DOI:
10.1039/D0QM00488J
(Research Article)
Mater. Chem. Front., 2021,
5, 333-340
Orange super-long persistent luminescent materials: (Sr1−xBax)3SiO5:Eu2+,Nb5+†
Received
15th July 2020
, Accepted 30th September 2020
First published on 30th September 2020
Abstract
Persistent luminescent materials are widely used as night-vision and marking materials in various important fields. Although significant achievements have been made in blue and green persistent luminescent (PersL) materials, the research and development of PersL materials in the warm-color region (550–660 nm) are relatively lacking. Only the orange PersL phosphor Y2O2S:Eu3+,Mg2+,Ti4+ (∼610 nm) and deep red PersL phosphor (Ca1−xSrx)S:Eu2+,Tm3+ (∼650 nm) fulfill the demands of commercial applications. However, sulfide phosphors have poor chemical stability and a relatively short persistent duration time. Herein, we report a series of orange PersL materials, (Sr1−xBax)3SiO5:Eu2+,Nb5+, which exhibit a strong PersL emission band at 550–670 nm and a super-long persistent time of more than 20 h at the 0.32 mcd m−2 threshold value after UV radiation. These new orange PersL materials are compared to the commercial warm-color sulfide PersL phosphor, Y2O2S:Eu,Mg,Ti, with regards to PersL time and brightness, and it is determined that they would have great potential applications.
Introduction
The persistent luminescence (PersL) phosphor was largely promoted in 1996 by the commercial green persistent phosphor, SrAl2O4:Eu2+,Dy3+,1 which has broad applications in many fields such as road markers that glow in the dark, emergency signs, optical information storage, etc. Since then, several other commercial Eu2+-doped blue or green persistent phosphors have been found, such as Sr4Al14O25:Eu2+, Dy3+,2,3 CaAl2O4:Eu2+,Nd3+
4–6 and Sr2MgSi2O7:Eu2+,Dy3+
7–9 (Fig. S1, ESI†). All these phosphors have not only good chemical stability, but also excellent PersL performance, high initial brightness and a long afterglow time. For example, SrAl2O4:Eu2+,Dy3+ has an initial persistent brightness of about 2000–5000 mcd m−2,10,11 with a persistent duration time of more than 30 h.12 Sr2MgSi2O7:Eu2+,Dy3+ has about 2200 mcd m−2 initial persistent brightness and at least 10 h of persistent duration time.8 Compared to these cool-color silicate and aluminate PersL phosphors, in the warm-color emission region, only the orange PersL phosphor Y2O2S:Eu3+,Mg2+,Ti4+
13,14 and deep red PersL phosphor (Ca1−xSrx)S:Eu2+,Tm3+
15 fulfill the high demands of commercial applications. However, these two sulfide phosphors have poor chemical stability and a relatively short persistent duration time. Thus, developing a stable, excellent warm-color PersL phosphor has great commercial value.
For all commercial PersL phosphors, a suitable trap is a necessary condition for high PersL performance. Hence, various strategies have been used by researchers to manipulate electron traps for designing novel PersL phosphors. Dorenbos et al. developed host referred binding energy (HRBE) and vacuum referred binding energy (VRBE) diagrams and they were successfully applied to predict the energy levels of lanthanide ions in many materials, such as LnPO4 (Ln = Y, La, Lu, Gd),16 ARE(Si,Ge)O4 (A = Li, Na; RE = Y, Lu),17 LnAlO3 (Ln =Y, Gd),18,19 SrSi2O2N2:Ln2+, Ln3+,20 Y3Al5Ga5−xO12:Ce3+ (YAGG)21,22 and the orange PersL phosphor Ca3Si2O7:Eu2+,Ln3+.23 This diagram even achieved success in the prediction of transition metal (TM) energy levels.24,25 Another condition for an excellent PersL material is a favorable charging and detrapping process. Conduction band engineering has been verified to be an effective way to control this process. Based on this engineering method, Ueda et al. reported a series of YAGG:Ce3+–Cr3+ PersL phosphors,26 which exhibit bright green PersL and can be efficiently charged by blue light. Wang et al. also reported the Lu2CaMg2Si3O12:Ce3+ PersL phosphor as a promising candidate in AC-LED through band gap tailoring via Ge doping.27
Besides Y2O2S:Eu3+,Mg2+,Ti4+, CaS:Eu2+,Tm3+ and Ca3Si2O7:Eu2+,Ln3+, several warm-color-emitting persistent phosphor materials have been reported in recent years, e.g. silicates such as Sr3SiO5:Eu2+,Dy3+,28,29 Sr3SiO5:Eu2+,Lu3+,30 CdSiO3:Eu3+,Mn2+,31,32 Ca2Al2SiO7:Mn2+,Ce3+ and MgSiO3:Mn2+,Eu2+,Dy3+,33 phosphates such as Ca6BaP4O17:Eu2+,Ho3+,34 nitride silicates such as (Ca,Sr)2Si5N8:Eu2+
35–38 and CaAlSiN3:Eu2+
39,40 and perovskite phosphors such as Ca3Ti2O7:Pr3+.41 However, compared to the two commercial sulfide compounds, the PersL performance of these phosphors has not been substantially improved. In our previous work, we improved the persistent luminescence performance of Sr3SiO5:Eu2+via Ge4+ and Nb5+ doping.42,43 Up to now, Nb5+-doped Sr3SiO5:Eu2+ has been shown to have about 1200 mcd m−2 initial persistent brightness and a persistent time of more than 14 h at the 0.32 mcd m−2 threshold value after UV radiation.
In this work, we utilize the chemical modification strategy of Ba/Sr cation replacement to develop a series of (Sr1−xBax)3SiO5:Eu2+,Nb5+ (x = 0–0.25) orange PersL materials. These materials display excellent persistent luminescence with 580–600 nm PersL emission and a persistence time of more than 20 h. A systematic comparison of PesL performance to commercial warm-color PersL phosphors is carried out. The comparison results show that (Sr1−xBax)3SiO5:Eu2+,Nb5+ has great potential to become a new commercial warm-color PersL phosphor.
Experimental
Materials and preparation
The (Sr1−xBax)3SiO5:0.5%Eu2+,0.5%Nb5+ (abbreviated as (Sr1−xBax)3SiO5:Eu2+,Nb5+) phosphors were prepared by the combustion method combined with the solid-state reaction method, using stoichiometric amounts of Sr(NO3)2 (99.9%, Sinopharm), Ba(NO3)2 (99.9%, Sinopharm), Eu(NO3)3·6H2O (99.9%, Aladdin), nano-SiO2 (15 nm, Aladdin), Nb2O5 (99.9%, Sinopharm) and NH3·H2O (25–28 wt%, Aladdin). The Sr(NO3)2, Ba(NO3)2, Eu(NO3)3·6H2O, nano-SiO2 and Nb2O5 were put into a ceramic bowl by adding 30 ml deionized water. Then, 5 ml of NH3·H2O was added under a constant stirring rate at room temperature. After all the raw materials were thoroughly mixed together, the ceramic bowl was transferred into a box furnace under 600 °C. After combustion, the mixed solution was ground into powder in an agate mortar and calcinated at 900 °C for 3 h. The obtained samples were naturally cooled down to room temperature, then ground into powder again and finally transferred into an alumina crucible at 1450 °C for 4 h under a weak reducing atmosphere of 10% H2/90% N2. The samples were allowed to cool to room temperature naturally and ground to fine powders for subsequent measurements. The commercial persistent luminescence phosphors Y2O2S:Eu3+,Mg2+,Ti4+ and (Ca,Sr)S:Eu2+,Tm3+ were bought from Dalian Lu-Ming Technology Group Co., Ltd.
Characterization
The X-ray diffraction (XRD) data were collected on a X’Pert3 powder diffractometer (Malvern Panalytical Corporation, Netherlands) (Cu Kα radiation, λ = 1.5406 Å) operating at 40 kV and 40 mA in the 2θ range from 20 to 70°. The steady photoluminescence spectra were measured by an Edinburgh Instruments, FLS 920 fluorescence spectrophotometer, equipped with a 450 W Xenon lamp as the radiating source. The persistent time was measured by a phosphor photometer (PR-305, SENSINGM), using 8 W 254 and 365 nm UV tubes as the excitation light source (ZF-20D, Shanghai Guanghao Analytical Instruments Co. Ltd). Thermoluminescence (TL) curves were obtained by a TOSL-3DS instrument (Ai-dirui-sheng Company, Guangzhou, China), equipped with a Hg lamp as the UV radiating source in the temperature range of 300–623 K, with a heating rate of 5 K s−1. For the persistent luminescence (PersL) excitation curve, prior to measurements, the samples were heated to 673 K in order to empty all the traps. The persistent luminescence (PersL) excitation curve is obtained with a series of PersL emission curves under various (monochromatic) excitation wavelengths from 240 to 500 nm, and then those curves are integrated as a function of the excitation wavelengths, i.e. the PersL excitation curve. The digital photographs were obtained using a HUAWEI P30 mobile phone. The excitation spectra in the VUV-UV range of (Sr1−xBax)3SiO5:Eu2+,Nb5+ were measured on the beam line 4B8 of the Beijing Synchrotron Radiation Facility (BSRF) under normal operating conditions using the spectrum of sodium salicylate (o-C6H4OHCOONa) as a reference.
Results and discussion
Crystal structure and photoluminescence
The end-member compound Sr3SiO5 crystallized in the tetragonal system with the space group P4/ncc (no. 130), while Ba3SiO5 belongs to the space group I4/mcm (no. 140). Fig. 1a shows the X-ray diffraction (XRD) patterns of the (Sr1−xBax)3SiO5:Eu2+,Nb5+ samples (x = 0–0.50). All the diffraction peaks of the x ≦ 0.30 phosphors are indexed to the standard data of the Sr3SiO5 phase. For the x = 0.4 and x = 0.5 samples, there exists a small Sr2SiO4 impurity phase, as the red symbol indicates in Fig. 1a. By increasing the x value from 0 to 0.5, the XRD peaks gradually shift to lower angles due to the lattice expansion by the substitution of larger Ba2+ ions (r = 1.35 Å, CN = 6) for Sr2+ ions (r = 1.18 Å, CN = 6). Magnified XRD patterns in the range of 29–31° clearly describe this tendency. To further explore the structure variation, Le Bail refinements of the XRD patterns using Fullprof44 software were performed, which show that with the Ba content increasing, the samples have larger cell parameters and the cell volumes increase from 521 Å3 to 539 Å3. Dependence of the lattice parameters and unit cell volumes on various x values are shown in Fig. 1b. According to Vegard's law, for continuous solid solutions without preferential occupation, the cell parameters will change in a linear relationship with the composition. However, for the (Sr1−xBax)3SiO5:Eu2+,Nb5+ samples, different slopes of the fitting curves for the composition dependence of the cell parameters occur at x = 0.20. This result shows that there exists a preferential substitution behavior when Sr ions are replaced by Ba.45Fig. 1c displays the crystal structure of Sr3SiO5 showing three types of coordination, i.e. the two Sr polyhedral sites and one Si tetrahedral site. It can be seen that the [Sr2–O] polyhedra are arranged along the c direction, and the c length is equal to the sum of four Sr2–O2 bonds (r = 2.680 Å) (Fig. 1d). The elongation of the c axis is mainly determined by an increase in the Sr2–O2 bond length, while the elongation of the a axis is mainly determined by the Sr1–O bond. Hence, for the phosphors of x = 0–0.18, the Ba ions preferentially occupy the Sr2 sites, which leads to a faster increasing of the c value (Fig. 1b). Meanwhile, when x = 0.18–0.5, the Ba ions take over the Sr1 sites, leading to a slower increasing of the c value and a faster increasing of the a value.
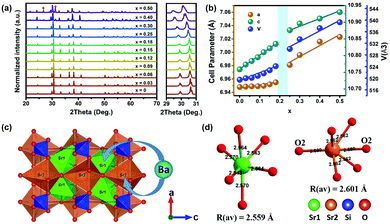 |
| Fig. 1 (a) XRD patterns of the (Sr1−xBax)3SiO5:Eu2+,Nb5+ samples. The samples of x = 0.4 and x = 0.5 have a small Sr2SiO4 phase (the red symbol). Magnified XRD patterns are in the range of 29–31°. (b) Dependence of the lattice parameters and unit cell volumes on various x values. (c) Crystal structure of Sr3SiO5 with a 4-fold coordination Si and a 6-fold coordination Sr polyhedron, Ba ions tend to occupy the Sr sites. (d) The bond length of the Sr1 and Sr2 sites. | |
The steady-state photoluminescence excitation (PLE) spectra of (Sr1−xBax)3SiO5:Eu2+,Nb5+ (x = 0–0.50) phosphors with different Ba contents are shown in Fig. 2a. The broad PLE bands from 250 to 530 nm were assigned to Eu2+ 4f → 5d (including both low and high 5d levels) electronic transitions. It shows that the broad excitation bands basically do not change with Ba replacement contents. For the normalized emission spectra of (Sr1−xBax)3SiO5:Eu2+,Nb5+, the emission peak wavelengths (denoted as λem) undergo a red shift from 580 to 600 nm as x increases from 0 to 0.18 and then blue shift from 600 to 586 nm with higher x contents (Fig. 2b). This phenomenon is consistent with previous reports.46 Data on the f–d spectroscopy of Eu2+ in the compounds have been analyzed and are compiled in Table 1.
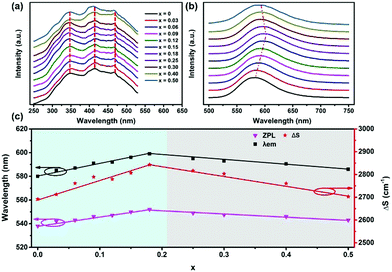 |
| Fig. 2 (a) PLE spectra of (Sr1−xBax)3SiO5:Eu2+,Nb5+ samples measured under 580 nm emission wavelength at room temperature. (b) PL spectra of all the phosphors measured under 410 nm excitation wavelength. (c) λem, ZPL line and Stokes shift (ΔS) as functions of x. | |
Table 1 Spectroscopic data of Eu2+ in the (Sr1−xBax)3SiO5:Eu2+,Nb5+ compounds
x
|
λ
em (nm) |
ZPL (nm) |
ΔS (cm−1) |
0 |
580 |
538 |
2692 |
0.03 |
585 |
542 |
2712 |
0.06 |
587 |
543 |
2761 |
0.09 |
591 |
546 |
2789 |
0.12 |
592 |
547 |
2779 |
0.15 |
596 |
550 |
2806 |
0.18 |
599 |
552 |
2843 |
0.25 |
595 |
549 |
2816 |
0.30 |
593 |
547.5 |
2803 |
0.40 |
590.5 |
546 |
2760 |
0.50 |
586 |
543 |
2703 |
The λem and zero-phonon line (ZPL) energy first red shift with x from 0 to 0.18 and then blue shift when x > 0.18 (Fig. 2c). The Stokes shift (ΔS) shows a similar trend. The zero-phonon energy values are taken from the cross point between the excitation and emission spectra. As the average bond length of Ba–O is larger than that of Sr–O, it is expected that the lowest 5d excitation state of Eu2+ will shift to higher energy due to a smaller crystal-field splitting. However, the unshifted PLE peak is inconsistent with expectation. This unusual behavior may be explained by the following reason. It is known that the emission band wavelength is generally determined by the location of the lowest 5d energy level and Stokes shift (ΔS). However, the cation substitutions have little effect on the crystal-field splitting of the 5d levels of the Eu2+ ions due to a special local structure, such as that in La3Si6N11:Ce3+.47 Hence, in (Sr1−xBax)3SiO5:Eu2+,Nb5+ phosphors, λem is mainly determined by the Stokes shift. In the range of x = 0–0.18, the Stokes shift slightly increases with Ba contents, leading to the red shift of emission band. In the range of x = 0.18–0.50, the blue shift of the emission band can be mainly attributed to the decreasing Stokes shift. This variation in Stokes shift with Ba substitution possibly originates from the changes of local structure, such as in the cases of (Ca1−xLix)(Al1−xSi1+x)N348 and Sr(LiAl)1−xMg2xAl2N4:Eu2+.49
Persistent luminescence properties
The persistent luminescence decay curves of the (Sr1−xBax)3SiO5:Eu2+,Nb5+ samples were measured at room temperature to quantitatively compare their persistent luminescence properties. All the phosphors display an obvious persistent luminescence. Fig. 3a shows the afterglow brightness as a function of decay duration (log–log plot) for the samples with different Ba contents after 5 min UV light excitation (254 nm, 8 W lamp). The red dashed line fixed at 0.32 mcd m−2 represents the threshold value used in the industrial standard, defined as about 100 times higher than the sensitivity of human eyes.1,50 Here, we can see that the sample without Ba has the biggest initial brightness of about 1438 mcd m−2 and the sample with x = 0.15 has the longest decay time of about 23 h (more than 20 h). When the Ba content increases to 0.25, the persistent luminescence properties are sharply weakened. Hence, samples with a higher Ba content were not under our consideration in this work. Fig. 3c presents the persistent luminescence spectra (PersL) of (Sr1−xBax)3SiO5:Eu2+,Nb5+ after the UV excitation turn-off. These samples show yellow – orange persistent emission. The persistent emission wavelength first red shifts and then blue shifts, corresponding to the photographic images shown to the right. To further study the afterglow properties of the materials, the charging–discharging curve of Sr3SiO5:Eu2+,Nb5+ under λex = 254 nm was obtained, as shown in Fig. 3d. It can be seen that the emission intensities increase with charging time; this is a charging process. When the charging time reaches 1000 s, the maximum intensity is reached. This intensity is contributed by PL intensity (77%) and PersL intensity (23%). Based on previous research,51,52 the efficiency of the persistent luminescence is determined to be about 60% (Fig. S2, ESI†). This is a very high persistent luminescence efficiency. As a comparison, the persistent luminescence efficiency of SrAl2O4:Eu,Dy under 435 nm excitation is about 65%, as reported by Smet et al.51
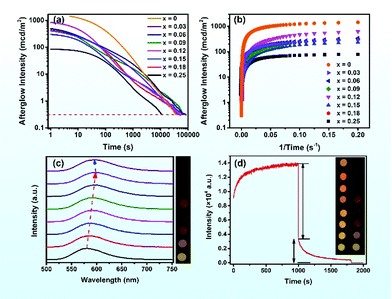 |
| Fig. 3 (a) Persistent luminescence decay curves of the (Sr1−xBax)3SiO5:Eu2+,Nb5+ samples after 5 min UV irradiation. (b) Function of afterglow intensity (I) versus reciprocal time (t−1) of all the samples excited by UV light for 5 min. (c) PersL emission spectra of (Sr1−xBax)3SiO5:Eu2+,Nb5+ after UV charging. (d) The charging curve (λex = 254 nm) started at t = 0 s and afterglow curve started at t = 1000 s for the Sr3SiO5:Eu2+,Nb5+ samples. | |
Thermoluminescence (TL) and persistent luminescence (PersL) excitation spectra
In order to better understand the Ba/Sr-ratio-dependent PersL behavior, we evaluated the TL glow curves of all the samples in detail. As shown in Fig. 4a, all the TL glow curves exhibited a very broad TL peak and a relatively high TL intensity. The peak temperature of the TL glow curves and integrated intensity of the (Sr1−xBax)3SiO5:Eu2+,Nb5+ samples after 254 nm UV irradiation are plotted as a function of Ba concentration, as shown in Fig. 4b. As x increases, the TL peaks shift to a higher temperature from 360 to 464 K, indicating deeper trap depths. Also, we can see that the TL intensity shows a continuous rising trend with x, which means an obvious increase in trap quantity. Therefore, the persistent initial brightness becomes weaker and the persistent luminescence decay time increases, which is consistent with the phenomenon in Fig. 3a.
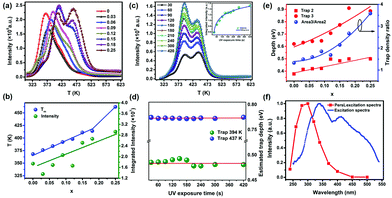 |
| Fig. 4 (a) TL glow curves of the (Sr1−xBax)3SiO5:Eu2+,Nb5+ samples after UV light excitation from a Hg lamp at temperatures from 300 to 623 K (heating rate, 5 K s−1 and radiation time, 100 s). (b) Integrated TL intensity and TL peak temperature of the (Sr1−xBax)3SiO5:Eu2+,Nb5+ samples. (c) TL glow curves of the (Sr0.85Ba0.15)3SiO5:Eu2+,Nb5+ sample after UV light excitation at room temperature with various durations (30–420 s), at a heating rate of 5 K s−1. (d) Estimated traps depth in (Sr0.85Ba0.15)3SiO5:Eu2+,Nb5+ for increasing excitation duration. (e) Estimated traps depth (mainly trap 2 and trap 3, see Fig. S3, ESI†) and trap density ratio value in (Sr1−xBax)3SiO5:Eu2+,Nb5+. (f) PersL excitation spectra of (Sr0.85Ba0.15)3SiO5:Eu2+,Nb5+ obtained from the PersL intensity at 595 nm after 120 s, monitored as a function of the excitation wavelength from 240 nm to 500 nm. | |
Fig. 4c presents the TL curves by pre-exciting the sample at 254 nm (UV lamp, 3 W) for different durations and then recording at a heating rate of 5 K s−1. The TL intensity enhances with prolonging the exposure time, because more electrons are captured by the traps. The intensity of the TL peak at about 394 K increases more obviously than that of the peak at about 437 K, which already has been reported by many researchers.51 However, the positions of all the TL peaks show no shift for various duration times, and the trap depths show no change (Fig. 4d). This phenomenon suggests that the traps obey first order kinetics, that is, each electron is released from the traps and then immediately produces luminescence, rather than being re-captured by traps. Hence, we can use the first order kinetic function to fit all the TL glow curves. Detailed fitting information can be found in Fig. S3 (ESI†). The trap depth and trap density ratio of trap 2 to trap 3 were obtained and their variations vs. compositions are shown in Fig. 4e. These results indicate that Ba/Sr substitution can alter the depth of the traps, leading to super-long persistent luminescence for (Sr1−xBax)3SiO5:Eu2+,Nb5+.
To understand the efficiency of different excitation wavelengths for producing the PersL in (Sr1−xBax)3SiO5:Eu2+,Nb5+ and to further explore the persistent luminescence mechanism, persistent luminescence (PersL) excitation curves are recorded. Fig. 4f presents the PersL excitation curve of (Sr0.88Ba0.12)3SiO5:Eu2+,Nb5+ excited by wavelengths from 240 to 500 nm. The PersL excitation curve is mainly located at shorter wavelengths compared to that of the photoluminescence excitation (PLE) spectrum. For PLE, the intensity remains at a high level from 330–400 nm, in which region the intensity of PersL excitation is diminished. This indicates that the steady state excitation process is not the same as the PersL excitation process. The traps can be efficiently charged by electrons excited directly from the 4f7 to higher 5d excited levels closed or submerged in the conduction band, rather than the lower 5d excited levels. This feature for the PersL excitation process has also been observed in many persistent phosphors such as (Ca,Sr)AlSiN3:Eu2+
40 and SiAlON:Eu2+.53
Excellent persistent luminescence properties and comparison to the commercial phosphors
The (Sr1−xBax)3SiO5:Eu2+,Nb5+ samples exhibit suitable deep traps, a broad trap depth distribution and high trap density (Fig. 4a), which are beneficial for persistent luminescence.54 Herein, we show the excellent persistent luminescence of the Sr3SiO5:Eu2+,Nb5+ and (Sr0.88Ba0.12)3SiO5:Eu2+,Nb5+ samples and compare them with the commercial phosphors, Y2O2S:Eu3+,Mg2+,Ti4+ and (Ca,Sr)S:Eu2+,Tm3+. As shown in Fig. 6a, four persistent phosphors (denoted as 1–4) show different body colors (yellow, white, orange and red) under 254 nm radiation. However, when the UV light is turned off, Y2O2S:Eu3+,Mg2+,Ti4+ presents an orange color, while the other three phosphors show no changes. From left to right, the images were taken at the times corresponding to turn off instantaneously and after 30, 60, 120, 300 and 600 seconds, respectively. We can see that Y2O2S:Eu3+,Mg2+,Ti4+ has the highest initial brightness. However, the intensity decreases faster compared to Sr3SiO5:Eu2+,Nb5+. A similar phenomenon can be seen (Fig. 5a). Fig. 5b presents the TL curves of the four samples under a heating rate of 5 K s−1. This clearly shows that all the phosphors have broad TL spectra covering 300–423 K. Our (Sr1−xBax)3SiO5:Eu2+,Nb5+ samples have a bigger TL intensity compared with commercial sulfide phosphors. Fig. 5c shows the afterglow brightness as a function of decay duration (a log-linear plot). Y2O2S:Eu3+,Mg2+,Ti4+ has the largest initial afterglow brightness (2539 mcd m−2, 5 seconds) and the next is Sr3SiO5:Eu2+,Nb5+ with about 1438 mcd m−2. Under the same test conditions, Sr3SiO5:Eu2+,Nb5+, Y2O2S:Eu3+,Mg2+,Ti4+, (Sr0.88Ba0.12)3SiO5:Eu2+,Nb5+ and (Ca,Sr)S:Eu2+,Tm3+ show afterglow durations of 12.5, 3.68, 17.5 and 0.36 h, respectively. Detailed results are listed in Table 2. Fig. 5d displays the PersL emission spectra of the four phosphors excited by 254 nm UV light. The PersL emission spectra of Y2O2S:Eu3+,Mg2+,Ti4+ is composed of two parts. The broad yellow-orange emission comes from partially converted Ti4+, while the sharp emission is from Eu3+ f–f emission.13,14 The other three phosphors show one broad PersL emission spectra originating from Eu2+ 5d–4f emission. Fig. 5e shows the CIE coordinates of the four persistent phosphors. (Sr0.88Ba0.12)3SiO5:Eu2+,Nb5+ has very similar CIE coordinates to Y2O2S:Eu3+,Mg2+,Ti4+. On the basis of the above results, we think (Sr0.88Ba0.12)3SiO5:Eu2+,Nb5+ has great potential to serve as a new warm-colored persistent phosphor.
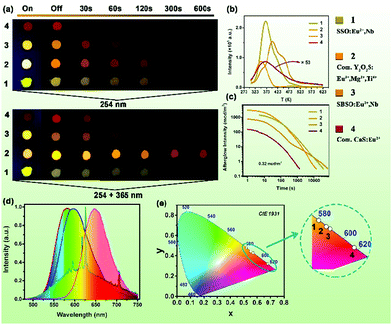 |
| Fig. 5 (a) Warm-light PersL images of (Sr, Ba)3SiO5:Eu2+,Nb5+ obtained at different PersL decay times after 254 nm UV-lamp excitation for 5 min (upper image) and after 254 nm + 365 nm UV-lamp excitation for 5 min (lower image). (b) TL glow curves of Sr3SiO5:Eu2+,Nb5+ (red line, denoted as 1), Y2O2S:Eu3+,Mg2+,Ti4+ (green line, denoted as 2), (Sr0.88Ba0.12)3SiO5:Eu2+,Nb5+ (blue line, denoted as 3) and (Ca,Sr)S:Eu2+ (purple line, denoted as 4). (c) Afterglow decay as a function of time. (d) PersL emission spectra of those four kinds of phosphors. (e) CIE coordinates of the four persistent phosphors. | |
Table 2 A detailed comparison of the PersL performance of (Sr,Ba)3SiO5:Eu2+,Nb5+ and commercial warm-color PersL phosphors
Materials |
CIE |
Peak wavelength (nm) |
Intensity (mcd m−2) |
Persistent time (h) |
5 s |
10 min |
60 min |
Under 254 + 365 nm radiation.
Under 254 nm radiation.
|
Y2O2S:Eu3+,Mg2+,Ti4+ a |
(0.5512, 0.4411) |
597, 626 |
2119 |
50.5 |
2.84 |
∼5 |
Y2O2S:Eu3+,Mg2+,Ti4+ b |
(0.5512, 0.4411) |
597, 626 |
2216 |
40.5 |
1.46 |
∼4 |
Sr3SiO5:Eu2+,Nb5+-1a |
(0.5186, 0.4777) |
585 |
1438.7 |
68.2 |
6.36 |
10–14 |
Sr3SiO5:Eu2+,Nb5+-2a |
(0.5186, 0.4777) |
585 |
652 |
48.4 |
8.2 |
10–14 |
(Sr,Ba)3SiO5:Eu2+,Nb5+ a |
(0.5694, 0.4290) |
595 |
605.4 |
9.8 |
3.02 |
13–22 |
(Ca,Sr)S:Eu2+ a |
(0.6769, 0.3199) |
650 |
93.11 |
1.11 |
— |
∼1 |
Persistent luminescence mechanism
Several models were proposed to explain the mechanism of persistent luminescence. Herein, combining our previous work,39,40 the following mechanism is suggested to interpret the super-long persistence luminescence of the (Sr,Ba)3SiO5:Eu2+,Nb5+ phosphor, as shown in Fig. 6. To simplify the description, we labelled all the traps (mainly Trap 2 and Trap 3, as shown in Fig. 4e) as Traps (the light green rectangle in Fig. 6). As the Ba content increases, the band gap decreases (Fig. S4, ESI†). Also, all the traps become deeper and the trap density gets denser (Fig. 4e). The deeper the trap is, the longer the decay time will be. Herein, we choose (Sr0.88Ba0.12)3SiO5:Eu2+,Nb5+ as an example for the persistent luminescence mechanism. In the charging process (process i in Fig. 6), electrons are excited from the 4f ground state to the higher 5d levels by ultraviolet irradiation with suitable energy larger than 3.10 eV (400 nm). In Fig. 4f, the PersL excitation intensity of (Sr0.88Ba0.12)3SiO5:Eu2+,Nb5+ nearly falls to zero at around 500 nm (∼2.48 eV) while the PLE spectrum still remains at a high level. After excitation, the mobile electrons are transported through the conduction band and are captured by various depths of trap, as shown by the violet dotted arrow (process ii). The trap depth ranges from 0.48 to 0.71 eV. After that, the electrons in the traps can be thermally promoted into the conduction band at room temperature and then released to the lowest 5d excited state of Eu2+ (process iii). Subsequently, those released electrons return to the 4f ground state and yield yellow-orange PersL (process iv).
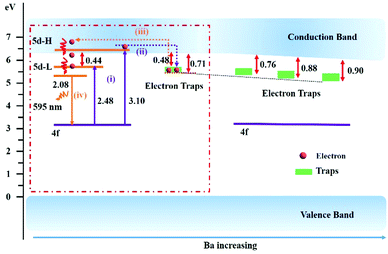 |
| Fig. 6 A schematic representation of the yellow-orange PersL mechanisms of (Sr1−xBax)3SiO5:Eu2+,Nb5+. Only (Sr0.88Ba0.12)3SiO5:Eu2+,Nb5+ is given in the red rectangle. Other Ba content compounds have similar PersL mechanisms. | |
Conclusions
In summary, a series of orange super-long persistent luminescent materials, (Sr1−xBax)3SiO5:Eu2+,Nb5+ (x = 0–0.25), have been synthesized by the combustion method combined with the solid-state reaction method successfully. The Ba substitution for Sr can not only lead to a red shift of the persistent emission peak wavelength from 580 to 600 nm, but also the PersL time can be improved to a very long duration (22 h for x = 0.15). A systematic comparison of the PesL performance of (Sr,Ba)3SiO5:Eu2+,Nb5+ and commercial warm-colored PersL phosphors is carried out. (Sr0.88Ba0.12)3SiO5:Eu2+,Nb5+ has a very close similar coordinate to Y2O2S:Eu3+,Mg2+,Ti4+, and a super-long persistent decay time (about 17 hours). The comparison results show that (Sr,Ba)3SiO5:Eu2+,Nb5+ has excellent afterglow performance, which gives it great potential to become a new commercial warm-colored PersL phosphor. Comparing the TL, PLE and PersL excitation curve measurements, a model was carried out to understand the excellent afterglow performance. This material with high persistent brightness, super-long afterglow time and warm color can make up for the lack of PersL materials in the orange region and could greatly expand the application of PersL materials in energy, environmental, and optical information storage fields.
Conflicts of interest
There are no conflicts to declare.
Acknowledgements
This work is supported by the National Natural Science Foundation of China (No. 51672027, 51832005).
Notes and references
- T. Matsuzawa, Y. Aoki, N. Takeuchi and Y. Murayama, A New Long Phosphorescent Phosphor with High Brightness, SrAl2O4:Eu2+, Dy3+, J. Electrochem. Soc., 1996, 143, 2670–2673 CrossRef CAS.
- Y. H. Lin, Z. L. Tang and Z. T. Zhang, Preparation of long-afterglow Sr4Al14O25-based luminescent material and its optical properties, Mater. Lett., 2001, 51, 14–18 CrossRef CAS.
- E. Nakazawa, Y. Murazaki and S. Saito, Mechanism of the persistent phosphorescence in Sr4Al14O25:Eu and SrAl2O4:Eu codoped with rare earth ions, J. Appl. Phys., 2006, 100, 113113 CrossRef.
- H. Yamamoto and T. Matsuzawa, Mechanism of long phosphorescence of SrAl2O4: Eu2+,Dy3+ and CaAl2O4: Eu2+, Nd3+, J. Lumin., 1997, 72, 287–289 CrossRef.
- W. Y. Jia, H. B. Yuan, L. Z. Lu, H. M. Liu and W. M. Yen, Crystal growth and characterization of Eu2+,Dy3+: SrAl2O4 and Eu2+,Nd3+: CaAl2O4 by the LHPG method, J. Cryst. Growth, 1999, 200, 179–184 CrossRef CAS.
- J. Hölsä, H. Jungner, M. Lastusaari and J. Niittykoski, Persistent luminescence of Eu2+ doped alkaline earth aluminates, MAl2O4: Eu2+, J. Alloys Compd., 2001, 323, 326–330 CrossRef.
- Y. H. Lin, Z. L. Tang, Z. T. Zhang, X. X. Wang and J. Y. Zhang, Preparation of a new long afterglow blue-emitting Sr2MgSi2O7-based photoluminescent phosphor, J. Mater. Sci. Lett., 2001, 20, 1505–1506 CrossRef CAS.
- Y. H. Lin, C.-W. Nan, X. S. Zhou, J. B. Wu, H. F. Wang, D. P. Chen and S. M. Xu, Preparation and characterization of long afterglow M2MgSi2O7-based (M: Ca, Sr, Ba) photoluminescent phosphors, Mater. Chem. Phys., 2003, 82, 860–863 CrossRef CAS.
- Q. Fei, C. K. Chang and D. L. Mao, Luminescent properties of Sr2MgSi2O7 and Ca2MgSi2O7 long lasting phosphors activated by Eu2+, Dy3+, J. Alloys Compd., 2005, 390, 133–137 CrossRef CAS.
- H. J. Song and D. H. Chen, Combustion synthesis and luminescence properties of SrAl2O4:Eu2+,Dy3+,Tb3+ phosphor, Luminescence, 2007, 22, 554–558 CrossRef CAS.
- Y. N. Zhu, Z. Y. Pang, J. Wang, M. Q. Ge, S. J. Sun, Z. H. Hu, J. H. Zhai, J. X. Gao and F. S. Jiang, Effect of light conversion agent on luminous properties of a new down-converting material SrAl2O4:Eu2+,Dy3+/light conversion agent, J. Rare Earths, 2016, 34, 483–488 CrossRef CAS.
- T. Katsumata, T. Nabae, K. Sasajima, S. Komuro and T. Morikawa, Effects of Composition on the Long Phosphorescent SrAl2O4:Eu2+,Dy3+ Phosphor Crystals, J. Electrochem. Soc., 1997, 144, L243 CrossRef CAS.
- C.-C. Kang, R.-S. Liu, J.-C. Chang and B.-J. Lee, Synthesis and luminescent properties of a new yellowish-orange afterglow phosphor Y2O2S:Ti, Mg, Chem. Mater., 2003, 15, 3966–3968 CrossRef CAS.
- Y. H. Wang and Z. L. Wang, Characterization of Y2O2S:Eu3+,Mg2+,Ti4+ long-lasting phosphor synthesized by flux method, J. Rare Earths, 2006, 24, 25–28 CrossRef.
- D. D. Jia, W. Y. Jia, D. Evans, W. Dennis, H. M. Liu, J. Zhu and W. Yen, Trapping processes in CaS: Eu2+, Tm3+, J. Appl. Phys., 2000, 88, 3402–3407 CrossRef CAS.
- T. Lyu and P. Dorenbos, Charge carrier trapping processes in lanthanide doped LaPO4, GdPO4, YPO4, and LuPO4, J. Mater. Chem. C, 2018, 6, 369–379 RSC.
- T. Lyu and P. Dorenbos, Vacuum referred binding energies of bismuth and lanthanide levels in ARE(Si, Ge)O4 (A = Li, Na; RE = Y,Lu); towards designing charge carrier trapping processes for energy storage, Chem. Mater., 2020, 32, 1192–1209 CrossRef CAS.
- H. D. Luo, A. J. Bos and P. Dorenbos, Controlled electron–hole trapping and detrapping process in GdAlO3 by valence band engineering, J. Phys. Chem. C, 2016, 120, 5916–5925 CrossRef CAS.
- H. D. Luo and P. Dorenbos, The dual role of Cr3+ in trapping holes and electrons in lanthanide co-doped GdAlO3 and LaAlO3, J. Mater. Chem. C, 2018, 6, 4977–4984 RSC.
- Y. X. Zhuang, Y. Lv, L. Wang, W. W. Chen, T.-L. Zhou, T. Takeda, N. Hirosaki and R.-J. Xie, Trap depth engineering of SrSi2O2N2: Ln2+, Ln3+ (Ln2+= Yb, Eu; Ln3+= Dy, Ho, Er) persistent luminescence materials for information storage applications, ACS Appl. Mater. Interfaces, 2018, 10, 1854–1864 CrossRef CAS.
- J. Ueda, P. Dorenbos, A. J. Bos, K. Kuroishi and S. Tanabe, Control of electron transfer between Ce3+ and Cr3+ in the Y3Al5−xGaxO12 host via conduction band engineering, J. Mater. Chem. C, 2015, 3, 5642–5651 RSC.
- Y. Katayama, A. Hashimoto, J. Xu, J. Ueda and S. Tanabe, Thermoluminescence investigation on Y3Al5−xGaxO12:Ce3+-Bi3+ green persistent phosphors, J. Lumin., 2017, 183, 355–359 CrossRef CAS.
- J. Ueda, R. Maki and S. Tanabe, Vacuum referred binding energy (VRBE)-guided design of orange persistent Ca3Si2O7:Eu2+ phosphors, Inorg. Chem., 2017, 56, 10353–10360 CrossRef CAS.
- P. Dorenbos and E. G. Rogers, Vacuum referred binding energies of the lanthanides in transition metal oxide compounds, ECS J. Solid State Sci. Technol., 2014, 3, R150–R158 CrossRef CAS.
- E. Rogers and P. Dorenbos, Vacuum referred binding energy of the single 3d, 4d, or 5d electron in transition metal and lanthanide impurities in compounds, ECS J. Solid State Sci. Technol., 2014, 3, R173 CrossRef CAS.
- J. Ueda, K. Kuroishi and S. Tanabe, Bright persistent ceramic phosphors of Ce3+-Cr3+-codoped garnet able to store by blue light, Appl. Phys. Lett., 2014, 104, 101904 CrossRef.
- H. Lin, B. Wang, Q. M. Huang, F. Huang, J. Xu, H. Chen, Z. B. Lin, J. M. Wang, T. Hu and Y. S. Wang, Lu2CaMg2(Si1−xGex)3O12: Ce3+ solid-solution phosphors: bandgap engineering for blue-light activated afterglow applicable to AC-LED, J. Mater. Chem. C, 2016, 4, 10329–10338 RSC.
- X. Y. Sun, J. H. Zhang, X. Zhang, Y. S. Luo and X.-J. Wang, Long lasting yellow phosphorescence and photostimulated luminescence in Sr3SiO5:Eu2+ and Sr3SiO5:Eu2+,Dy3+ phosphors, J. Phys. D: Appl. Phys., 2008, 41, 195414 CrossRef.
- Y. Li, B. H. Li, C. C. Ni, S. X. Yuan, J. Wang, Q. Tang and Q. Su, Synthesis, Persistent Luminescence, and Thermoluminescence Properties of Yellow Sr3SiO5:Eu2+,RE3+ (RE = Ce, Nd, Dy, Ho, Er, Tm, Yb) and Orange-Red Sr3−xBaxSiO5:Eu2+,Dy3+ Phosphor, Chem. – Asian J., 2014, 9, 494–499 CrossRef CAS.
- X. Yu, T. Wang, X. H. Xu, D. C. Zhou and J. B. Qiu, Yellow Photo- Stimulated Long Persistent Luminescence in Strontium Silicate Phosphor, ECS Solid State Lett., 2013, 3, R4 CrossRef.
- J. Y. Kuang, Y. L. Liu and B. F. Lei, Effect of RE3+ as a co-dopant in long-lasting phosphorescence CdSiO3: Mn2+ (RE= Y, La, Gd, Lu), J. Lumin., 2006, 118, 33–38 CrossRef CAS.
- X. F. Qu, L. X. Cao, W. Liu, G. Su and P. P. Wang, Luminescence properties of CdSiO3: Mn2+, RE3+ (RE= Sm, Dy, Eu) phosphors, J. Alloys Compd., 2009, 487, 387–390 CrossRef CAS.
- X.-J. Wang, D. D. Jia and W. M. Yen, Mn2+ activated green, yellow, and red long persistent phosphors, J. Lumin., 2003, 102, 34–37 CrossRef.
- H. J. Guo, W. B. Chen, W. Zeng, G. Li, Y. H. Wang, Y. Y. Li, Y. Li and X. Ding, Structure and luminescence properties of a novel yellow super long-lasting phosphate phosphor Ca6BaP4O17:Eu2+,Ho3+, J. Mater. Chem. C, 2015, 3, 5844–5850 RSC.
- K. Van den Eeckhout, P. F. Smet and D. Poelman, Persistent luminescence in rare-earth codoped Ca2Si5N8:Eu2+, J. Lumin., 2009, 129, 1140–1143 CrossRef CAS.
- X. M. Teng, Y. H. Liu, Y. Z. Liu, Y. S. Hu, H. Q. He and W. B. Zhuang, Luminescence properties of Tm3+ co-doped Sr2Si5N8:Eu2+ red phosphor, J. Lumin., 2010, 130, 851–854 CrossRef CAS.
- H. R. Zhang, H. W. Dong, B. F. Lei, P. Wang, J. F. Li, Y. L. Liu, J. Wang, Y. Xiao, M. T. Zheng and J. X. Meng, Enhanced performance of Ca2Si5N8:Eu2+,Tm3+ reddish-orange afterglow phosphor by co-doping with Dy3+, Opt. Mater., 2014, 36, 1846–1849 CrossRef CAS.
- S. X. Wang, Z. Song, Y. W. Kong, S. Y. Zhang, Z. G. Xia and Q. L. Liu, Enhanced performance of Sr2Si5N8:Eu2+ red afterglow phosphor by co-doping with boron and oxygen, J. Lumin., 2018, 204, 36–40 CrossRef CAS.
- J. Ueda, S. Tanabe, K. Takahashi, T. Takeda and N. Hirosaki, Thermal quenching mechanism of CaAlSiN3:Eu2+ red phosphor, Bull. Chem. Soc. Jpn., 2018, 91, 173–177 CrossRef CAS.
- X. L. Liu, Z. Song, S. X. Wang and Q. L. Liu, The red persistent luminescence of (Sr,Ca)AlSiN3:Eu2+ and mechanism different to SrAl2O4:Eu2+,Dy3+, J. Lumin., 2019, 208, 313–321 CrossRef CAS.
- B. Wang, H. Lin, J. Xu, H. Chen, Z. B. Lin, F. Huang and Y. S. Wang, Design, preparation, and characterization of a novel red long-persistent perovskite phosphor: Ca3Ti2O7:Pr3+, Inorg. Chem., 2015, 54, 11299–11306 CrossRef CAS.
- Z. Z. Wang, Z. Song, L. X. Ning, Z. G. Xia and Q. L. Liu, Enhanced Yellow Persistent Luminescence in Sr3SiO5: Eu2+ through Ge Incorporation, Inorg. Chem., 2019, 58, 8694–8701 CrossRef CAS.
- Z. Z. Wang, Z. Song, L. X. Ning and Q. L. Liu, Sunlight-activated yellow long persistent luminescence from Nb-doped Sr3SiO5:Eu2+ for warm-color mark applications, J. Mater. Chem. C, 2020, 8, 1143–1150 RSC.
- J. Rodríguez-Carvajal, Recent advances in magnetic structure determination by neutron powder diffraction, Phys. B, 1993, 192, 55–69 CrossRef.
- L. Z. He, Z. Song, Q. C. Xiang, Z. G. Xia and Q. L. Liu, Relationship between thermal quenching of Eu2+ luminescence and cation ordering in (Ba1−xSrx)2SiO4:Eu phosphors, J. Lumin., 2016, 180, 163–168 CrossRef CAS.
- H. S. Jang, Y.-H. Won, S. Vaidyanathan, D. H. Kim and D. Y. Jeon, Emission Band Change of (Sr1−xMx)3SiO5:Eu2+(M = Ca, Ba) Phosphor for White Light Sources Using Blue/Near-Ultraviolet LEDs, J. Electrochem. Soc., 2009, 156, J138–J142 CrossRef CAS.
- Z. Song and Q. L. Liu, Understanding Abnormal Spectral Unshift with Cation Substitution in Highly Efficient Phosphor La3Si6N11:Ce3+, Phys. Chem. Chem. Phys., 2020, 22, 14162–14168 RSC.
- T. Wang, Q. C. Xiang, Z. G. Xia, J. Chen and Q. L. Liu, Evolution of Structure and Photoluminescence by Cation Cosubstitution in Eu2+-Doped (Ca1–xLix)(Al1–xSi1+x)N3 Solid Solutions, Inorg. Chem., 2016, 55, 2929–2933 CrossRef CAS.
- D. P. Cui, Z. Song, Z. G. Xia and Q. L. Liu, Luminescence tuning, thermal quenching, and electronic structure of narrow-band red-emitting nitride phosphors, Inorg. Chem., 2017, 56, 11837–11844 CrossRef CAS.
- F. Clabau, X. Rocquefelte, S. Jobic, P. Deniard, M.-H. Whangbo, A. Garcia and T. Le Mercier, Mechanism of phosphorescence appropriate for the long-lasting phosphors Eu2+-doped SrAl2O4 with codopants Dy3+ and B3+, Chem. Mater., 2005, 17, 3904–3912 CrossRef CAS.
- K. Van den Eeckhout, A. J. Bos, D. Poelman and P. F. Smet, Revealing trap depth distributions in persistent phosphors, Phys. Rev. B: Condens. Matter Mater. Phys., 2013, 87, 045126 CrossRef.
- Z. Y. He, X.-J. Wang and W. M. Yen, Investigation on charging processes and phosphorescent efficiency of SrAl2O4: Eu, Dy, J. Lumin., 2006, 119, 309–3136 CrossRef.
- S. X. Wang, X. L. Liu, B. Y. Qu, Z. Song, Z. Z. Wang, S. Y. Zhang, F. X. Wang, W.-T. Geng and Q. L. Liu, Green persistent luminescence and the electronic structure of β-Sialon:Eu2+, J. Mater. Chem. C, 2019, 7, 12544–12551 RSC.
- Y. X. Zhuang, L. Wang, Y. Lv, T. L. Zhou and R. J. Xie, Optical data storage and multicolor emission readout on flexible films using deep-trap persistent luminescence materials, Adv. Funct. Mater., 2018, 28, 1705769 CrossRef.
Footnote |
† Electronic supplementary information (ESI) available: Commercial cold-light persistent phosphors with excellent performance (Fig. S1); low temperature PL emission spectra under 254 nm excitation of Sr3SiO5:Eu2+,Nb5+ (Fig. S2); and detailed fitting results using the first-order kinetic function of three typical TL glow curves (Fig. S3). The excitation spectra in the VUV-UV range of (Sr1−xBax)3SiO5:Eu2+,Nb5+ (x = 0–0.5) samples (Fig. S4). See DOI: 10.1039/d0qm00488j |
|
This journal is © the Partner Organisations 2021 |
Click here to see how this site uses Cookies. View our privacy policy here.