DOI:
10.1039/D0QI00659A
(Research Article)
Inorg. Chem. Front., 2021,
8, 48-58
Activating Co nanoparticles on graphitic carbon nitride by tuning the Schottky barrier via P doping for the efficient dehydrogenation of ammonia-borane†
Received
5th June 2020
, Accepted 20th August 2020
First published on 24th August 2020
Abstract
Herein, a highly active Mott–Schottky nanocatalyst with tunable Schottky barrier was constructed by embedding Co nanoparticles (NPs) into phosphorus-doped graphitic carbon nitrides (PxCN) for the enhanced dehydrogenation of ammonia-borane (AB). Analyses show that P doping can efficiently decrease the work function of g-C3N4 and elevate the conduction band edge potential; thus, P doping offers a simple approach to tune the Schottky barrier of Co/PxCN by varying the doping amount of P atoms. Upon rationally tuning the Schottky barrier from 0.60 to 0.78 eV, the obtained photocatalyst with the molar composition Co/P3.59CN showed a remarkable TOF (total turnover frequency) value of 67.09 mol H2 per mol metal per min for the dehydrogenation of AB under visible light at 298 K, which, to the best of our knowledge, is one of the highest values reported to date for non-noble metal-based monometallic catalysts and is even comparable to those of some noble metal catalysts. This study opens a new prospect for the rational design and preparation of metal/g-C3N4 nanocatalysts with tunable Schottky barriers and provides a simple way to elevate the catalytic activity of these nanocatalysts.
Introduction
In recent years, the rapidly increasing energy demands have necessitated the development of alternative energy sources that are clean and renewable.1 Hydrogen has been considered as an ideal candidate for clean energy production because it possesses a higher gravimetric energy density than petroleum (120 kJ g−1 for hydrogen vs. 44 kJ g−1 for petroleum) and generates water as the only by-product.2,3 The key point of the so-called “hydrogen-based economy” is to find a safe and efficient hydrogen storage medium.4,5 Various complex hydrides, such as borane-nitrogen (BN) and ammonia-borane (NH3BH3, AB) compounds,6,7 have been investigated for hydrogen storage. Ammonia-borane has attracted significant attention due to its relatively high hydrogen content (19.6 wt%) and a low molecular weight (30.7 g mol−1).2,8,9 AB can release three equivalents of hydrogen quickly when approprite catalysts are used under mild conditions. Thus, efficient catalysts for the dehydrogenation of AB are highly desired.
However, to date, the traditional catalysts used for the hydrolytic dehydrogenation of AB are mostly based on noble metals such as Pt, Au, and Pd. Although they can exhibit excellent catalytic activity, their high cost limits their practical applications in large scale.10–13 Thus, the development of catalysts based on non-noble metals with high efficiency for the dehydrogenation of AB is highly required. Previously, a series of studies have been reported on non-noble metal, including Cu, Co, and Ni, catalysts;3,14–22 among them, Co-based catalysts have recently attracted substantial attention because of their low cost and relatively high catalytic activity.23–25
The current studies are mainly focused on the catalysts based on ultrafine metal nanoparticles (NPs), which contribute to the catalytic activity because their high surface areas can provide abundant active sites. However, the agglomeration of Co NPs due to their high surface energy may reduce the catalytic activity of the corresponding catalyst.26 An efficient strategy to inhibit the agglomeration of these NPs is the dispersion of Co NPs on a high-surface-area support.6 More importantly, Mott–Schottky rectification occurred when a proper semiconductor was used as a support, which could efficiently enhance the electron density of Co NPs. Due to the difference between the work functions of the Co NPs and semiconductor support (exemplified using an n-type semiconductor here), the electrons migrate from the semiconductor support to the Co NPs; this makes the Co NPs more “noble” until the Fermi level on both sides of the interface reaches an equilibrium. This Mott–Schottky rectification between the Co NPs and the semiconductor support mainly depends on the band structure of the semiconductor.27 Thus, a potential barrier, which is called Schottky barrier, is generated at the interface of the semiconductor support and the Co NPs.28,29 As the Schottky barrier can restrict the backward transfer of electrons, it plays a key role in enhancing the separation efficiency of the photo-generated electron/hole pairs. Therefore, the electron density of the Co NPs is enriched and the catalytic activities are promoted.
Among the various semiconductors available for embedding the metal NPs, graphitic carbon nitride (g-C3N4) has been considered as an ideal candidate due to its suitable band structure, where the Fermi level of most of the metals is located between the conduction band and valence band of g-C3N4.27,30–33 Although several heterogeneous catalysts constructed by non-noble metal NPs and g-C3N4 have been investigated for the dehydrogenation of AB,3,34–36 their catalytic activities are still not sufficient.14,23 In principle, rationally tuning the band structure of g-C3N4 could be an effective approach to increase the Schottky barrier of Mott–Schottky heterojunctions, causing enrichment of the electron density of metal NPs. Doping g-C3N4 with heteroatoms, such as B, P, S, I, and F, has been proved to be an efficient way to modify the electron structure of g-C3N4.37–41 However, only few studies have been reported on the relationship between the heterogeneous atoms doped into g-C3N4 and Schottky barrier, especially on the impact of heteroatom doping on the catalytic activities.
Herein, based on that consideration, we constructed a Mott–Schottky interface between the Co NPs and phosphorus-doped graphitic carbon nitrides (PxCN) for the efficient dehydrogenation of AB under visible light, and the Schottky barrier of Co/PxCN was rationally tuned by adjusting the doping content of the P atoms. In our study, we found that P doping can decrease the work function, narrow the bandgap, and elevate the conduction band edge potential of g-C3N4; thus, P doping offers an efficient method to tune the Schottky barrier of Co/PxCN. The Schottky barrier of Co/PxCN can be enlarged from 0.60 eV to 0.78 eV by varying the doping amount of P atoms. The obtained photocatalyst with the molar composition Co/P3.59CN shows a remarkable TOF (total turnover frequency) value of 67.09 mol H2 per mol metal per min for the dehydrogenation of AB under visible light at 298 K. This work provides new insights into the control of the catalytic activity of the catalysts based on metal nanoparticles embedded into a semiconductor support. By rationally tuning the Schottky barrier of metal/g-C3N4 nanocatalysts, the catalytic activity of heterojunctions was optimized using a small doping amount of atoms.
Experimental
Materials
Melamine (C3H6N6, Sinopharm Chemical Reagent Co. Ltd, >99%), sodium borohydride (NaBH4, Sinopharm Chemical Reagent Co. Ltd, 96%), ethylene glycol (C2H6O2, Sinopharm Chemical Reagent Co. Ltd, >99%), 1-hydroxyethylidene-1,1-diphosphonic acid (C2H8O7P2, HEDP, Meryer Chemical Technology Co. Ltd, >99%), cobalt chloride hexahydrate (CoCl2·6H2O, Tansoole, ≥99%), and ammonia-borane (NH3BH3, AB, Macklin, 97%) were used as received without further purification. Deionized water (DI) was utilized in all the experimental processes.
Synthetic procedures
Synthesis of PxCN.
Phosphorus-doped graphitic carbon nitride was obtained according to the method previously reported in the literature.42 Melamine (4.8 g) and HEDP in different amounts (0.0, 0.2, 0.4, 0.6, and 0.8 g) were dissolved in a 64 ml mixed solution of DI water and ethylene glycol (with a volume ratio of 15
:
1) at 80 °C. After removing the solution, the mixed powder was dried overnight at 60 °C under vacuum conditions; then, the resultant mixture was calcined at 550 °C for 4 hours in air. Finally, P-doped g-C3N4 was obtained after cooling the product down to room temperature. The results of Inductively coupled plasma emission spectroscopy (ICP) analyses indicate that the percentages of P atoms in PxCN are 0.0%, 2.16%, 3.59%, 5.26%, and 8.49% (Table S1 in the ESI†). In order to express the doping amount of P atoms, the resulting samples were labelled as PxCN, where x (x = 0, 2.16, 3.59, 5.26, and 8.49) refers to the percentage of the P atoms. Energy dispersive X-ray spectroscopy elemental mapping images suggest that the P atoms are well dispersed in g-C3N4 (Fig. S1 and S2†).
Synthesis of Co/PxCN.
Typically, 9 mg of the as-prepared PxCN and 0.017 mmol of CoCl2·6H2O were dispersed in 5 mL DI water under magnetic stirring for 24 h. Then, 2 mL of a freshly prepared NaBH4 aqueous solution (containing 0.017 mmol NaBH4) was added to the as-prepared solution. After centrifugation, the catalyst was washed with DI water and dried in vacuum at 60 °C overnight. The as-obtained black solid samples were used for further characterizations.
Characterization
Powder X-ray diffraction (XRD) measurements were carried out by Rigaku D/max-2600PC. Fourier transform infrared spectroscopy (FTIR) was conducted using Thermo Fisher Nicolet 6700. X-ray photoelectron spectroscopy (XPS) spectra were obtained via Kratos AXIS Ultra DLD. UV-visible absorption spectra were acquired using PerkinElmer Lambda 950. The energy dispersive X-ray spectroscopy (EDS) analysis and elemental mapping were performed using Hitachi S-4800. Inductively coupled plasma emission spectroscopy (ICP) analyses were conducted via Varian ICP 710.
Electrochemical measurements
The electrochemical measurements were carried out in a conventional three-electrode cell using the CHI 760E workstation as an electrochemical analyzer. A Pt wire and a Ag/AgCl electrode were used as the counter electrode and the reference electrode, respectively. Catalyst powder in a certain amount was dispersed in distilled water containing ethanol and Nafion solution. Subsequently, blending and sonication were conducted to obtain a homogeneous slurry. The working electrode was obtained by dropwise adding the slurry on a glassy carbon electrode. Herein, 0.1 M KOH aqueous solution without any additive was used as an electrolyte. Mott–Schottky plots were obtained at the frequencies of 500, 750, and 1000 Hz in the bias potential range from 1.0 to −1.0 V.
Catalytic activity measurements
The catalytic activity of the hybrid catalysts for the dehydrogenation of AB was measured using a classic water-filled gas burette. Typically, 9 mg of substrate (PxCN), 0.017 mmol of CoCl2·6H2O, and 3 ml of DI water were filled in a two-necked jacketed round-bottom flask under vigorous stirring. After this, 0.85 mmol AB and 0.017 mmol NaBH4 dissolved in 2 ml DI water were quickly added to the flask (the molar ratio of AB and Co is nAB
:
nCo = 50
:
1, where n means the mole number). The molar ratio of AB/NaBH4 is 50
:
1; thus, the hydrogen gas generated by NaBH4 can be ignored in this system. The reactor was irradiated by a 300 W Xe lamp with a 420 nm cutoff filter. The volume of the generated hydrogen gas was quantified by the gas burette. To get the apparent activation energy (Ea) of the hydrolysis of AB catalyzed by the nanocatalysts, the catalytic performance was investigated at different temperatures (288, 293, 298, and 303 K), which was thermostated by a water bath. The total turnover frequency (TOF) value was calculated by the following equation:
where nAB and nmetal refer to the mole number of NH3BH3 and Co, respectively, and t refers to the reaction time (min).
Results and discussion
Direct evidences for the formation of P-doped g-C3N4 (PxCN) were obtained by XRD and FTIR (Fig. 1). The XRD patterns of all samples exhibit two distinct diffraction peaks at 13.0° (100) and 27.3° (002) (Fig. 1a), which could be ascribed to the basic crystal structure of g-C3N4 (JCPDS card no. 87-1526).39 The strong peak at 27.3° corresponds to the (002) interlayer stacking, whereas the peak at 13.0° is ascribed to the (100) in-plane ordering of tri-s-triazine units. The intensity of both peaks decreased with an increase in the amount of P atoms; this indicates that the doping of P atoms increased the disturbance of the graphitic-like structure and significantly decreased the crystallinity of g-C3N4.40 Especially, the (002) peak of P5.26CN and P8.49CN exhibits an obvious left shift when compared with that of P0CN, suggesting that the doping of P atoms loosened the interlayer stacking system and slightly increased the distance between layers.43,44 The molecular structure of PxCN was investigated by the FTIR spectra. As can be observed from Fig. 1b, the characteristic IR spectra of P2.16CN, P3.59CN, P5.26CN, and P8.49CN are similar to that of P0CN. The broad peaks between 3000 and 3500 cm−1 are attributed to N–H stretching, whereas the peaks in the 1200–1600 cm−1 region correspond to the typical stretching modes of CN heterocycles, and the sharp peak at around 803 cm−1 is related to triazine units.45,46 A new peak was observed at 950 cm−1 after P doping. It is presumed that this peak originates from P–N stretching, suggesting that the P atoms have successfully squeezed into the triazine units of g-C3N4 and probably substitute the C atoms to form P–N bonding (Fig. 1c).42,47
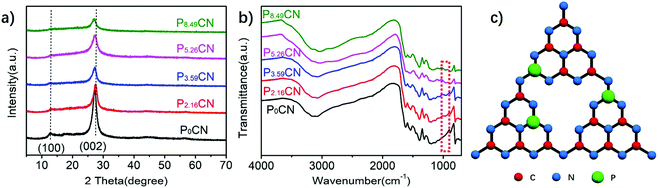 |
| Fig. 1 (a) XRD patterns and (b) FTIR spectra of P0CN, P2.16CN, P3.59CN, P5.26CN, and P8.49CN. (c) Schematic of the structure of PxCN. | |
To explore the modification of the electronic structure of g-C3N4 caused by the doping of P atoms, X-ray photoelectron spectroscopy (XPS) was performed. As shown in Fig. 2a, the XPS survey spectrum of P0CN exhibits three peaks, which are ascribed to C 1s, N 1s, and O 1s. The C 1s spectrum exhibits two remarkable peaks centered at 284.8 eV and 288.2 eV, which could be assigned to graphitic carbon (C–C) and sp2-hybridized carbon in the N-containing triazine ring (N–C
N), respectively. The N 1s spectra can be deconvoluted into three peaks located at 398.6 eV, 399.8 eV, and 401.0 eV. These peaks originated from the sp2-hybridized nitrogen in the triazine rings (C–N
C), tertiary nitrogen N–(C)3 groups, and amino functions carrying hydrogen (C–N–H). The XPS survey spectrum of P3.59CN is similar to that of P0CN, except for the weak signals of the P atoms. The P 2p peak of P3.59CN centered at 133.4 eV is ascribed to the P–N bonding38 (P–C bonding is ∼1–2 eV lower37), which is in good agreement with the results of the FTIR spectra. From these XPS results, we can again confirm that the P atoms had squeezed into the skeleton and probably replaced the C atoms in g-C3N4. Moreover, it was found that the peaks of N–C
N and C–N
C shifted to lower energies by 0.20 and 0.14 eV after the introduction of the P atoms, respectively; this indicates that the P atoms acted as good electron donors for g-C3N4. That is, the addition of the P atoms could significantly enhance the electron density of the g-C3N4 networks.
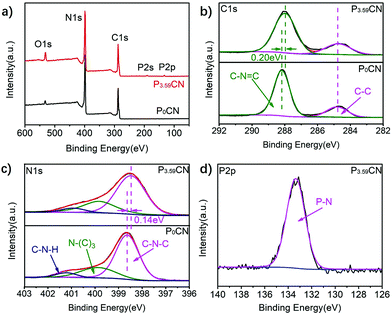 |
| Fig. 2 (a) XPS survey spectra of P0CN and P3.59CN. (b–d) The C 1s, N 1s, and P 2p deconvoluted XPS spectra of P0CN and P3.59CN, respectively. | |
The UV-visible absorption spectra, Mott–Schottky plots, and XPS valence band spectra of PxCN were obtained to further investigate the change in the band structure caused by the modification of the electron structure of g-C3N4. As shown in Fig. 3a, the introduction of the P atoms significantly enhanced the absorption of visible light for g-C3N4, resulting in an ambiguous color change from pale yellow for P0CN to black for P8.59CN (Fig. S3†). Moreover, the band absorption edge of PxCN displays an obvious red shift when compared with that of P0CN. With an increase in the doping content of P atoms, this shift in the band absorption edge increased. Thus, the bandgap gradually decreased from 2.79 eV for P0CN to 2.57 eV, 2.27 eV, 2.24 eV, and 2.22 eV for P2.16CN, P3.59CN, P5.26CN, and P8.49CN, respectively (Fig. 3b). Therefore, we can conclude that the bandgap can be well-tuned by simply controlling the doping content of the P atoms. The flat-band potentials of P0CN, P2.16CN, P3.59CN, P5.26CN, and P8.49CN obtained from the Mott–Schottky plots are −0.73, −0.82, −0.94, −0.97, and −1.01 V vs. Ag/AgCl, respectively (Fig. 4a–e). The flat-band potential against Ag/AgCl can be converted to that against SHE (standard hydrogen electrode) by the following equation:41
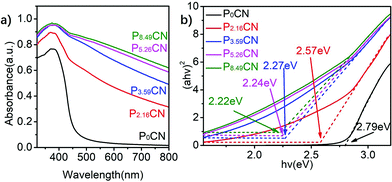 |
| Fig. 3 (a) UV-Visible absorption spectra and (b) band gap energy spectra of P0CN, P2.16CN, P3.59CN, P5.26CN, and P8.49CN. | |
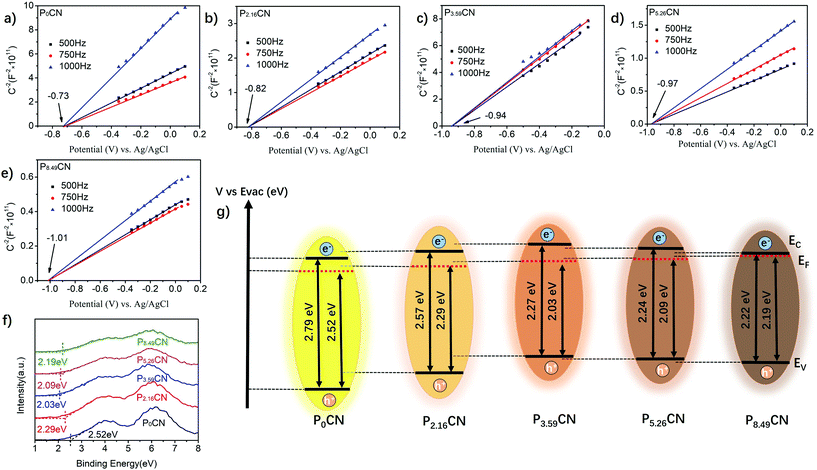 |
| Fig. 4 Mott–Schottky plots of (a) P0CN, (b) P2.16CN, (c) P3.59CN, (d) P5.26CN, and (e) P8.49CN. (f) XPS valence band spectra of P0CN, P2.16CN, P3.59CN, P5.26CN, and P8.49CN. (g) Band structures of P0CN, P2.16CN, P3.59CN, P5.26CN, and P8.49CN (EC: conduction band, EF: Fermi level, and EV: valence band). | |
Therefore, the flat-band potentials of P0CN, P2.16CN, P3.59CN, P5.26CN, and P8.49CN were calculated to be 0.235, 0.145, 0.025, −0.005, and −0.045 V vs. SHE, respectively. According to the reference standard for which 0 V vs. SHE is equal to −4.44 V vs. Evac (vacuum level), the Fermi level (EF) can be calculated by the following equation:48
EF = −Vfb (vs. SHE) × e − 4.44 eV |
Accordingly, the EF values of P0CN, P2.16CN, P3.59CN, P5.26CN, and P8.49CN were calculated to be −4.67, −4.58, −4.46, −4.43, and −4.39 eV, respectively. Hence, it can be observed that the work functions of P-doped g-C3N4 efficiently decreased owing to the enrichment of the electron density of g-C3N4 networks caused by the doped P atoms. The energetic gap between the valence band edge potential and Fermi level was determined by the XPS valence band spectra,48 which was 2.52, 2.29, 2.03, 2.09, and 2.19 eV for P0CN, P2.16CN, P3.59CN, P5.26CN, and P8.49CN, respectively (Fig. 4f). Thus, the valence band edge potentials of P0CN, P2.16CN, P3.59CN, P5.26CN, and P8.49CN were calculated to be −7.19, −6.87, −6.49, −6.52, and −6.58 eV, respectively. In addition to the bandgap energies of P0CN, P2.16CN, P3.59CN, P5.26CN, and P8.49CN deduced from the UV-visible absorption spectra (Fig. 3b), the conduction band edge potentials of P0CN, P2.16CN, P3.59CN, P5.26CN, and P8.49CN were calculated to be −4.40, −4.30, −4.22, −4.28, and −4.36 eV, respectively. In our study, it was found that the decrease in the bandgap slowed down when the doping content of the P atoms was higher than 3.59%, and the elevation of Fermi level also followed this trend when the P loading amount was above 3.59% (Fig. 4g and Table 1). However, the energetic gap between the Fermi level and conduction band edge potential decreased from 0.27 eV for P0CN to 0.28 eV, 0.21 eV, 0.11 eV, and 0.03 eV for P2.16CN, P3.59CN, P5.26CN, and P8.49CN, respectively; this is in good agreement with the previous theory.49 Therefore, the PxCN catalysts acquire highest conduction band edge potential when the doping content of P atoms is 3.59%. When Mott–Schottky heterojunctions were constructed using Co NPs, this elevated conduction band edge potential enlarged the Schottky barrier of Co/PxCN. As a result, we can conclude that the conduction band edge potential, the bandgap, and the work function of PxCN can be well-tuned by simply adjusting the doping amount of the P atoms.
Table 1 The percentages of P atoms and band structures of P0CN, P2.16CN, P3.59CN, P5.26CN, and P8.49CN
Samples |
P atom percentage (%) |
Bandgap (eV) |
Fermi levela (eV) |
Conduction banda (eV) |
Valence banda (eV) |
The potential is against the vacuum level.
|
P0CN |
0 |
2.79 |
−4.67 |
−4.40 |
−7.19 |
P2.16CN |
2.16 |
2.57 |
−4.58 |
−4.30 |
−6.87 |
P3.59CN |
3.59 |
2.27 |
−4.46 |
−4.22 |
−6.49 |
P5.26CN |
5.26 |
2.24 |
−4.43 |
−4.28 |
−6.52 |
P8.49CN |
8.49 |
2.22 |
−4.39 |
−4.36 |
−6.58 |
In order to couple the Co NPs with PxCN, cobalt chloride hexahydrate was in situ reduced by NaBH4 using PxCN as a substrate. As shown in the TEM images (Fig. S4†), the Co NPs are well dispersed on the surface of P3.59CN. The high-resolution TEM (HRTEM) images show that the interlayer spacing in the particle is 0.204 nm, which matches well with the (111) lattice plane of metallic Co.50,51 The formation of metallic Co NPs was again confirmed by the XRD pattern of Co/P3.59CN (ICP analysis indicates that the actual loading amount of Co was 9.52 wt%). A weak and broad peak was observed at 44.2°, which was ascribed to the metallic Co NPs (Fig. S5†). The chemical state of the metallic Co NPs was revealed by the XPS spectra of Co/P3.59CN (Fig. S6†). The Co 2p spectra were deconvoluted into four peaks. The peaks located at 780.8 eV, 782.8 eV, 786.1 eV, and 777.6 eV are ascribed to Co2+, Co3+, shakeup satellites, and metallic Co, respectively.50 Oxidized Co are clearly existed in the sample. However, after the etching treatment, it was found that the intensity of the peak of metallic Co dramatically enhanced; this suggested that metallic Co exists inside the oxidation layer. This implies that an oxidation layer was formed on the surface of the Co NPs, which was beneficial to the stability of Co/P3.59CN in air.52
The Mott–Schottky plots of Co/P0CN, Co/P3.59CN, and Co/P8.49CN were obtained to explore charge rectification and the changes in the Schottky barrier. As shown in Fig. 5, the flat-band potentials of Co/P0CN, Co/P3.59CN, and Co/P8.49CN were calculated to be −0.68, −0.76, and −0.79 V vs. Ag/AgCl, respectively. When these values were compared with those of P0CN, P3.59CN, and P8.49CN (−0.73, −0.94, and −1.01 V vs. Ag/AgCl, respectively), it was found that the flat-band potentials of P0CN, P3.59CN, and P8.49CN exhibited an obvious positive shift after the loading of the metallic Co NPs. This means that the electrons migrate from the PxCN supports to the surface of the Co NPs, leading to the enrichment of the electron density of Co NPs.53 Hence, an interfacial electric field, which is beneficial for the separation of electron/hole pairs at the interface of the Co NPs and PxCN supports, must have generated at the interface of the Co NPs and PxCN, and an upward band bending of the conduction band and valence band must have occurred.54 As the Schottky barrier is related to the work function of the Co NPs (5 eV)55,56 and the conduction band edge potential of PxCN, the Schottky barriers of Co/P0CN, Co/P2.16CN, Co/P3.59CN, Co/P5.26CN, and Co/P8.49CN were calculated to be 0.60, 0.70, 0.78, 0.72, and 0.64 eV, respectively, indicating that the Schottky barrier of Co/PxCN was enlarged by the doping of P atoms. Thus, we can conclude that the Schottky barrier of Co/PxCN can be well-tuned by simply varying the doping amount of the P atoms (Fig. 6). As the Schottky barrier can restrict the backward transfer of electrons, the enlarged Schottky barrier undoubtedly improves the separation efficiency of the photo-generated electron/hole pairs. Thus, more electrons can transfer from the PxCN supports to the Co NPs, leading to the enrichment of the electron density of Co NPs. Finally, the catalytic performance of the Co NPs for the dehydrogenation of AB was remarkably elevated.
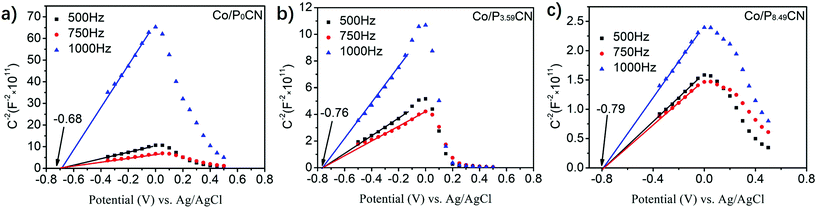 |
| Fig. 5 Mott–Schottky plots of (a) Co/P0CN, (b) Co/P3.59CN, and (c) Co/P8.49CN. | |
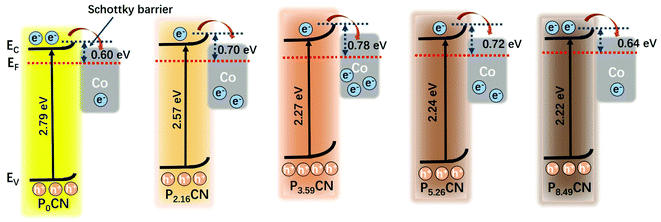 |
| Fig. 6 Band structures and Schottky barriers of Co/P0CN, Co/P2.16CN, Co/P3.59CN, Co/P5.26CN, and Co/P8.49CN (EC: conduction band, EF: Fermi level, and EV: valence band). | |
The catalytic activities of Co/P0CN, Co/P2.16CN, Co/P3.59CN, Co/P5.26CN, and Co/P8.49CN for the dehydrogenation of AB were investigated under visible-light irradiation. To eliminate the thermal influence caused by the irradiation, the temperature was maintained at 298 K by a water bath during the reaction. As can be observed in Fig. 7, the catalytic activity was remarkably promoted after the doping of P atoms. Obviously, Co/P3.59CN possesses the highest catalytic performance in the hydrolysis of AB. Its TOF value is up to 67.09 mol mol−1 min−1 under visible-light irradiation at 298 K, which is one of the highest values achieved to date for non-noble metal-based monometallic catalysts without NaOH treatment and is even comparable to those of some noble metal catalysts (Table 2). It can be observed that the catalytic activities of the nanocatalysts have a positive proportional relationship with the Schottky barrier (Fig. 7b). Therefore, we can conclude that the enhanced photocatalytic activity of Co/P3.59CN may be due to the enlarged Schottky barrier. As discussed above, the introduction of P atoms enriched the electron density of the g-C3N4 networks. Thus, the conduction band edge potential, the bandgap, and the work function of PxCN could be well-tuned by adjusting the doping amount of the P atoms. When the doping amount of the P atoms reached 3.59%, the maximum Schottky barrier of 0.78 eV was acquired for Co/P3.59CN. As a result, more electrons were injected into the surface of the Co NPs, which made the Co NPs more “noble”. Consequently, the catalytic performance of Co/P3.59CN in the hydrolysis of AB was significantly enhanced (Fig. 8).
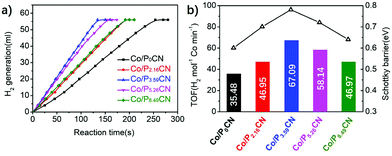 |
| Fig. 7 (a) Hydrogen evolution curves for the AB hydrolysis catalyzed by Co/P0CN, Co/P2.16CN, Co/P3.59CN, Co/P5.26CN, and Co/P8.49CN under visible-light irradiation ([AB] = 170 mM, 5 mL, nCo/nAB = 0.02, and T = 298 K). (b) TOF values of Co/P0CN, Co/P2.16CN, Co/P3.59CN, Co/P5.26CN, and Co/P8.49CN for the AB hydrolysis and the corresponding Schottky barriers. | |
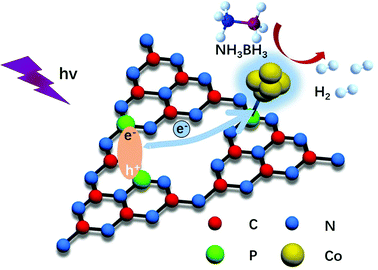 |
| Fig. 8 Schematic of the dehydrogenation of AB catalyzed by Co/P3.59CN under visible-light irradiation. | |
Table 2 TOF values and activation energy values for the hydrolysis of AB catalyzed by different monometallic-based catalysts
Catalysts |
TOF ((H2)mol per (Cat-M)mol per min) |
Activation energy, Ea (kJ mol−1) |
Ref. |
The reaction was promoted by the addition of NaOH.
The reaction was tested under visible-light irradiation.
|
Co/P3.59CN |
67.09b(48.52) |
34.33 |
This work |
Co/g-C3N4 |
55.6b |
— |
23
|
40 wt% Pt/C |
55.56 |
— |
57
|
Co/CTF-1 |
42.3 |
42.7 |
58
|
Ni@3D-(N)GFs |
41.7 |
— |
59
|
Ni2P |
40.4 |
44.6 |
15
|
CuNPs@SCF |
40.0 |
— |
60
|
Co/PEI-GO |
39.9 |
28.2 |
61
|
Ni/ZIF-8 |
35.3(85.7a) |
42.7(28a) |
62
|
Ni@MCS-30 |
30.7 |
— |
16
|
Pd/CN-CS |
27.7 |
35.3 |
34
|
CoP |
24.9(72.2a) |
46.7 |
63
|
Ni/CNT |
23.53 |
— |
64
|
Ni/CN |
18.7b |
36 |
3
|
Co/graphene |
13.8 |
32.75 |
65
|
Ni@MCM-41 |
9.3 |
— |
16
|
Ni NPs/C |
8.8 |
28 |
66
|
Co@C–N@SiO2-800 |
8.4 |
36.1 |
67
|
Visible light can be used to promote the catalytic performance of Co/PxCN in the AB hydrolysis. As shown in Fig. 9a, the evolution rate of hydrogen under the visible-light irradiation is substantially faster than that without irradiation in the AB hydrolysis catalyzed by Co/P3.59CN. This is because visible-light irradiation can excite the P-modified carbon nitride support and inject more electrons into the Co NPs.
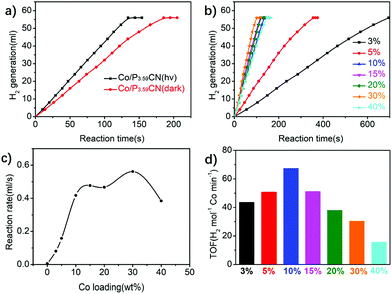 |
| Fig. 9 (a) Hydrogen evolution curves for the AB hydrolysis catalyzed by Co/P3.59CN (10 wt%) with and without visible-light irradiation ([AB] = 170 mM, 5 mL, nCo/nAB = 0.02, and T = 298 K). (b) Hydrogen evolution curves for the AB hydrolysis catalyzed by Co/P3.59CN with different Co loading amounts in the range from 3 wt% to 40 wt% under visible-light irradiation and the corresponding (c) reaction rates and (d) TOF values ([AB] = 170 mM, 5 mL, and T = 298 K). | |
The catalytic activity of Co/P3.59CN with different loading amounts of Co ranging from 3 wt% to 40 wt% was evaluated to investigate the effect of Co loading on the catalytic activity of Co/P3.59CN. As can be observed from Fig. 9b, the catalytic activities gradually increased with an increase in the loading amount of Co up to 30 wt%. However, a further increase in the Co loading amount to 40 wt% led to poor catalytic activity (Fig. 9c). This is most likely because limited sites are available for building an effective metal–semiconductor contact on the surface of P3.59CN.27 Although Co/P3.59CN acquired the best catalytic activity at the Co loading amount of 30 wt%, the optimum catalytic performance with the highest TOF was achieved at the Co loading amount of 10 wt% (Fig. 9d).
The catalytic performance of Co/P3.59CN at different temperatures (288–303 K) was evaluated to study the reaction kinetics of AB hydrolysis. As shown in Fig. 10a, the decomposition time for Co/P3.59CN at 288 K is 199 s, whereas the decomposition time at 303 K is 96 s. This means that the hydrogen generation rate accelerated with an increase in temperature. This result indicates the vital role of temperature in the dehydrogenation of AB. The apparent activation energy (Ea) of the hydrolysis of AB was obtained by the following Arrhenius equation:58
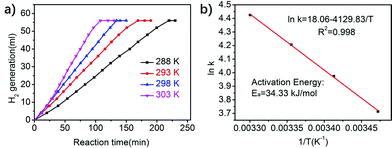 |
| Fig. 10 (a) Hydrogen evolution curves and (b) Arrhenius plots for AB hydrolysis catalyzed by Co/P3.59CN at different temperatures in the range of 288–303 K ([AB] = 170 mM, 5 mL, and nCo/nAB = 0.02). | |
The Arrhenius plots of ln
k vs. the inverse absolute temperature (1/T) are shown in Fig. 10b. From the equation, the Ea value for Co/P3.59CN was calculated to be 34.33 kJ mol−1, which is relatively low as compared to those of the other non-noble metal-based monometallic catalysts for AB hydrolysis (Table 2).
Since the stability and reusability of the nanocatalysts are also important factors for the practical application of these nanocatalysts, the recycling test of Co/P3.59CN was conducted under visible-light irradiation at 298 K. As shown in Fig. 11, the catalysts exhibited high catalytic activity even after 8 runs, and the volume of the hydrogen generated by AB remained unchanged; this suggested good stability and recyclability of Co/P3.59CN. The TEM images and XRD patterns of Co/P3.59CN exhibit no obvious change after eight cycles (Fig. S7†), again demonstrating the high stability of Co/P3.59CN.
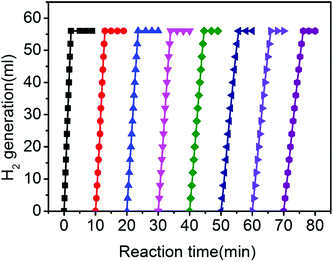 |
| Fig. 11 Hydrogen evolution curves for AB hydrolysis catalyzed by Co/P3.59CN during an eight-cycle reusability test ([AB] = 170 mM, 5 mL, nCo/nAB = 0.02, and T = 298 K). | |
Conclusions
In conclusion, herein, we developed a simple method to tune the Schottky barrier of Co/PxCN by varying the doping amount of P atoms for the efficient dehydrogenation of AB. By simply adjusting the doping amount of P atoms from 0 to 3.59%, the Schottky barrier could be controlled from 0.60 eV to 0.78 eV because the doped P atoms act as good electron donors for g-C3N4, leading to the enrichment of the electron density of g-C3N4 networks. Upon further increasing the doping content of P atoms, the energetic gap between the Fermi level and conduction band edge potential was significantly decreased, resulting in a decrease in the Schottky barrier of Co/PxCN. The maximum TOF value for Co/P3.59CN in the dehydrogenation of AB is up to 67.09 mol mol−1 min−1 under visible-light irradiation at 298 K. To the best of our knowledge, this is one of the highest values achieved to date for non-noble metal-based monometallic catalysts without NaOH treatment and is even comparable to those of some noble metal catalysts. This study provides an easy and feasible method to tune the Schottky barrier of metal/g-C3N4-type Mott–Schottky nanocatalysts and provides us a new vision to facilitate their catalytic activity.
Conflicts of interest
There are no conflicts to declare.
Acknowledgements
This work was supported by the Natural Science Foundation of Shanghai (No. 17ZR1420000), the Shanghai talent development funding for the project (No. 2017044), the International Science & Technology Cooperation Program of Shanghai (No. 18520723300), the Shanghai Science and Technology Commission (19XD1431800), and the China Postdoctoral Science Foundation (No. 2018M642021).
Notes and references
- X. B. Yu, Z. W. Tang, D. L. Sun, L. L. Ouyang and M. Zhu, Recent advances and remaining challenges of nanostructured materials for hydrogen storage applications, Prog. Mater. Sci., 2017, 88, 1–48 CrossRef.
- W. W. Zhan, Q. L. Zhu and Q. Xu, Dehydrogenation of ammonia borane by metal nanoparticle catalysts, ACS Catal., 2016, 6, 6892–6905 CrossRef CAS.
- M. Y. Gao, Y. S. Yu, W. W. Yang, J. Li, S. C. Xu, M. Feng and H. B. Li, Ni nanoparticles supported on graphitic carbon nitride as visible light catalysts for hydrolytic dehydrogenation of ammonia borane, Nanoscale, 2019, 11, 3506–3513 RSC.
- Z. W. Tang, H. Chen, X. W. Chen, L. M. Wu and X. B. Yu, Graphene oxide based recyclable dehydrogenation of ammonia borane within a hybrid nanostructure, J. Am. Chem. Soc., 2012, 134, 5464–5467 CrossRef CAS.
- H. Liu, B. L. Huang, J. H. Zhou, K. Wang, Y. S. Yu, W. W. Yang and S. J. Guo, Enhanced electron transfer and light absorption on imino polymer capped PdAg nanowire networks for efficient room-temperature dehydrogenation of formic acid, J. Mater. Chem. A, 2018, 6, 1979–1984 RSC.
- Y. Y. Cai, X. H. Li, Y. N. Zhang, X. Wei, K. X. Wang and J. S. Chen, Highly efficient dehydrogenation of formic acid over a palladium-nanoparticle-based Mott-Schottky photocatalyst, Angew. Chem., Int. Ed., 2013, 52, 11822–11825 CrossRef CAS.
- Y. H. Guo, X. B. Yu, W. W. Sun, D. L. Sun and W. N. Yang, The hydrogen-enriched Al-B-N system as an advanced solid hydrogen-storage candidate, Angew. Chem., Int. Ed., 2011, 50, 1087–1091 CrossRef CAS.
- A. Staubitz, A. P. M. Robertson and I. Manners, Ammonia-borane and related compounds as dihydrogen sources, Chem. Rev., 2010, 110, 4079–4124 CrossRef CAS.
- B. L. Davis, D. A. Dixon, E. B. Garner, J. C. Gordon, M. H. Matus, B. Scott and F. H. Stephens, Efficient regeneration of partially spent ammonia borane fuel, Angew. Chem., Int. Ed., 2009, 48, 6812–6816 CrossRef CAS.
- J. M. Yan, X. B. Zhang, T. Akita, M. Haruta and Q. Xu, One-step seeding growth of magnetically recyclable Au@Co core-shell nanoparticles: highly efficient catalyst for hydrolytic dehydrogenation of ammonia borane, J. Am. Chem. Soc., 2010, 132, 5326–5327 CrossRef CAS.
- X. G. Li, C. L. Zhang, M. H. Luo, Q. L. Yao and Z. H. Lu, Ultrafine Rh nanoparticles confined by nitrogenrich covalent organic frameworks for methanolysis of ammonia borane, Inorg. Chem. Front., 2020, 7, 1298–1306 RSC.
- M. Y. Gao, W. W. Yang and Y. S. Yu, Monodisperse PtCu alloy nanoparticles as highly efficient catalysts for the hydrolytic dehydrogenation of ammonia borane, Int. J. Hydrogen Energy, 2018, 43, 14293–14300 CrossRef CAS.
- W. Y. Chen, J. Ji, X. Feng, X. Z. Duan, G. Qian, P. Li, X. G. Zhou, D. Chen and W. K. Yuan, Mechanistic insight into size-dependent activity and durability in Pt/CNT catalyzed hydrolytic dehydrogenation of ammonia borane, J. Am. Chem. Soc., 2014, 136, 16736–16739 CrossRef CAS.
- H. Zhang, X. J. Gu, J. Song, N. Fan and H. Q. Su, Non-noble metal nanoparticles supported by postmodified porous organic semiconductors: highly efficient catalysts for visible-light-driven on-demand H2 evolution from ammonia borane, ACS Appl. Mater. Interfaces, 2017, 9, 32767–32774 CrossRef CAS.
- C. Y. Peng, L. Kang, S. Cao, Y. Chen, Z. S. Lin and W. F. Fu, Nanostructured Ni2P as a robust catalyst for the hydrolytic dehydrogenation of ammonia-borane, Angew. Chem., Int. Ed., 2015, 54, 15725–15729 CrossRef CAS.
- P. Z. Li, A. Aijaz and Q. Xu, Highly dispersed surfactant-free nickel nanoparticles and their remarkable catalytic
activity in the hydrolysis of ammonia borane for hydrogen generation, Angew. Chem., Int. Ed., 2012, 51, 6753–6756 CrossRef CAS.
- C. Y. Wang, D. D. Sun, X. F. Yu, X. H. Zhang, Z. M. Lu, X. X. Wang, J. L. Zhao, L. L. Li and X. J. Yang, Cu/Ni nanoparticles supported on TiO2(B) nanotubes as hydrogen generation photocatalysts via hydrolysis of ammonia borane, Inorg. Chem. Front., 2018, 5, 2038–2044 RSC.
- J. G. Yang, Q. Yuan, Y. Liu, X. L. Huang, Y. X. Qiao, J. N. Lu and C. L. Song, Low-cost ternary Ni-Fe-P catalysts supported on Ni foam for hydrolysis of ammonia borane, Inorg. Chem. Front., 2019, 6, 1189–1194 RSC.
- Q. L. Yao, Z. H. Lu, Y. W. Yang, Y. Z. Chen, X. S. Chen and H. L. Jiang, Facile synthesis of graphene-supported Ni-CeOx nanocomposites as highly efficient catalysts for hydrolytic dehydrogenation of ammonia borane, Nano Res., 2018, 11, 4412–4422 CrossRef CAS.
- Q. L. Yao, K. Yang, X. L. Hong, X. S. Chen and Z. H. Lu, Base-promoted hydrolytic dehydrogenation of ammonia borane catalyzed by noble-metal-free nanoparticles, Catal. Sci. Technol., 2018, 8, 870–877 RSC.
- Q. L. Yao, Z. H. Lu, W. Huang, X. S. Chen and J. Zhu, High Pt-like activity of the Ni-Mo/graphene catalyst for hydrogen evolution from hydrolysis of ammonia borane, J. Mater. Chem. A, 2016, 4, 8579–8583 RSC.
- Y. N. Men, J. Su, C. Z. Huang, L. J. Liang, P. Cai, G. Z. Cheng and W. Luo, Three-dimensional nitrogen-doped graphene hydrogel supported Co-CeOx nanoclusters as efficient catalysts for hydrogen generation from hydrolysis of ammonia borane, Chin. Chem. Lett., 2018, 29, 1671–1674 CrossRef CAS.
- H. Zhang, X. J. Gu, P. L. Liu, J. Song, J. Cheng and H. Q. Su, Highly efficient visible-light-driven catalytic hydrogen evolution from ammonia borane using non-precious metal nanoparticles supported by graphitic carbon nitride, J. Mater. Chem. A, 2017, 5, 2288–2296 RSC.
- H. C. Zheng, K. Feng, Y. P. Shang, Z. H. Kang, X. H. Sun and J. Zhong, Cube-like CuCoO nanostructures on reduced graphene oxide for H2 generation from ammonia borane, Inorg. Chem. Front., 2018, 5, 1180–1187 RSC.
- Y. Yuan, X. Y. Chen, X. Zhang, Z. M. Wang and R. B. Yu, A MOF-derived CuCo(O)@ carbon-nitrogen framework as an efficient synergistic catalyst for the hydrolysis of ammonia borane, Inorg. Chem. Front., 2020, 7, 2043–2049 RSC.
- P. P. Ghimire, L. P. Zhang, U. A. Kinga, Q. Y. Guo, B. J. Jiang and M. Jaroniec, Development of nickel-incorporated MCM-41-carbon composites and their application in nitrophenol reduction, J. Mater. Chem. A, 2019, 7, 9618–9628 RSC.
- L. T. Guo, Y. Y. Cai, J. M. Ge, Y. N. Zhang, L. H. Gong, X. H. Li, K. X. Wang, Q. Z. Ren, J. Su and J. S. Chen, Multifunctional Au-Co@CN nanocatalyst for highly efficient hydrolysis of ammonia borane, ACS Catal., 2014, 5, 388–392 CrossRef.
- H. H. Wang, B. Zhang, X. H. Li, M. Antonietti and J. S. Chen, Activating Pd nanoparticles on sol-gel prepared porous g-C3N4/SiO2 via enlarging the Schottky barrier for efficient dehydrogenation of formic acid, Inorg. Chem. Front., 2016, 3, 1124–1129 RSC.
- X. H. Li and M. Antonietti, Metal nanoparticles at mesoporous N-doped carbons and carbon nitrides: functional Mott-Schottky heterojunctions for catalysis, Chem. Soc. Rev., 2013, 42, 6593–6604 RSC.
- A. Vinu, K. Ariga, T. Mori, T. Nakanishi, S. Hishita, D. Golberg and Y. Bando, Preparation and characterization of well-ordered hexagonal mesoporous carbon nitride, Adv. Mater., 2005, 17, 1648–1652 CrossRef CAS.
- G. P. Mane, D. S. Dhawale, C. Anand, K. Ariga, Q. M. Ji, M. A. Wahab, T. Mori and A. Vinu, Selective sensing performance of mesoporous carbon nitride with a highly ordered porous structure prepared from 3-amino-1,2,4-triazine, J. Mater. Chem. A, 2013, 1, 2913–2920 RSC.
- H. Liu, X. Y. Liu, W. W. Yang, M. Q. Shen, S. Geng, C. Yu, B. Shen and Y. S. Yu, Photocatalytic dehydrogenation of formic acid promoted by a superior PdAg@g-C3N4 Mott-Schottky heterojunction, J. Mater. Chem. A, 2019, 7, 2022–2026 RSC.
- Y. J. Wang, S. Y. Bao, Y. Q. Liu, W. W. Yang, Y. S. Yu, M. Feng and K. F. Li, Efficient photocatalytic reduction of Cr(VI) in aqueous solution over CoS2/gC3N4-rGO nanocomposites under visible light, Appl. Surf. Sci., 2020, 510, 145495 CrossRef CAS.
- H. Jia, X. Chen, X. R. Song, X. C. Zheng, X. X. Guan and P. Liu, Graphitic carbon nitride-chitosan composites-anchored palladium nanoparticles as high-performance catalyst for ammonia borane hydrolysis, Int. J. Energy Res., 2018, 43, 535–543 CrossRef.
- C. H. Han, P. Meng, E. R. Waclawik, C. Zhang, X. H. Li, H. Q. Yang, M. Antonietti and J. S. Xu, Palladium/graphitic carbon nitride (g-C3N4) stabilized emulsion microreactor as a store for hydrogen from ammonia borane for use in alkene hydrogenation, Angew. Chem., Int. Ed., 2018, 57, 14857–14861 CrossRef CAS.
- Y. R. Fan, X. J. Li, X. C. He, C. M. Zeng, G. Y. Fan, Q. Q. Liu and D. M. Tang, Effective hydrolysis of ammonia borane catalyzed by ruthenium nanoparticles immobilized on graphic carbon nitride, Int. J. Hydrogen Energy, 2014, 39, 19982–19989 CrossRef CAS.
- Y. J. Zhang, T. Mori, J. H. Ye and M. Antonietti, Phosphorus-doped carbon nitride solid: enhanced electrical conductivity and photocurrent generation, J. Am. Chem. Soc., 2010, 132, 6294–6295 CrossRef CAS.
- W. Liu, L. L. Cao, W. R. Cheng, Y. J. Cao, X. K. Liu, W. Zhang, X. L. Mou, L. Jin, X. S. Zheng, W. Che, Q. H. Liu, T. Yao and S. Q. Wei, Single-site active cobalt-based photocatalyst with a long carrier lifetime for spontaneous overall water splitting, Angew. Chem., Int. Ed., 2017, 56, 9312–9317 CrossRef CAS.
- B. Liu, L. Q. Ye, R. Wang, J. F. Yang, Y. X. Zhang, R. Guan, L. H. Tian and X. B. Chen, Phosphorus-doped graphitic carbon nitride nanotubes with amino-rich surface for efficient CO2 capture, enhanced photocatalytic activity, and product selectivity, ACS Appl. Mater. Interfaces, 2018, 10, 4001–4009 CrossRef CAS.
- J. S. Zhang, M. W. Zhang, G. G. Zhang and X. C. Wang, Synthesis of carbon nitride semiconductors in sulfur flux for water photoredox catalysis, ACS Catal., 2012, 2, 940–948 CrossRef CAS.
- M. Q. Wu, T. Ding, J. M. Cai, Y. T. Wang, H. Xian, H. Zhang, Y. Tian, T. Y. Zhang and X. G. Li, Co-addition of phosphorus and proton to graphitic carbon nitride for synergistically enhanced visible light photocatalytic degradation and hydrogen evolution, ACS Sustainable Chem. Eng., 2018, 6, 8167–8177 CrossRef CAS.
- Y. P. Zhu, T. Z. Ren and Z. Y. Yuan, Mesoporous phosphorus-doped g-C3N4 nanostructured flowers with superior photocatalytic hydrogen evolution performance, ACS Appl. Mater. Interfaces, 2015, 7, 16850–16856 CrossRef CAS.
- P. Niu, L. L. Zhang, G. Liu and H. M. Cheng, Graphene-like carbon nitride nanosheets for improved photocatalytic activities, Adv. Funct. Mater., 2012, 22, 4763–4770 CrossRef CAS.
- X. C. Wang, J. M. Carlsson, K. Domen, K. Maeda, A. Thomas, K. Takanabe, G. Xin and M. Antonietti, A metal-free polymeric photocatalyst for hydrogen production from water under visible light, Nat. Mater., 2009, 8, 76–80 CrossRef CAS.
- M. Shalom, S. Inal, C. Fettkenhauer, D. Neher and M. Antonietti, Improving carbon nitride photocatalysis by supramolecular preorganization of monomers, J. Am. Chem. Soc., 2013, 135, 7118–7121 CrossRef CAS.
- P. X. Qiu, H. Chen, C. M. Xu, N. Zhou, F. Jiang, X. Wang and Y. S. Fu, Fabrication of an exfoliated graphitic carbon nitride as a highly active visible light photocatalyst, J. Mater. Chem. A, 2015, 3, 24237–24244 RSC.
- T. Y. Ma, J. Ran, S. Dai, M. Jaroniec and S. Z. Qiao, Phosphorus-doped graphitic carbon nitrides grown in situ on carbon-fiber paper: flexible and reversible oxygen electrodes, Angew. Chem., Int. Ed., 2015, 54, 4646–4650 CrossRef CAS.
- L. P. Zhang, J. R. Ran, S. Z. Qiao and M. Jaroniec, Characterization of semiconductor photocatalysts, Chem. Soc. Rev., 2019, 48, 5184–5206 RSC.
-
R. F. Pierret, Semiconductor device fundamentals, Addison-Wesley Publishing, New Jersey, 1996, ch. 2 Search PubMed.
- Z. H. Xue, H. Su, Q. Y. Yu, B. Zhang, H. H. Wang, X. H. Li and J. S. Chen, Janus Co/CoP nanoparticles as efficient Mott-Schottky electrocatalysts for overall water splitting in wide pH range, Adv. Energy Mater., 2017, 7, 1602355 CrossRef.
- H. Su, K. X. Zhang, B. Zhang, H. H. Wang, Q. Y. Yu, X. H. Li, M. Antonietti and J. S. Chen, Activating cobalt nanoparticles via the Mott-Schottky effect in nitrogen-rich carbon shells for base-free aerobic oxidation of alcohols to esters, J. Am. Chem. Soc., 2017, 139, 811–818 CrossRef CAS.
- T. J. Zhao, Y. N. Zhang, K. X. Wang, J. Su, X. Wei and X. H. Li, General transfer hydrogenation by activating ammonia-borane over cobalt nanoparticles, RSC Adv., 2015, 5, 102736–102740 RSC.
- Y. Y. Bu, Z. Y. Chen and W. B. Li, Using electrochemical methods to study the promotion mechanism of the photoelectric conversion performance of Ag-modified mesoporous g-C3N4 heterojunction material, Appl. Catal., B, 2014, 144, 622–630 CrossRef CAS.
- Y. Yang, J. Gu, J. L. Young, E. M. Miller, J. A. Turner, N. R. Neale and M. C. Beard, Semiconductor interfacial carrier dynamics via photoinduced electric fields, Science, 2015, 350, 1061–1065 CrossRef CAS.
- D. E. Eastman, Photoelectric work functions of transition, rare-earth, and noble metals, Phys. Rev. B: Condens. Matter Mater. Phys., 1970, 2, 1–2 CrossRef.
- H. B. Michaelson, The work function of the elements and its periodicity, J. Appl. Phys., 1977, 48, 4729–4733 CrossRef CAS.
- Q. Xu and M. Chandra, A portable hydrogen generation system: Catalytic hydrolysis of ammonia-borane, J. Alloys Compd., 2007, 446–447, 729–732 CrossRef CAS.
- Z. Li, T. He, L. Liu, W. D. Chen, M. Zhang, G. T. Wu and P. Chen, Covalent triazine framework supported non-noble metal nanoparticles with superior activity for catalytic hydrolysis of ammonia borane: from mechanistic study to catalyst design, Chem. Sci., 2017, 8, 781–788 RSC.
- M. Mahyari and A. Shaabani, Nickel nanoparticles immobilized on three-dimensional nitrogen-doped graphene as a superb catalyst for the generation of hydrogen from the hydrolysis of ammonia borane, J. Mater. Chem. A, 2014, 2, 16652–16659 RSC.
- M. Kaya, M. Zahmakiran, S. O. zkar and M. R. Volkan, Copper(0) nanoparticles supported on silica-coated cobalt ferrite magnetic particles: Cost effective catalyst in the hydrolysis of ammonia-borane with an exceptional reusability performance, ACS Appl. Mater. Interfaces, 2012, 4, 3866–3873 CrossRef CAS.
- J. T. Hu, Z. X. Chen, M. X. Li, X. H. Zhou and H. B. Lu, Amine-capped Co nanoparticles for highly efficient dehydrogenation of ammonia borane, ACS Appl. Mater. Interfaces, 2014, 6, 13191–13200 CrossRef CAS.
- C. L. Wang, J. Tuninetti, Z. Wang, C. Zhang, R. Ciganda, L. Salmon, S. Moya, J. Ruiz and D. Astruc, Hydrolysis of ammonia-borane over Ni/ZIF-8 nanocatalyst: high efficiency, mechanism and controlled hydrogen release, J. Am. Chem. Soc., 2017, 139, 11610–11615 CrossRef CAS.
- Z. C. Fu, Y. Xu, S. L. Chan, W. W. Wang, F. Li, F. Liang, Y. Chen, Z. S. Lin, W. F. Fu and C. M. Che, Highly efficient hydrolysis of ammonia borane by anion (−OH, F−, Cl−)-tuned interactions between reactant molecules and CoP nanoparticles, Chem. Commun., 2017, 53, 705–708 RSC.
- G. Q. Zhao, J. Zhong, J. Wang, T. K. Sham, X. H. Sun and S. T. Lee, Revealing the synergetic effects in Ni nanoparticle-carbon nanotube hybrids by scanning transmission X-ray microscopy and their application in the hydrolysis of ammonia borane, Nanoscale, 2015, 7, 9715–9722 RSC.
- L. Yang, N. Cao, C. Du, H. M. Dai, K. Hu, W. Luo and G. Z. Cheng, Graphene supported cobalt(0) nanoparticles for hydrolysis of ammonia borane, Mater. Lett., 2014, 115, 113–116 CrossRef CAS.
- Ö. Metin, V. Mazumder, S. Özkar and S. H. Sun, Monodisperse nickel nanoparticles and their catalysis in hydrolytic dehydrogenation of ammonia borane, J. Am. Chem. Soc., 2010, 132, 1468–1469 CrossRef.
- M. L. Chen, R. Xiong, X. Cui, Q. Wang and X. W. Liu, SiO2 encompassed Co@N-Doped porous carbon assemblies as recyclable catalysts for efficient hydrolysis of ammonia borane, Langmuir, 2019, 35, 671–677 CrossRef CAS.
Footnote |
† Electronic supplementary information (ESI) available: TEM, XPS and EDS elemental mapping images. See DOI: 10.1039/d0qi00659a |
|
This journal is © the Partner Organisations 2021 |
Click here to see how this site uses Cookies. View our privacy policy here.