatic units, in contrast to those of all-aromatics polymers.88,103 This phenomenon is rationalized by the hybridization of acceptors (quinoids) and donors (aromatics) in a co-repeating unit. The low-lying LUMO of the quinoid moiety contributes predominantly to the hybridized LUMO; extending the aromatic donor moiety dilutes such a contribution, leading to an increased energy gap. Moreover, in-depth studies regarding diketopyrrolopyrrole-based polymers suggest that the low-energy absorption band originates from charge redistribution within the diketopyrrolopyrrole unit and vibronic coupling during aggregation.109 This explanation is likely generic for quinoidal polymers, indicating that quinoid-containing polymers behave differently from the conventional all-aromatic donor–acceptor polymers. The nature of narrow gaps is inherited from the innate narrow gap of the quinoid moiety itself, rather than as a major contribution from the molecular-orbital hybridization of both the donor and the acceptor in the case of conventional donor–acceptor polymers. Based on these features, these quinoidal structures provide a new chemical space to explore the electronic properties of semiconducting polymers and open a new avenue to a complementary mode of frontier orbital tuning, thus boding well for both academic and industrial interests. Computational tools may offer insight a priori into such molecular descriptors rather than merely post-rationalizing the guidelines.
Although studies on quinoidal polymers serve as an inspiration for structural evolution in semiconductor design, there are still many challenges in unveiling the innate structure–property relationship. Developing a new class of high-performance semiconductors is a multidisciplinary area in which the procedure commences with molecular design and organic synthesis, followed by physicochemical characterization, and finishes with empirical trial-and-error device optimization. In terms of synthesis, despite the landmark development of diketopyrrolopyrrole (isoelectronic to 1,5-naphthoquinone), new quinoidal building blocks require target-oriented synthesis that are confronted with the need to obtain unprecedented fused-ring aromatic heterocycles in at least gram-scale quantities. There have been many examples due to the lack of scalable synthetic methodologies for the construction of structurally conceivable quinoid-embedded molecules, including pyrrolo[3,4-f]isoindole-1,5-dione (a fused diketopyrrolopyrrole congener).110 In addition, the development of a synthetic route is faced with the problem of unknown solubility and chemoselectivity (e.g., the competition between N- and O-alkylation for lactam alkylation), both of which can be varied depending on the electronic structures of the intermediates. Stereocontrol is another concern for quinoids, especially for those with a connectivity pattern that contains double-bond linkages. This is important to reduce the kinetic or thermodynamic disorder of the backbone and to prevent unfavorable transformation under radiation. The diversity-oriented preparation of polymer structures, especially the easy-to-functionalize heteroquinoids, is also necessary in the design step and late-stage optimization, by achieving a high level of sophistication through substitution. In terms of characterization, a direct observation of the local polymer chains and stacks can provide more precise insights over the global statistic information estimated from X-ray diffraction.49 Modern techniques are being developed to meet the requirement of comprehensive studies but are still limited to empirical understandings.
Systematic studies on how the high performance is achieved sometimes give controversial conclusions for the lack of physical underpinnings. Many influencing parameters, both important as well as trivial, may generate unmet problems in performance control. A modest change in the quinoidal structure can result in substantial differences in the chemical and electrical properties. All of these distinctive structural factors involved are difficult, if not impossible, to deconvolute in the current laboratory setting. It is worth noting that an increased molecular complexity does not consistently lead to high performance. Examples include the π-extension of the quinoidal moiety. Although extending the conjugation likely affords a lowered energy gap, the side-chain attaching sites become far away from each other, thus affecting the interdigitation of contiguous chains. The linearity of the backbone is typically altered by the insertion of quinoidal subunits, giving rise to an uncontrollable packing pattern that is crucial for intermolecular charge transport. Methods to confer an enthalpic advantage to intramolecular conformational and configurational control, such as the tactical S,S-oxidation protocols, can also affect such intermolecular packing, molecular symmetry, and backbone linearity. Structural modification requires a balancing of all the potential factors such that it can propel the optimization of the device performance to an applicable level.
In the pragmatic aspects, the applications of quinoidal semiconducting polymers are now limited in conventional mobility tests. These materials have a great potential for other applications, including the emerging stretchable electronics, bioelectronics, thermoelectrics, and spintronics. In particular, heteroquinoid-containing materials are structural analogs that are commonly used in diketopyrrolopyrrole- or isoindigo-based semiconductors, thus showing promise in these applications. Through either strain-accommodating engineering or blending technique, quinoidal semiconducting polymers could readily tolerate the applied stress.111 In addition, their structural tunability allows the introduction of softer building blocks, including bulky alkyl chains, hydrogen-bonded moieties, and a small fraction of non-conjugated linkers, thus further enhancing the competitiveness in related uses. Considering the growing potential of quinoidal polymers, it is anticipated that the quinoidization of known building blocks would be a creative tool in the semiconductor-construction toolbox.
Conflicts of interest
There are no conflicts to declare.
Acknowledgements
We are thankful for the financial support from the National Natural Science Foundation of China (Grants 21474116) and the National Key Research and Development Program of China (2017YFA0204703).
Notes and references
- M. L. Hammock, A. Chortos, B. C. Tee, J. B. Tok and Z. Bao, 25th anniversary article: the evolution of electronic skin (e-skin): a brief history, design considerations, and recent progress, Adv. Mater., 2013, 25, 5997–6038 CrossRef CAS.
- K. Fukuda and T. Someya, Recent progress in the development of printed thin-film transistors and circuits with high-resolution printing technology, Adv. Mater., 2017, 29, 1602736 CrossRef.
- H. Sirringhaus, 25th anniversary article: Organic field-effect transistors: The path beyond amorphous silicon, Adv. Mater., 2014, 26, 1319–1335 CrossRef CAS.
- J. A. Rogers, T. Someya and Y. Huang, Materials and mechanics for stretchable electronics, Science, 2010, 327, 1603–1607 CrossRef CAS.
- S. Wang, J. Y. Oh, J. Xu, H. Tran and Z. Bao, Skin-inspired electronics: An emerging paradigm, Acc. Chem. Res., 2018, 51, 1033–1045 CrossRef CAS.
- B. Wang, W. Huang, L. Chi, M. Al-Hashimi, T. J. Marks and A. Facchetti, High-k gate dielectrics for emerging flexible and stretchable electronics, Chem. Rev., 2018, 118, 5690–5754 CrossRef CAS.
- Y. S. Rim, S. H. Bae, H. Chen, N. De Marco and Y. Yang, Recent progress in materials and devices toward printable and flexible sensors, Adv. Mater., 2016, 28, 4415–4440 CrossRef CAS.
- Y. Liu, K. He, G. Chen, W. R. Leow and X. Chen, Nature-inspired structural materials for flexible electronic devices, Chem. Rev., 2017, 117, 12893–12941 CrossRef CAS.
- J. Xu, S. Wang, G. N. Wang, C. Zhu, S. Luo, L. Jin, X. Gu, S. Chen, V. R. Feig, J. W. To, S. Rondeau-Gagne, J. Park, B. C. Schroeder, C. Lu, J. Y. Oh, Y. Wang, Y. H. Kim, H. Yan, R. Sinclair, D. Zhou, G. Xue, B. Murmann, C. Linder, W. Cai, J. B. Tok, J. W. Chung and Z. Bao, Highly stretchable polymer semiconductor films through the nanoconfinement effect, Science, 2017, 355, 59–64 CrossRef CAS.
- J. Y. Oh, S. Rondeau-Gagne, Y. C. Chiu, A. Chortos, F. Lissel, G. N. Wang, B. C. Schroeder, T. Kurosawa, J. Lopez, T. Katsumata, J. Xu, C. Zhu, X. Gu, W. G. Bae, Y. Kim, L. Jin, J. W. Chung, J. B. Tok and Z. Bao, Intrinsically stretchable and healable semiconducting polymer for organic transistors, Nature, 2016, 539, 411–415 CrossRef CAS.
- Q. H. Wu, R. J. Li, W. Hong, H. X. Li, X. K. Gao and D. B. Zhu, Dicyanomethylene-substituted fused tetrathienoquinoid for high-performance, ambient-stable, solution-processable n-channel organic thin-film transistors, Chem. Mater., 2011, 23, 3138–3140 CrossRef CAS.
- Y. Shi, L. Jiang, J. Liu, Z. Tu, Y. Hu, Q. Wu, Y. Yi, E. Gann, C. R. McNeill, H. Li, W. Hu, D. Zhu and H. Sirringhaus, Bottom-up growth of n-type monolayer molecular crystals on polymeric substrate for optoelectronic device applications, Nat. Commun., 2018, 9, 2933 CrossRef.
- C. K. Frederickson, B. D. Rose and M. M. Haley, Explorations of the indenofluorenes and expanded quinoidal analogues, Acc. Chem. Res., 2017, 50, 977–987 CrossRef CAS.
- Y. Xiong, J. Tao, R. Wang, X. Qiao, X. Yang, D. Wang, H. Wu and H. Li, A furan-thiophene-based quinoidal compound: A new class of solution-processable high-performance n-type organic semiconductor, Adv. Mater., 2016, 28, 5949–5953 CrossRef CAS.
- N. Berube, J. Gaudreau and M. Cote, Low band gap polymers design approach based on a mix of aromatic and quinoid structures, Macromolecules, 2013, 46, 6873–6880 CrossRef CAS.
- T. Zheng, L. Lu, N. E. Jackson, S. J. Lou, L. X. Chen and L. Yu, Roles of quinoidal character and regioregularity in determining the optoelectronic and photovoltaic properties of conjugated copolymers, Macromolecules, 2014, 47, 6252–6259 CrossRef CAS.
- Q. T. Zhang and J. M. Tour, Low optical bandgap polythiophenes by an alternating donor/acceptor repeat unit strategy, J. Am. Chem. Soc., 1997, 119, 5065–5066 CrossRef CAS.
- J. D. Douglas, G. Griffini, T. W. Holcombe, E. P. Young, O. P. Lee, M. S. Chen and J. M. J. Fréchet, Functionalized isothianaphthene monomers that promote quinoidal character in donor–acceptor copolymers for organic photovoltaics, Macromolecules, 2012, 45, 4069–4074 CrossRef CAS.
- C. Zhang and X. Zhu, n-Type quinoidal oligothiophene-based semiconductors for thin-film transistors and thermoelectrics, Adv. Funct. Mater., 2020, 30, 2000765 CrossRef CAS.
- Y. Sun, Y. Guo and Y. Liu, Design and synthesis of high performance π-conjugated materials through antiaromaticity and quinoid strategy for organic field-effect transistors, Mater. Sci. Eng., R, 2019, 136, 13–26 CrossRef.
- T. Lei, J.-H. Dou and J. Pei, Influence of alkyl chain branching positions on the hole mobilities of polymer thin-film transistors, Adv. Mater., 2012, 24, 6457–6461 CrossRef CAS.
- A. Troisi and A. Shaw, Very large pi-conjugation despite strong nonplanarity: A path for designing new semiconducting polymers, J. Phys. Chem. Lett., 2016, 7, 4689–4694 CrossRef CAS.
- S. A. Mollinger, B. A. Krajina, R. Noriega, A. Salleo and A. J. Spakowitz, Percolation, tie-molecules, and the microstructural determinants of charge transport in semicrystalline conjugated polymers, ACS Macro Lett., 2015, 4, 708–712 CrossRef CAS.
- R. Noriega, A. Salleo and A. J. Spakowitz, Chain conformations dictate multiscale charge transport phenomena in disordered semiconducting polymers, Proc. Natl. Acad. Sci. U. S. A., 2013, 110, 16315–16320 CrossRef CAS.
- N. E. Jackson, L. X. Chen and M. A. Ratner, Charge transport network dynamics in molecular aggregates, Proc. Natl. Acad. Sci. U. S. A., 2016, 113, 8595–8600 CrossRef CAS.
- D. Venkateshvaran, M. Nikolka, A. Sadhanala, V. Lemaur, M. Zelazny, M. Kepa, M. Hurhangee, A. J. Kronemeijer, V. Pecunia, I. Nasrallah, I. Romanov, K. Broch, I. McCulloch, D. Emin, Y. Olivier, J. Cornil, D. Beljonne and H. Sirringhaus, Approaching disorder-free transport in high-mobility conjugated polymers, Nature, 2014, 515, 384–388 CrossRef CAS.
- Y. Olivier, D. Niedzialek, V. Lemaur, W. Pisula, K. Mullen, U. Koldemir, J. R. Reynolds, R. Lazzaroni, J. Cornil and D. Beljonne, 25th anniversary article: High-mobility hole and electron transport conjugated polymers: How structure defines function, Adv. Mater., 2014, 26, 2119–2136 CrossRef CAS.
- T. M. Swager, 50th anniversary perspective: Conducting/semiconducting conjugated polymers. A personal perspective on the past and the future, Macromolecules, 2017, 50, 4867–4886 CrossRef CAS.
- J. N. Wilson, P. M. Windscheif, U. Evans, M. L. Myrick and U. H. F. Bunz, Band gap engineering of poly(p-phenyleneethynylene)s: Cross-conjugated PPE−PPV hybrids, Macromolecules, 2002, 35, 8681–8683 CrossRef CAS.
- M. Gholami and R. R. Tykwinski, Oligomeric and polymeric systems with a cross-conjugated π-framework, Chem. Rev., 2006, 106, 4997–5027 CrossRef CAS.
- J. Huang, X. Liu, D. Gao, C. Wei, W. Zhang and G. Yu, Benzothiophene-flanked diketopyrrolopyrrole polymers: Impact of isomeric frameworks on carrier mobilities, RSC Adv., 2016, 6, 83448–83455 RSC.
- W. Zhang, Z. Mao, N. Zheng, J. Zou, L. Wang, C. Wei, J. Huang, D. Gao and G. Yu, Highly planar cross-conjugated alternating polymers with multiple conformational locks: Synthesis, characterization and their field-effect properties, J. Mater. Chem. C, 2016, 4, 9266–9275 RSC.
- V. Lemaur, L. Muccioli, C. Zannoni, D. Beljonne, R. Lazzaroni, J. Cornil and Y. Olivier, On the supramolecular packing of high electron mobility naphthalene diimide copolymers: The perfect registry of asymmetric branched alkyl side chains, Macromolecules, 2013, 46, 8171–8178 CrossRef CAS.
- T. Lei, Y. Cao, X. Zhou, Y. Peng, J. Bian and J. Pei, Systematic investigation of isoindigo-based polymeric field-effect transistors: Design strategy and impact of polymer symmetry and backbone curvature, Chem. Mater., 2012, 24, 1762–1770 CrossRef CAS.
- L. Ying, B. B. Hsu, H. Zhan, G. C. Welch, P. Zalar, L. A. Perez, E. J. Kramer, T. Q. Nguyen, A. J. Heeger, W. Y. Wong and G. C. Bazan, Regioregular pyridal[2,1,3]thiadiazole pi-conjugated copolymers, J. Am. Chem. Soc., 2011, 133, 18538–18541 CrossRef CAS.
- L. A. Perez, P. Zalar, L. Ying, K. Schmidt, M. F. Toney, T.-Q. Nguyen, G. C. Bazan and E. J. Kramer, Effect of backbone regioregularity on the structure and orientation of a donor–acceptor semiconducting copolymer, Macromolecules, 2014, 47, 1403–1410 CrossRef CAS.
- L. Ying, F. Huang and G. C. Bazan, Regioregular narrow-bandgap-conjugated polymers for plastic electronics, Nat. Commun., 2017, 8, 14047 CrossRef CAS.
- R. J. Kline, M. D. McGehee, E. N. Kadnikova, J. Liu, J. M. J. Fréchet and M. F. Toney, Dependence of regioregular poly(3-hexylthiophene) film morphology and field-effect mobility on molecular weight, Macromolecules, 2005, 38, 3312–3319 CrossRef CAS.
- A. Gasperini and K. Sivula, Effects of molecular weight on microstructure and carrier transport in a semicrystalline poly(thieno)thiophene, Macromolecules, 2013, 46, 9349–9358 CrossRef CAS.
- S. Himmelberger, K. Vandewal, Z. Fei, M. Heeney and A. Salleo, Role of molecular weight distribution on charge transport in semiconducting polymers, Macromolecules, 2014, 47, 7151–7157 CrossRef CAS.
- C. Grand, W. Zajaczkowski, N. Deb, C. K. Lo, J. L. Hernandez, D. G. Bucknall, K. Mullen, W. Pisula and J. R. Reynolds, Morphology control in films of isoindigo polymers by side-chain and molecular weight effects, ACS Appl. Mater. Interfaces, 2017, 9, 13357–13368 CrossRef CAS.
- K. Müllen, Molecular defects in organic materials, Nat. Rev. Mater., 2016, 1, 15013 CrossRef.
- K. Kawabata, M. Saito, I. Osaka and K. Takimiya, Very small bandgap pi-conjugated polymers with extended thienoquinoids, J. Am. Chem. Soc., 2016, 138, 7725–7732 CrossRef CAS.
- Z. Chen, J. Huang, D. Gao, J. Yang, W. Zhang, H. Ju and G. Yu, Highly-soluble multi-alkylated polymer semiconductors and applications in high-performance field-effect transistors, J. Mater. Chem. C, 2019, 7, 9591–9598 RSC.
- N. E. Jackson, B. M. Savoie, K. L. Kohlstedt, M. Olvera de la Cruz, G. C. Schatz, L. X. Chen and M. A. Ratner, Controlling conformations
of conjugated polymers and small molecules: The role of nonbonding interactions, J. Am. Chem. Soc., 2013, 135, 10475–10483 CrossRef CAS.
- P. Espinet and A. M. Echavarren, The mechanisms of the Stille reaction, Angew. Chem., Int. Ed., 2004, 43, 4704–4734 CAS.
- B. Carsten, F. He, H. J. Son, T. Xu and L. Yu, Stille polycondensation for synthesis of functional materials, Chem. Rev., 2011, 111, 1493–1528 CrossRef CAS.
- C. Cordovilla, C. Bartolomé, J. M. Martínez-Ilarduya and P. Espinet, The Stille reaction, 38 years later, ACS Catal., 2015, 5, 3040–3053 CrossRef CAS.
- D. A. Warr, L. M. A. Perdigão, H. Pinfold, J. Blohm, D. Stringer, A. Leventis, H. Bronstein, A. Troisi and G. Costantini, Sequencing conjugated polymers by eye, Sci. Adv., 2018, 4, eaas9543 CrossRef.
- J. Huang, S. Lu, P. A. Chen, K. Wang, Y. Y. Hu, Y. Liang, M. Wang and E. Reichmanis, Rational design of a narrow-bandgap conjugated polymer using the quinoidal thieno[3,2-b]thiophene-based building block for organic field-effect transistor applications, Macromolecules, 2019, 52, 4749–4756 CrossRef CAS.
- R. Stalder, J. Mei, K. R. Graham, L. A. Estrada and J. R. Reynolds, Isoindigo, a versatile electron-deficient unit for high-performance organic electronics, Chem. Mater., 2014, 26, 664–678 CrossRef CAS.
- T. Lei, Y. Cao, Y. Fan, C. J. Liu, S. C. Yuan and J. Pei, High-performance air-stable organic field-effect transistors: Isoindigo-based conjugated polymers, J. Am. Chem. Soc., 2011, 133, 6099–6101 CrossRef CAS.
- R. S. Ashraf, A. J. Kronemeijer, D. I. James, H. Sirringhaus and I. McCulloch, A new thiophene substituted isoindigo based copolymer for high performance ambipolar transistors, Chem. Commun., 2012, 48, 3939–3941 RSC.
- T. Minami and M. Nakano, Diradical character view of singlet fission, J. Phys. Chem. Lett., 2011, 3, 145–150 CrossRef.
- H. Hwang, D. Khim, J. M. Yun, E. Jung, S. Y. Jang, Y. H. Jang, Y. Y. Noh and D. Y. Kim, Quinoidal molecules as a new class of ambipolar semiconductor originating from amphoteric redox behavior, Adv. Funct. Mater., 2015, 25, 1146–1156 CrossRef CAS.
- H. Hwang, Y. Kim, M. Kang, M.-H. Lee, Y.-J. Heo and D.-Y. Kim, A conjugated polymer with high planarity and extended π-electron delocalization via a quinoid structure prepared by short synthetic steps, Polym. Chem., 2017, 8, 361–365 RSC.
- A. Baeyer, Untersuchungen über die gruppe des indigblaus, Ber. Dtsch. Chem. Ges., 1879, 12, 1309–1319 CrossRef.
- G. V. Tormos, K. A. Belmore and M. P. Cava, The indophenine reaction revisited - properties of a soluble dialkyl derivative, J. Am. Chem. Soc., 1993, 115, 11512–11515 CrossRef CAS.
- Y. Kim, H. Hwang, N. K. Kim, K. Hwang, J. J. Park, G. I. Shin and D. Y. Kim, pi-Conjugated polymers incorporating a novel planar quinoid building block with extended delocalization and high charge carrier mobility, Adv. Mater., 2018, 30, 1706557 CrossRef.
- Y. Deng, B. Sun, Y. He, J. Quinn, C. Guo and Y. Li, Thiophene-S,S-dioxidized indophenine: A quinoid-type building block with high electron affinity for constructing n-type polymer semiconductors with narrow band gaps, Angew. Chem., Int. Ed., 2016, 55, 3459–3462 CrossRef CAS.
- Y. Deng, J. Quinn, B. Sun, Y. He, J. Ellard and Y. Li, Thiophene-S,S-dioxidized indophenine (IDTO) based donor–acceptor polymers for n-channel organic thin film transistors, RSC Adv., 2016, 6, 34849–34854 RSC.
- K. Guo, B. T. Wu, Y. Jiang, Z. L. Wang, Z. Q. Liang, Y. N. Li, Y. F. Deng and Y. H. Geng, Synthesis of an isomerically pure thienoquinoid for unipolar n-type conjugated polymers: Effect of backbone curvature on charge transport performance, J. Mater. Chem. C, 2019, 7, 10352–10359 RSC.
- K. Hwang, M.-H. Lee, J. Kim, Y.-J. Kim, Y. Kim, H. Hwang, I.-B. Kim and D.-Y. Kim, 3,4-Ethylenedioxythiophene-based isomer-free quinoidal building block and conjugated polymers for organic field-effect transistors, Macromolecules, 2020, 53, 1977–1987 CrossRef CAS.
- Y. Sun, Y. Zhang, Y. Ran, L. Shi, Q. Zhang, J. Chen, Q. Li, Y. Guo and Y. Liu, Methoxylation of quinoidal bithiophene as single regioisomer building block for narrow-bandgap conjugated polymers and high-performance organic field-effect transistors, J. Mater. Chem. C, 2020 10.1039/d0tc02199g.
- Y. Solberg, Studies on chemistry of lichens 0.15. New synthetic nitrogen-derivatives of pulvinic acid, Z. Naturforsch., C: J. Biosci., 1977, 32, 292–293 Search PubMed.
- J. Wuckelt, M. Doring, P. Langer, H. Gorls and R. Beckert, Lactam analogues of pentalene. A new one-pot synthesis of pyrrolo[3,2-b]pyrrole-2,5-diones deriving from pulvinic acid, Tetrahedron Lett., 1997, 38, 5269–5272 CrossRef CAS.
- S. F. Lu, M. Drees, Y. Yao, D. Boudinet, H. Yan, H. L. Pan, J. Q. Wang, Y. N. Li, H. Usta and A. Facchetti, 3,6-Dithiophen-2-yl-diketopyrrolo[3,2-b]pyrrole (isoDPPT) as an acceptor building block for organic opto-electronics, Macromolecules, 2013, 46, 3895–3906 CrossRef CAS.
- K. Iijima and T. Mori, Electron transport in isodiketopyrrolopyrrole (isoDPP), Chem. Lett., 2017, 46, 357–359 CrossRef CAS.
- S. Song, S. J. Ko, H. Shin, Y. Jin, I. Kim, J. Y. Kim and H. Suh, Synthesis of the pyrrolo[3,2-b]pyrrole-based copolymer with enhanced open circuit voltage, Synth. Met., 2012, 162, 2288–2293 CrossRef CAS.
- I. Welterlich, O. Charov and B. Tieke, Deeply colored polymers containing 1,3,4,6-tetraarylpyrrolo[3,2-b]pyrrole-2,5-dione (isoDPP) units in the main chain, Macromolecules, 2012, 45, 4511–4519 CrossRef CAS.
- H. Zhang, I. Welterlich, J.-M. Neudörfl, B. Tieke, C. Yang, X. Chen and W. Yang, Synthesis and characterization of 1,3,4,6-tetraarylpyrrolo[3,2-b]-pyrrole-2,5-dione (isoDPP)-based donor–acceptor polymers with low band gap, Polym. Chem., 2013, 4, 4682–4689 RSC.
- H. C. Zhang, K. Yang, K. Zhang, Z. Z. Zhang, Q. K. Sun and W. J. Yang, Thionating iso-diketopyrrolopyrrole-based polymers: From p-type to ambipolar field effect transistors with enhanced charge mobility, Polym. Chem., 2018, 9, 1807–1814 RSC.
- A. J. Tilley, C. Guo, M. B. Miltenburg, T. B. Schon, H. Yan, Y. N. Li and D. S. Seferos, Thionation enhances the electron mobility of perylene diimide for high performance n-channel organic field effect transistors, Adv. Funct. Mater., 2015, 25, 3321–3329 CrossRef CAS.
- S.-Y. Liu, H.-Y. Li, M.-M. Shi, H. Jiang, X.-L. Hu, W.-Q. Li, L. Fu and H.-Z. Chen, Pd/c as a clean and effective heterogeneous catalyst for C–C couplings toward highly pure semiconducting polymers, Macromolecules, 2012, 45, 9004–9009 CrossRef CAS.
- S. Gronowitz and B. Uppström, On the reaction of 2, 5-dihalothiophenes with tetracyanoethylene oxide, Acta Chem. Scand., Ser. B, 1974, 28, 981–985 CrossRef.
- K. Takimiya and K. Kawabata, Thienoquinoidal system: Promising molecular architecture for optoelectronic applications, J. Synth. Org. Chem., Jpn., 2018, 76, 1176–1184 CrossRef CAS.
- I. Osaka, T. Abe, H. Mori, M. Saito, N. Takemura, T. Koganezawa and K. Takimiya, Small band gap polymers incorporating a strong acceptor, thieno[3,2-b]thiophene-2,5-dione, with p-channel and ambipolar charge transport characteristics, J. Mater. Chem. C, 2014, 2, 2307–2312 RSC.
- K. Kawabata, I. Osaka, M. Nakano, N. Takemura, T. Koganezawa and K. Takimiya, Thienothiophene-2,5-dione-based donor-acceptor polymers: Improved synthesis and influence of the donor units on ambipolar charge transport properties, Adv. Electron. Mater., 2015, 1, 1500039 CrossRef.
- O. Annen, R. Egli, R. Hasler, B. Henzi, H. Jakob and P. Matzinger, Replacement of disperse anthraquinone dyes, Rev. Prog. Color. Relat. Top., 1987, 17, 72–85 CrossRef CAS.
- T. Lei, J.-H. Dou, X.-Y. Cao, J.-Y. Wang and J. Pei, Electron-deficient poly(p-phenylene vinylene) provides electron mobility over 1 cm2 V−1 s−1 under ambient conditions, J. Am. Chem. Soc., 2013, 135, 12168–12171 CrossRef CAS.
- Z. Yan, B. Sun and Y. Li, Novel stable (3E,7E)-3,7-bis(2-oxoindolin-3-ylidene)benzo[1,2-b:4,5-b′]difuran-2,6(3H,7H)-dione based donor-acceptor polymer semiconductors for n-type organic thin film transistors, Chem. Commun., 2013, 49, 3790–3792 RSC.
- K. Zhang and B. Tieke, Low-bandgap benzodifuranone-based polymers, Macromolecules, 2011, 44, 4596–4599 CrossRef CAS.
- K. Zhang, B. Tieke, J. C. Forgie, F. Vilela and P. J. Skabara, Donor–acceptor conjugated polymers based on p- and o-benzodifuranone and thiophene derivatives: Electrochemical preparation and optical and electronic properties, Macromolecules, 2011, 45, 743–750 CrossRef.
- Z. Deng, K. Yang, L. Li, W. Bao, X. Hao, T. Ai and K. Kou, Solution processed air-stable p-channel organic crystal field-effect transistors of aminobenzodifuranone, Dyes Pigm., 2018, 151, 173–178 CrossRef CAS.
- C. W. Greenhalgh, J. L. Carey and D. F. Newton, The synthesis of quinodimethanes in the benzodifuranone and benzodipyrrolidone series, Dyes Pigm., 1980, 1, 103–120 CrossRef CAS.
- W. B. Cui, J. Yuen and F. Wudl, Benzodipyrrolidones and their polymers, Macromolecules, 2011, 44, 7869–7873 CrossRef CAS.
- W. Hong, C. Guo, Y. N. Li, Y. Zheng, C. Huang, S. F. Lu and A. Facchetti, Synthesis and thin-film transistor performance of benzodipyrrolinone and bithiophene donor-acceptor copolymers, J. Mater. Chem., 2012, 22, 22282–22289 RSC.
- W. Hong, B. Sun, C. Guo, J. Yuen, Y. Li, S. Lu, C. Huang and A. Facchetti, Dipyrrolo[2,3-b:2′,3′-e]pyrazine-2,6(1H,5H)-dione based conjugated polymers for ambipolar organic thin-film transistors, Chem. Commun., 2013, 49, 484–486 RSC.
- J. W. Rumer, S. Rossbauer, M. Planells, S. E. Watkins, T. D. Anthopoulos and I. McCulloch, Reduced roughness for improved mobility in benzodipyrrolidone-based, n-type OFETs, J. Mater. Chem. C, 2014, 2, 8822–8828 RSC.
- W. Cui and F. Wudl, Dithienylbenzodipyrrolidone: New acceptor for donor–acceptor low band gap polymers, Macromolecules, 2013, 46, 7232–7238 CrossRef CAS.
- L. H. Smith, S. C. Coote, H. F. Sneddon and D. J. Procter, Beyond the Pummerer reaction: Recent developments in thionium ion chemistry, Angew. Chem., Int. Ed., 2010, 49, 5832–5844 CrossRef CAS.
- J. W. Rumer, M. Levick, S. Y. Dai, S. Rossbauer, Z. Huang, L. Biniek, T. D. Anthopoulos, J. R. Durrant, D. J. Procter and I. McCulloch, BPTs: Thiophene-flanked benzodipyrrolidone conjugated polymers for ambipolar organic transistors, Chem. Commun., 2013, 49, 4465–4467 RSC.
- H. C. Zhang, J. M. Neudorfl and B. Tieke, 1,6-Naphthodione-based monomers and polymers, Polym. Chem., 2014, 5, 3754–3757 RSC.
- H. Zhang, S. Ying, B. Tieke, J. Zhang and W. Yang, 1,6-Naphthodipyrrolidone-based donor–acceptor polymers with low bandgap, Polymer, 2015, 60, 215–220 CrossRef CAS.
- H. C. Zhang, K. Yang, Y. M. Chen, R. Bhatta, M. Tsige, S. Z. D. Cheng and Y. Zhu, Polymers based on benzodipyrrolidone and naphthodipyrrolidone with latent hydrogen-bonding on the main chain, Macromol. Chem. Phys., 2017, 218, 1600617 CrossRef.
- H. C. Zhang, S. Zhang, Y. F. Mao, K. W. Liu, Y. M. Chen, Z. Jiang, J. Strzalka, W. J. Yang, C. L. Wang and Y. Zhu, Naphthodipyrrolidone (NDP) based conjugated polymers with high electron mobility and ambipolar transport properties, Polym. Chem., 2017, 8, 3255–3260 RSC.
- Z. F. Deng, X. L. Hao, P. F. Zhang, L. Q. Li, X. Q. Yuan, T. T. Ai, W. W. Bao and K. C. Kou, Donor-acceptor-donor molecules based on diketopyrrolopyrrole, benzodipyrrolidone and naphthodipyrrolidone: Organic crystal field-effect transistors, Dyes Pigm., 2019, 162, 883–887 CrossRef CAS.
- K. Kawabata, I. Osaka, M. Sawamoto, J. L. Zafra, P. Mayorga Burrezo, J. Casado and K. Takimiya, Dithienyl acenedithiophenediones as new pi-extended quinoidal cores: Synthesis and properties, Chem. – Eur. J., 2017, 23, 4579–4589 CrossRef CAS.
- M. S. Vezie, S. Few, I. Meager, G. Pieridou, B. Dorling, R. S. Ashraf, A. R. Goni, H. Bronstein, I. McCulloch, S. C. Hayes, M. Campoy-Quiles and J. Nelson, Exploring the origin of high optical absorption in conjugated polymers, Nat. Mater., 2016, 15, 746–753 CrossRef CAS.
- M. Chu, J. X. Fan, S. Yang, D. Liu, C. F. Ng, H. Dong, A. M. Ren and Q. Miao, Halogenated tetraazapentacenes with electron mobility as high as 27.8 cm2 V−1 s−1 in solution-processed n-channel organic thin-film transistors, Adv. Mater., 2018, 30, 1803467 CrossRef.
- X. Xu, Y. Yao, B. Shan, X. Gu, D. Liu, J. Liu, J. Xu, N. Zhao, W. Hu and Q. Miao, Electron mobility exceeding 10 cm2 V−1 s−1 and band-like charge transport in solution-processed n-channel organic thin-film transistors, Adv. Mater., 2016, 28, 5276–5283 CrossRef CAS.
- Z. Liang, Q. Tang, R. Mao, D. Liu, J. Xu and Q. Miao, The position of nitrogen in n-heteropentacenes matters, Adv. Mater., 2011, 23, 5514–5518 CrossRef CAS.
- X. Liu, B. He, C. L. Anderson, J. Kang, T. Chen, J. Chen, S. Feng, L. Zhang, M. A. Kolaczkowski, S. J. Teat, M. A. Brady, C. Zhu, L. W. Wang, J. Chen and Y. Liu, Para-azaquinodimethane: A compact quinodimethane variant as an ambient stable building block for high-performance low band gap polymers, J. Am. Chem. Soc., 2017, 139, 8355–8363 CrossRef CAS.
- X. Liu, B. He, A. Garzón-Ruiz, A. Navarro, T. L. Chen, M. A. Kolaczkowski, S. Feng, L. Zhang, C. A. Anderson, J. Chen and Y. Liu, Unraveling the main chain and side chain effects on thin film morphology and charge transport in quinoidal conjugated polymers, Adv. Funct. Mater., 2018, 28, 1801874 CrossRef.
- C. L. Anderson, N. Dai, S. J. Teat, B. He, S. Wang and Y. Liu, Electronic tuning of mixed quinoidal-aromatic conjugated polyelectrolytes: Direct ionic substitution on polymer main-chains, Angew. Chem., Int. Ed., 2019, 58, 17978–17985 CrossRef CAS.
- Z. Liang, Q. Tang, J. Xu and Q. Miao, Soluble and stable n-heteropentacenes with high field-effect mobility, Adv. Mater., 2011, 23, 1535–1539 CrossRef CAS.
- W. L. Leong, G. C. Welch, L. G. Kaake, C. J. Takacs, Y. Sun, G. C. Bazan and A. J. Heeger, Role of trace impurities in the photovoltaic performance of solution processed small-molecule bulk heterojunction solar cells, Chem. Sci., 2012, 3, 2103–2109 RSC.
- W. R. Mateker, J. D. Douglas, C. Cabanetos, I. T. Sachs-Quintana, J. A. Bartelt, E. T. Hoke, A. El Labban, P. M. Beaujuge, J. M. J. Fréchet and M. D. McGehee, Improving the long-term stability of PBDTTPD polymer solar cells through material purification aimed at removing organic impurities, Energy Environ. Sci., 2013, 6, 2529–2537 RSC.
- S. Wood, J. Wade, M. Shahid, E. Collado-Fregoso, D. D. C. Bradley, J. R. Durrant, M. Heeney and J.-S. Kim, Natures of optical absorption transitions and excitation energy dependent photostability of diketopyrrolopyrrole (DPP)-based photovoltaic copolymers, Energy Environ. Sci., 2015, 8, 3222–3232 RSC.
- S. Luňák, J. Vyňuchal and R. Hrdina, DFT and TD-DFT study of isomeric linear benzodifuranones, benzodipyrrolinones and their homologues, J. Mol. Struct., 2009, 935, 82–91 CrossRef.
- M. Shin, J. Y. Oh, K. E. Byun, Y. J. Lee, B. Kim, H. K. Baik, J. J. Park and U. Jeong, Polythiophene nanofibril bundles surface-embedded in elastomer: A route to a highly stretchable active channel layer, Adv. Mater., 2015, 27, 1255–1261 CrossRef CAS.
|
This journal is © the Partner Organisations 2021 |