DOI:
10.1039/D1PY00534K
(Paper)
Polym. Chem., 2021,
12, 4359-4371
Oxazoline-methacrylate graft-copolymers with upper critical solution temperature behaviour in Yubase oil†
Received
20th April 2021
, Accepted 27th May 2021
First published on 28th May 2021
Abstract
Thermoresponsive behavior of polymers in aqueous solutions has been widely studied and utilized in various applications. However, the fundamental understanding on the use of oil soluble polymers in non-aqueous solutions is very limited. Herein, we report the synthesis of linear homo and copolymers as well as graft-copolymers based on 2-oxazoline monomers and the investigation of their solution behavior and thermal properties via turbidity measurements and thermal analyses (DSC and TGA). The homo and copolymers were synthetized via living cationic ring-opening polymerization (CROP) of 2-stearyl-2-oxazoline (SteOx) and 2-ethyl-2-oxazoline (EtOx) and their composition was varied in order to tune the thermoresponsive behavior in a commercially available base oil (Yubase-4). The graft-copolymers were obtained using the grafting-onto method. The backbone composed of a methacrylic acid and 2-ethylhexyl methacrylate copolymer was synthetized via reversible addition–fragmentation chain-transfer (RAFT) polymerization, while side chains consisting of SteOx–EtOx copolymers were obtained via CROP. Various polymerization parameters were evaluated in order to optimize the grafting efficiency and the solubility in oil of the synthetized graft-copolymers. It was seen that not only the overall hydrophobicity of the polymer chains but also the overall order of the system have significant effects on the upper critical solution temperature (UCST) in oil. Finally, a relationship between the crystallization temperature measured using DSC and the transition temperature (Ttrans) values obtained from the turbidity measurements was compared.
Introduction
Living cationic ring-opening polymerization (CROP) of 2-oxazolines was first reported in 1966 by four independent research groups.1–4 Recently, 2-oxazoline monomers and their corresponding poly(2-alkyl-2-oxazolines)s have gained an increasing interest for the development of high-performance polymeric materials especially for biomedical applications.5–8 Under optimized reaction conditions, the CROP of 2-oxazolines can proceed in a living or quasi-living fashion, following the typical chain-growth polymerization mechanism.9
The living nature of the polymerization allows the synthesis of well-defined homopolymers, but also of well-defined random and block copolymers depending on the reactivity of the 2-oxazoline monomers.10–12 Additionally, by varying the substituent on the 2-position of the 2-oxazoline ring, the structure and physical properties of poly(2-alkyl-2-oxazoline)s can be precisely tuned and adjusted depending on the desired application.13 In addition to linear (co)polymers, different architectures obtained via copolymerization with other monomers have been described in literature.14–16 However, graft architectures based on poly(2-oxazoline)s are rarely reported. Most previous examples have mainly utilized the grafting-through method, in which living oxazolinium species are end-capped with a (meth)acrylate and then polymerized with other monomers.17–21 Examples of graft-copolymers obtained via grafting-from,22–24 and grafting-onto approach are also reported in the literature.19,25–28
The utilization of living polymerization techniques enables many advantages, such as good control over the molecular weight, controlled dispersity values and macromolecular architecture, well-defined end groups, and the possibility to easily access block copolymers.29 Comparatively, a large variety of monomers can be (co)polymerized with conventional free radical polymerization due to simple reaction conditions and high tolerance towards functional groups. However, well-defined (co)polymers cannot be obtained via free radical polymerization due to the occurrence of termination reactions.30 Therefore, the development of processes combining both the advantages of living polymerizations and the versatility of free radical polymerization has been one of the major goals in the polymer chemistry field.31 Among all, reversible addition–fragmentation chain-transfer (RAFT) polymerization is considered one of the most powerful and versatile methods to provide living character to radical polymerizations.32 For instance, RAFT polymerization is often preferred because of its easy reaction conditions and high functional group tolerance, which allow the polymerization of a wide variety of monomers over a broad range of temperatures and in a large choice of solvents.33 Therefore, the combination of CROP and RAFT polymerization techniques provides a powerful tool to obtain well-defined polymers based on poly(2-alkyl-2-oxazoline)s and RAFT monomers with precise architectures.34–37
The ability of combining hydrophilic and hydrophobic monomers into well-defined copolymers with specific architectures opens a variety of potential applications, especially for their amphiphilic character, which results in self-assembly in solution into nanoscale-size objects.38 It is well known that the self-assembly behaviour can also be triggered by an external stimulus.39 Among all, polymers that respond to temperature are gaining special attention due to their potential applications.40,41 However, the temperature-responsive behaviour of linear (co)polymers and (co)polymers with more complex architectures has been studied especially in pure water or in alcohol/water mixtures. For instance, only a limited amount of studies report polymers with thermoresponsiveness in non-aqueous media. Examples of random, block, and graft copolymers based on methacrylates and acrylates exhibiting thermoresponsiveness in alkanes and oils have already been described in literature.42–49 Also in the case of poly(2-alkyl-2-oxazoline)-based materials, their thermoresponsive behaviour has been evaluated exclusively in pure water or in water/alcohol mixtures. Their lower critical solution temperature (LCST) behaviour can be varied and precisely controlled by copolymerizing 2-oxazoline monomers with different alkyl side-chain length, varying the hydrophilicity/hydrophobicity ratios.50–53 More complex architectures have also been studied in order to enhance the properties of the final material and have a better control over the temperature range of the phase transition.19,23,34,54,55 However, despite the large amount of work on 2-oxazoline polymers showing an LCST behaviour, only a limited number of poly(2-alkyl-2-oxazoline)s is reported to show a upper critical solution temperature (UCST) phase transition, which only occurs in alcohol/water mixtures.52,56–58
In this study, linear homo and copolymers of 2-stearyl-2-oxazoline (SteOx) and 2-ethyl-2-oxazoline (EtOx) are synthesized via living CROP resulting in well-defined copolymers with varying oil solubility behaviour. Moreover, graft-copolymers consisting of a methacrylic acid and 2-ethylhexyl methacrylate random copolymer backbone (poly(xMA)) obtained via RAFT polymerization, and SteOx–EtOx random copolymer side chains (polyOx) are synthetized using the grafting-onto method (Scheme 1). Their solubility behaviour in a commercially available base oil (i.e. Yubase-4) and their thermal properties are evaluated using turbidity measurements and thermal analyses, respectively. To the best of our knowledge, this is the first report of thermoresponsive linear copolymers and graft-copolymers based on 2-oxazoline and methacrylate monomers showing a UCST-type phase transition in pure non-aqueous systems.
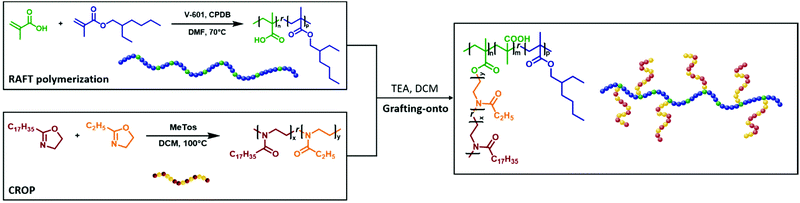 |
| Scheme 1 Schematic depiction of the synthetic route used to synthesize graft-copolymers. RAFT polymerization of methacrylic acid and 2-ethylhexyl methacrylate (top left), CROP of 2-stearyl-2-oxazoline and 2-ethyl-2-oxazoline (bottom left), and grafting-onto reaction (right). For brevity, the end-groups of copolymers are not shown. | |
Experimental section
Materials
2-Ethyl-2-oxazoline (99+%, Acros Organics, EtOx) was dried over calcium hydride and distilled under reduced pressure prior to use. Methyl tosylate (98%, Aldrich, MeTos) was distilled under reduced pressure and stored under nitrogen. Triethylamine (≥99%, Sigma Aldrich, TEA) was distilled and stored under nitrogen. The used extra dry solvents dichloromethane (99.8%, DCM) and chlorobenzene (99.8%) were purchased from Acros Organics and stored over molecular sieves and under inert atmosphere. Titanium(IV) n-butoxide (99%), ethanolamine, and 3-amino-1-propanol (99%) were purchased from Acros Organics and used as received. The initiator V-601 was purchased from Fujifilm Wako Chemicals Corporation and used as received. The monomers methacrylic acid (99%, Aldrich) and 2-ethylhexyl methacrylate (99%, Acros Organics), and the transfer agent 2-cyano-2-propyl benzodithioate (>97%, Aldrich, CPBD) were used as received. Sodium methoxide (95%, NaOMe) and stearic acid (95%) were purchased from Sigma-Aldrich and used as received. N,N-Dimethylformamide (≥99%, DMF) was purchased from Fisher Chemical.
Synthesis of 2-stearyl-2-oxazoline (SteOx) monomer.
Stearic acid (1.00 eq.) was dissolved in MeOH (30 eq.) in a 500mL round bottom flask equipped with a magnetic stirring bar. Sulfuric acid (0.007 eq.) was then added and the reaction mixture was stirred at 85 °C under reflux overnight. The temperature was then decreased and the cooling water was kept running until RT was reached. Solid NaHCO3 was added slowly, until no release of gas was observed. The solvent was removed in vacuo, and the resulting methyl stearate was used as obtained. Subsequently, ethanolamine (4 eq.), and sodium methoxide (3 mol%) were added to the round bottom flask, which was then placed in an oil bath and heated up to 120 °C overnight. After completion of the amination, the reaction mixture was cooled down to 90 °C and distilled under reduced pressure from 90 up to 160 °C. When 160 °C were reached, the solution was kept at this temperature for 15 minutes before adding the titanium(IV) n-butoxide (0.14 eq.). The reaction mixture was then stirred at 160 °C overnight under reduced pressure. The resulting 2-oxazoline was obtained from distillation under reduced pressure of the crude mixture at temperatures higher than 250 °C. A white solid was obtained in 50–65% yield.
1H NMR (300 MHz, CDCl3), δ (ppm): 0.74–0.85 (m, 3H, CH2CH3), 1.11–1.31 (m, 28H, CH2 alkyl chain), 1.48–1.60 (m, 2H, CCH2CH2), 2.19 (t, 2H, CCH2CH2), 3.75 (t, 2H, CH2CH2O), 4.14 (t, 2H, NCH2CH2).
Synthesis of 2-alkyl-2-oxazoline random copolymers via CROP (polyOx).
All copolymers were synthesized under similar conditions, varying the amounts of monomers and the reaction time depending on the final copolymer composition. As an example, the 25
:
25 EtOx
:
SteOx copolymer (P1) was synthesized as follows. EtOx (0.097 mL, 0.96 mmol) and SteOx (0.2960 g, 0.96 mmol) were transferred into a microwave vial equipped with a magnetic stirring bar, which was then sealed and immersed in an oil bath at 130 °C. The reaction mixture was deoxygenated with a nitrogen flow for 30 minutes before adding 0.15 mL (0.039 mmol) of MeTos stock solution (49 mg mL−1 in dry chlorobenzene). The ratio of [monomers]
:
[I] was 50
:
1. Subsequently, the reaction mixture was reacted for 1h and 40 min in order to reach full conversion. 1H NMR (300 MHz, CDCl3), δ (ppm): 0.69–0.91 (br, 6H, CH2CH2CH3, COCH2CH3), 0.91–1.32 (br, 28H, CH2 alkyl chain), 1.32–1.63 (br, 2H, NCOCH2CH2), 2.02–2.40 (br, 4H, NCOCH2CH2, NCOCH2CH3), 3.14–3.58 (br, 8H, CH2 backbone).
Synthesis of methacrylic acid-(2-ethylhexyl) methacrylate random copolymer via RAFT polymerization (poly(xMA)).
Methacrylic acid (0.66 mL, 7.8 mmol, MAA) and 2-ethylhexyl methacrylate (7 mL, 31.2 mmol, EHMA) were transferred in a round bottom flask equipped with a magnetic stirring bar and dissolved in DMF in order to have a final monomer concentration of 5 mol L−1. A solution of 22.4 mg of V-601 initiator (0.098 mmol) in DMF as well as a solution of 86 mg of 2-cyano-2-propyl benzodithioate (0.39 mmol) in DMF were added to the flask. The ratio of [MAA]
:
[EHMA]
:
[CTA]
:
[I] was 20
:
80
:
1
:
0.25. Subsequently, the reaction mixture was deoxygenated with a nitrogen flow for 30 minutes before the flask was capped with a silicon septum and heated at 70 °C in an oil bath for 20 hours. The copolymers were obtained as a pink powder by precipitation into methanol. The conversion was determined by 1H NMR.
1H NMR (400 MHz, CDCl3), δ (ppm): 0.72–1.15 (br, 12H, COOHCCH3, COOCH2CCH3, CHCH2CH3, CH2CH2CH3), 1.16–1.47 (br, 8H, CHCH2CH3, CHCH2CH2, CH2CH2CH2, CH2CH2CH3), 1.47–1.64 (br, 1H, CH2CHCH2), 1.68–2.26 (br, 4H, CH2CCOOH, CH2CCOOCH2), 3.53–4.15 (br, 2H, OCH2CH).
Synthesis of graft copolymers via grafting-onto method.
Graft-copolymers were synthetized following the same synthetic procedure. The synthesis of one of the graft-copolymer (GP2) is given as an example. The CROP of 2-oxazoline monomers was performed in solution. EtOx (0.07 mL, 0.69 mmol) and SteOx (0.2091 g, 0.68 mmol) were transferred in a microwave vial equipped with a magnetic stirring bar, which was sealed and immersed in an oil bath at 100 °C. The mixture was deoxygenated with a nitrogen flow for 30 minutes before adding dry DCM in order to have a final monomer concentration of 4 mol L−1. Subsequently, 0.2 mL (0.052 mmol) of MeTos stock solution (49 mg mL−1 in dry chlorobenzene) was added and the reaction mixture was kept at 100 °C for 1 hour in order to reach 85–90% conversion. Afterwards, a solution of 0.0607 g (0.0037 mmol) of poly(xMA) and 0.002 mL (0.011 mmol) of TEA in dry DCM in order to have a final total concentration of 2 mol L−1 was added via a syringe into the capped microwave vial containing the living oligomeric oxazolinium species. This mixture was heated at 100 °C for 1 h. After evaporation of the solvent, the resulting graft-copolymers were analyzed via GPC without further purification.
Characterization
Nuclear magnetic resonance (1H NMR, HSQC, DOSY).
All spectra were recorded on a Bruker Avance III HD 300 MHz, III HD 400 MHz, and 500 MHz. CDCl3 was used as solvent and the signal of the residual CHCl3 served as reference for the chemical shift, δ. The data analysis was performed using TopSpin 3.2 software.
Gel permeation chromatography (GPC).
The measurements were performed using different eluents depending on the solubility of the samples and on the type of analysis. (i) THF with 2% TEA. The Agilent Technologies 1260 Infinity instrument was equipped with a refractive index (RI) and 308 nm UV detectors, a PLgel 5 μm guard column, and a PLgel 5 μm mixed D column (300 × 7.5 mm). Samples were run at 1 mL min−1 at 40 °C. Poly(methyl methacrylate) standards (Agilent PMMA calibration kits, M-M-10 and M-L-10) were used for the calibration. Before injection (100 μL), the samples were filtered through a PTFE membrane with 0.2 μL pore size. (ii) CHCl3 with 2% TEA. The Agilent Infinity II MDS instrument was equipped with differential refractive index (DRI), viscometry (VS), dual angle light scatter (LS) and multiple wavelength UV detectors, 2× PLgel Mixed C columns (300 × 7.5 mm) and a PLgel 5 μm guard column. Samples were run at 1 mL min−1 at 30 °C. Poly(methyl methacrylate), and polystyrene standards (Agilent EasiVials) were used for the calibration. Ethanol was added as a flow rate marker. The samples were filtered through a GVHP membrane with 0.22 μm pore size before injection (100 μL). (iii) THF with 0.01% BHT. The viscometric GPC measurements were carried out at known polymer concentration on an Agilent 1260 Infinity II-MDS instrument equipped with a refractive index detector and viscometer, and two PLgel Mixed-C columns. Samples were run at 1 mL min−1 at 40 °C. PMMA standards (Agilent EasiVials) were used to calibrate the instrument. All samples were dissolved in eluent and left stirring overnight before being filtered over 0.2 μm PTFE syringe filters and analysed. The data were determined by conventional calibration using Agilent GPC/SEC software.
Turbidity measurements.
Turbidity analyses for the determination of the transition temperature of each sample were performed using an Agilent Technologies Cary 100 UV-Vis spectrophotometer equipped with an Agilent Technologies Cary temperature controller and an Agilent Technologies 6 × 6 multicell block Peltier. The measurements were performed using Suprasil® quartz cuvettes (Hellman, 100-QS, light path = 10.00 mm) filled with 5 mg mL−1 solutions of each polymer in YuBase4. For each sample, two heating/cooling cycles between 15 and 85 °C were performed with a temperature gradient of 1 °C min−1 at λ = 600 nm. All data were recorded using the Cary WinUV software and elaborated using OriginPro 2019.
Thermogravimetric analysis (TGA).
The analyses were performed on a Mettler-Toledo TGA equipped with an autosampler under an air flow of 50 mL min−1 from 25 to 550 °C with a heating rate of 1 °C min−1. The samples (5–20 mg) were prepared using aluminium pans.
Differential scanning calorimetry (DSC).
Thermal transitions were determined on a Mettler-Toledo DSC1 equipped with an autosampler under nitrogen atmosphere with a flow of 50 mL min−1 from −80 to 150 °C. A heating/cooling rate of 60 °C min−1 was used for the first cycle (not considered for the subsequent calculations), and of 1 °C min−1 for the further two heating–cooling cycles. The samples (5–20 mg) were prepared using aluminium pans.
Results and discussion
Synthesis and characterization of 2-oxazoline random copolymers (polyOx) via CROP
Before the synthesis of the graft-copolymers, the solution behaviour and thermal properties of copolymers of 2-ethyl-2-oxazoline (EtOx) and 2-stearyl-2-oxazoline (SteOx) have been investigated. It has been hypothesised that the SteOx monomer would give thermoresponsiveness to the final copolymers as a result of the crystallization of the long alkyl chains, while EtOx would break the crystallinity leading to a tunable phase transition temperature in oil. For instance, a temperature-induced phase transition driven by the crystallization of long alkyl chains is a well know phenomenon, which has already been reported for other polymers, such as poly(octadecyl vinyl ether).59,60
The CROP of EtOx and SteOx homopolymers was carried out at 130 °C in bulk using a stock solution of methyl tosylate (MeTos) in dry chlorobenzene (C = 49 mg mL−1) as initiator (Scheme 2). Although this polymerization could be easily performed in solution, in order to not limit industrial applications, the CROP has been carried on in bulk, resulting in shorter reaction times and in the absence of further purification steps. The [monomer] to [MeTos] ratio was 50
:
1 for both homopolymers. For the synthesis of copolymers, distilled EtOx was added to a sealed reactor containing the melted SteOx at 130 °C, followed by addition of the initiator stock solution. The ratio of the two monomers, chosen in order to have oil soluble final copolymers, was varied as shown in Table 1, and the overall [monomers] to [MeTos] ratio was kept at 50
:
1. A quantitative monomer conversion determined from the 1H NMR spectra was obtained for all homo and copolymers (Fig. 1a). The CROP resulted in well-defined homo and copolymers characterized by low dispersity values (Fig. 1b). As already reported for other oxazoline monomers,61 the length of the linear alkyl substituent has a minimal influence on the propagation rate constant (kp). Kinetics studies of the copolymerization in solution of EtOx and SteOx confirmed the synthesis of random copolymers, with apparent reactivity ratios close to 1 (Fig. S1 and Table S1†). As can be observed from the data reported in Table 1, the experimental
n values are lower than the theoretical ones for all the synthesized homo and copolymers regardless of the used eluent. This difference is expected since the PMMA standards used for the GPC calibration have a different hydrodynamic volume compared to the poly(2-oxazoline)s one. Nevertheless, the conversion results obtained from the 1H NMR spectra as well as the low dispersity values account for good polymerization control of the CROP of the 2-oxazoline monomers with the conditions reported above.
 |
| Scheme 2 Reaction scheme of the cationic ring opening copolymerization of EtOx and SteOx initiated with MeTos. | |
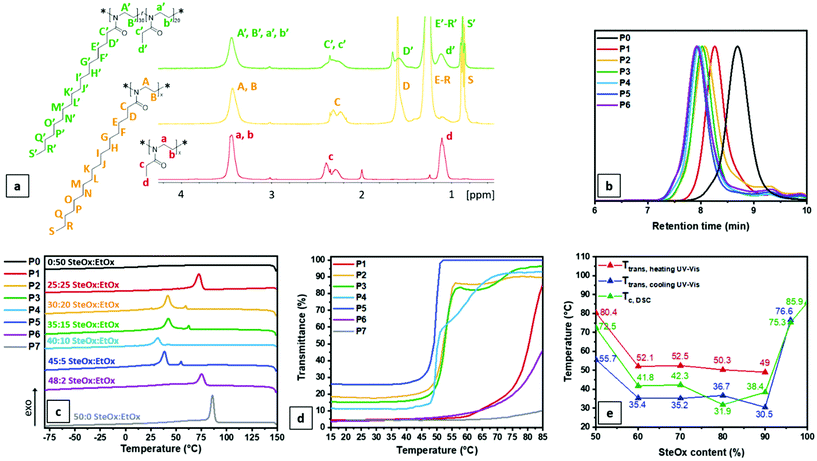 |
| Fig. 1 Characterization of 2-oxazoline homopolymers and copolymers with different SteOx : EtOx content obtained via CROP. [SteOx : EtOx] = 0 : 50 (P0, black), 25 : 25 (P1, red), 30 : 20 (P2, yellow), 35 : 15 (P3, green), 40 : 10 (P4, light blue), 45 : 5 (P5, navy blue), 48 : 2 (P6, purple), 50 : 0 (P7, grey). (a) 1H NMR spectra (400 MHz, CDCl3) and chemical structures of EtOx (red) and SteOx (yellow) homopolymers, and of the SteOx : EtOx 30 : 20 copolymer (P2, green), (b) GPC traces of P0–P6 using THF as eluent, (c) DSC curves of the third cooling cycle (exo up, normalised to the same heat flow per grams), (d) turbidity curves of the second heating cycle of polymer solutions in Yubase-4 (C = 5 mg mL−1) measured at λ = 600 nm, (e) comparison between transition temperatures of heating (Ttrans,heating UV-Vis, red) and cooling cycles (Ttrans,cooling UV-Vis, navy blue) obtained from turbidity analyses and crystallization temperatures (Tc, DSC, green) obtained from DSC cooling cycle. | |
Table 1 Copolymerization of 2-stearyl-2-oxazoline (SteOx) and 2-ethyl-2-oxazoline (EtOx) initiated with methyl tosylate (I)
Sample |
SteOx : EtOx : I |
Reaction time [min] |
1H NMR Conv. [%] |
n,th [g mol−1] |
n,GPC [g mol−1] |
Đ
|
Reaction conditions (SteOx : EtOx : I ratio, reaction time), theoretical and experimental number average molecular weight distributions ( n,th and n,GPC), and dispersity index (Đ) for each of the obtained homo and copolymers. For each homo and copolymer, a conversion of >99% was obtained and it was determined from 1H NMR spectra. For all copolymers and EtOx homopolymer n,GPCand Đ were obtained via GPC using THF as eluent against PMMA standards. In the case of SteOx homopolymer, due to its insolubility in THF, *CHCl3 against PMMA standards was used as eluent. |
P0
|
0 : 50 : 1 |
60 |
>99 |
5000 |
3300 |
1.22 |
P1
|
25 : 25 : 1 |
100 |
>99 |
10 000 |
5500 |
1.21 |
P2
|
30 : 20 : 1 |
120 |
>99 |
11 000 |
7500 |
1.26 |
P3
|
35 : 15 : 1 |
60 |
>99 |
12 000 |
9500 |
1.20 |
P4
|
40 : 10 : 1 |
80 |
>99 |
13 000 |
10 000 |
1.25 |
P5
|
45 : 5 : 1 |
90 |
>99 |
14 000 |
10 800 |
1.22 |
P6
|
48 : 2 : 1 |
95 |
>99 |
15 000 |
11 000 |
1.27 |
P7
|
50 : 0 : 1 |
90 |
>99 |
15 500 |
10 900* |
1.21* |
The thermal properties of the homo and copolymers were evaluated via thermogravimetric analysis (TGA) and differential scanning calorimetry (DSC) measurements. All polymers showed a thermal stability up to 300 °C (Fig. S2†). For the DSC measurements, a first fast heating/cooling cycle from −80 to 150 °C at 60 °C min−1 was used to remove the thermal history of the samples, then two further cycles at 1 °C min−1 were performed. Fig. 1c shows the traces of the third cooling cycle of each homo and copolymer. As can be observed, all random copolymers and the SteOx homopolymer present a crystallization peak, while the EtOx homopolymer (P0) does not show any thermal transitions in the analysed range of temperature. P1 and P6 show a higher crystallization temperature (Tc) closer to the one of the SteOx homopolymer (P7) compared to the other copolymers. This can be explained with an increase in the overall order of the two systems. On one hand, P6 contains the lowest amount of EtOx, resulting in a copolymer mainly composed of SteOx. Consequently, the number of EtOx units are not enough to break the crystallinity of the long alkyl side chains of the second monomer, which can easily align into ordered crystalline structures leading to a Tc closer to the one of the SteOx homopolymer. On the other hand, P1 contains the highest amount of EtOx; however, the two monomers are polymerized in equal amounts. Since EtOx and SteOx monomers have a similar reactivity, they should be evenly alternated in the final copolymer, resulting in an overall more ordered system compared to other copolymers. As a consequence, polymer chains can easily pack and form crystalline structures, which results in a high Tc. P2 and P3 show a similar crystallization temperature lower than the one of the SteOx homopolymer. The uneven distribution of two monomers along the polymer makes the packing between polymer chains more difficult, resulting in less crystalline areas. This aspect is even more accentuated in the case of P4, which shows the lowest Tc. Interestingly, the DSC curves of the samples P2–P5 present two exothermic phenomena, suggesting the presence of two microstructures, possibly a SteOx–EtOx-mixed and a SteOx-rich phase. Further analyses will be performed to elucidate the microstructure of the copolymers. The solubility behaviour in oil of the SteOx homopolymer and of the copolymers was evaluated via turbidity measurements. 5 mg mL−1 solutions in Yubase-4 were prepared and then subjected to two heating/cooling cycles from 15 to 85 °C at a wavelength of 600 nm. Fig. 1d shows the curves of the second heating cycle for each sample. All copolymers exhibit a UCST-type behaviour. At high temperatures, the polymers are soluble in the examined oil resulting in transparent solutions with a transmittance close to 100%. However, when the temperature is decreased, the transmittance drops for all polymers and the polymer solutions become heterogeneous due to the formation of large aggregates caused by the crystallization of the long alkyl chains of the SteOx units. However, it is important to notice that for all samples the transmittance at the lowest temperatures does not go down to 0%, meaning that the polymers are still slightly soluble in oil even at 15 °C. Furthermore, the copolymers with a less ordered structure (P2–P5) exhibit higher transmittance values at low temperatures compared to the more ordered counterparts. During the cooling cycle, the uneven distribution of the two monomers along the polymer chains hinders the formation of crystalline domains, resulting in less packed structures and, thus, in more oil soluble polymers even at low temperatures. As in the case of DSC analyses, the samples having an overall more ordered structure (i.e.P1, P6, P7) exhibit high transition temperatures (Ttrans) since a higher temperature is required to break the crystallinity of the polymer chains compared to the other less ordered polymers. By decreasing the order in the composition of the polymer chains, and thus, the amount of crystalline areas in the copolymers, the Ttrans decreases from around 52 °C in the case of P2 and P3, to 50 °C for P4, to 49 °C for P5 (Fig. 1d). The turbidity curves of the samples P2–P4 show two transition temperatures, which are probably caused by their low ordered nature: during the first transition the main crystalline areas of the polymer chains are solubilized resulting in a sharp phase transition. However, with an additional increase in temperature the polymer chains further expand, making also possible the solubilisation of small crystalline structures and, thus, resulting in a second, less sharp phase transition. Fig. 1e shows the comparison between the transition temperatures of the heating (Ttrans,heating UV-Vis) and cooling (Ttrans,cooling UV-Vis) cycles obtained via turbidity measurements and calculated at 50% transmittance, as well as the crystallization temperature (Tc, DSC) measured via DSC analyses as a function of the SteOx content (%) in the copolymer. As can be observed, the data are in good agreement, meaning that it is possible to correlate the degree of crystallinity in the copolymers with the temperature at which the phase transition in Yubase-4 occur.
Synthesis and characterization of graft copolymers obtained via the grafting-onto method
The first step involved in the grafting-onto method is the synthesis of the poly((methacrylic acid)-r-(2-ethylhexyl) methacrylate) backbone (poly(xMA)) via RAFT polymerization. The two monomers were chosen because 2-ethylhexyl methacrylate (EHMA) has been widely used for the synthesis of hydrophobic polymers, while the methacrylic acid (MAA) units represent the grafting sites on the final backbone. The polymerization of MAA and EHMA was carried out in a 5 M solution in DMF at 70 °C using 2-cyano-2-propyl benzodithioate (CPBD) as the chain transfer agent, and V-601 as the initiator. The effect on the hydrophobicity of the final copolymer was evaluated by varying the ratio between the two monomers. In order to have a final polymer soluble in the desired solvent for the grafting-onto reaction, the optimal [MAA] to [EHMA] ratio was found to be 20 to 80. The overall [monomer] to [CPDB] to [V-601] ratio was 100
:
1
:
0.25. The monomer conversion was determined by proton and HSQC NMR, which showed a quantitative conversion for both monomers (Fig. 2 and Fig. S4†), while the experimental
n (17
500 g mol−1) and the dispersity (1.18) values were determined from GPC measurements. The second step of the grafting-onto method is the connection between the polymer backbone and the 2-oxazoline side chains (Schemes 1 and 3). The CROP of SteOx and EtOx monomers was conducted at 100 °C in dry DCM (C = 4 M) using a stock solution of MeTos in dry chlorobenzene. When the desired conversion was reached, a solution containing a 1.4 times excess of poly(xMA) and triethylamine (TEA) in dry DCM was directly added in the vial containing the living oxazoline chains. TEA was added to deprotonate the carboxylic groups of the methacrylic acid units, used to directly end-cap the oxazoline polymer chains. An excess of poly(xMA) was used to avoid steric hindrance between the side chains in order to reach a high brush yield and to evaluate the effect of free carboxylic groups on the solution behaviour in oil of the final graft-copolymers. The final reaction mixture (C = 2 M) was heated at 100 °C for 1 hour. A library of graft-copolymers was synthetized in order to evaluate the effect of different parameters on the efficiency of the grafting-onto reaction (Table 2). Unfortunately, due to the solubility in same solvents of both the graft-copolymers and the unreacted oxazoline side chains, the purification of the final graft-copolymers was not possible. All synthetized polyOx are characterized by low polydispersity indexes as expected from a controlled living polymerization. Also the graft-copolymers show narrow molecular weight distributions, meaning that the grafting-onto step is a fast reaction. When the poly(xMA) is added to the reaction mixture containing the oxazoline copolymer, the deprotonated carboxylic groups immediately react with the living oxazoline chain ends resulting in well-defined graft-copolymers. Furthermore, DOSY analyses performed on the backbone copolymer and on one of the graft-copolymers show the success of the grafting-onto reaction, since no backbone signals are visible in the spectrum of the graft-copolymer (Fig. 2). Moreover, Mark–Houwink plots obtained from viscometric GPC analyses performed on one of the graft-copolymers, the polyOx side chains and the methacrylates backbone showed a decrease in the viscosity for the graft-copolymer, suggesting branching and, thus, that the polyOx side chains are actually grafted onto the backbone (Fig. S5†). Since it was not possible to clearly distinguish from the proton, HSQC and DOSY NMR spectra of the graft-copolymers the difference between the peaks of the grafted and unreacted polyOx side chains (Fig. 2 and Fig. S6†), the brush yield of all graft-copolymers was determined by integration of both the peaks corresponding to the unreacted polyOx and the graft-copolymer in the GPC trace (Fig. 3) (Please see ESI† for the calibration method.). As expected, similar results were obtained for the brush yield of all graft-copolymers using the two methods.
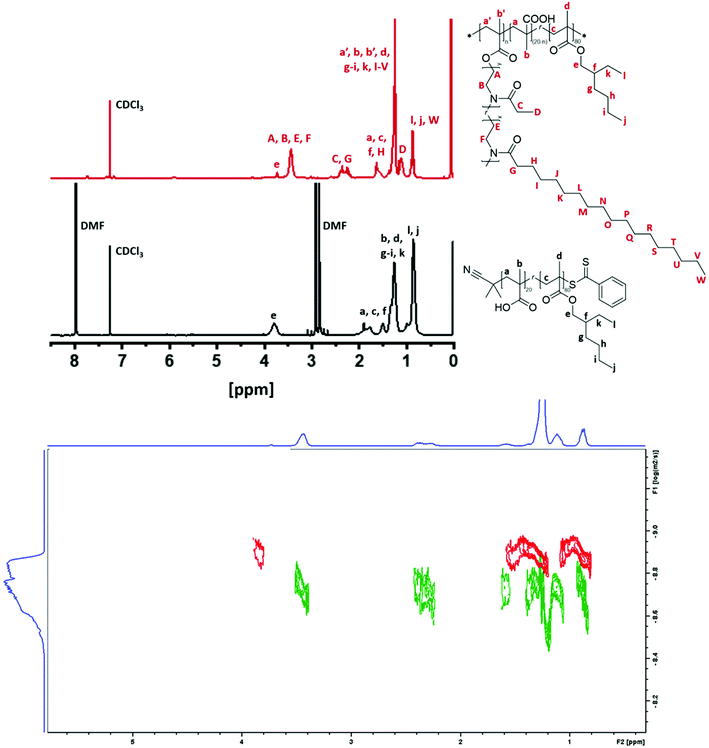 |
| Fig. 2
1H NMR spectra (400 MHz, CDCl3) and structures of the poly(methacrylic acid)-r-(2-ethylhexyl)methacrylate copolymer (poly(xMA)) (black), and a graft-copolymer (red), and DOSY spectrum (500 MHz, CDCl3) of the graft copolymer (green) with the overlap of the DOSY spectrum of the poly(xMA) backbone (red). | |
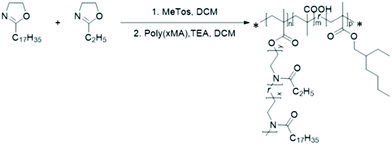 |
| Scheme 3 Reaction scheme of the synthetic procedure used to obtain the graft copolymers. (1) CROP of SteOx and EtOx monomers initiated with methyl tosylate (MeTos) in DCM, and (2) grafting-onto reaction with methacrylic acid-(2-ethyl hexyl) methacrylate copolymer in presence of trimethylamine (TEA) in DCM. | |
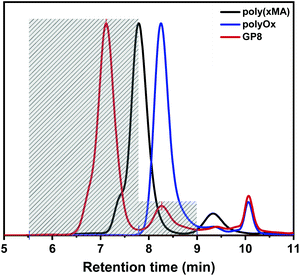 |
| Fig. 3 GPC traces using THF as eluent against PMMA standards of poly(xMA) (black), polyOx before the grafting-onto reaction (blue), and of the graft-copolymer (red), and integrated areas for the calculation of the brush yield. | |
Table 2 Graft copolymers with a poly(xMA) backbone and polyOx side chains obtained via the grafting-onto method
Sample |
SteOx : EtOx |
n,polyOx,th [g mol−1] |
n,polyOx,GPC [g mol−1] |
Đ
polyOx
|
Brush yielda [%] |
Brush yieldb [%] |
n,graft,th [g mol−1] |
n,graft,GPC [g mol−1] |
Đ
graft
|
Calculated by integration of the GPC traces of the graft copolymers.
Calculated by the calibration method.
PolyOx conversion = 50%, CROP time = 35 min.
PolyOx conversion = 85%, CROP time = 60 min.
PolyOx conversion = 99%, CROP time = 120 min.
PolyOx conversion = 99% (the polymerization went to full conversion, but it was kept reacting for a longer time), CROP time = 180 min.
Grafting reaction temperature = 70 °C.
Grafting T = 80 °C.
Grafting T = 120 °C.
PolyOx DP = 50.
PolyOx DP = 100.
[Ox+] : [COOH] = 7 : 20.
|
GP1
|
12.5 : 12.5 |
2600 |
4500 |
1.19 |
58 |
67 |
54 000 |
41 000 |
1.14 |
GP2
|
12.5 : 12.5 |
4300 |
7000 |
1.15 |
79 |
80 |
78 000 |
60 000 |
1.18 |
GP3
|
12.5 : 12.5 |
5110 |
5500 |
1.16 |
65 |
71 |
89 000 |
55 000 |
1.17 |
GP4
|
12.5 : 12.5 |
5110 |
6700 |
1.14 |
70 |
73 |
89 000 |
51 000 |
1.19 |
GP5
|
12.5 : 12.5 |
4700 |
4600 |
1.18 |
75 |
78 |
84 000 |
57 000 |
1.20 |
GP6
|
12.5 : 12.5 |
4600 |
4700 |
1.18 |
87 |
86 |
82 000 |
60 000 |
1.21 |
GP7
|
12.5 : 12.5 |
4100 |
5300 |
1.18 |
87 |
87 |
75 000 |
64 000 |
1.21 |
GP8
|
17.5 : 7.5 |
5100 |
5800 |
1.16 |
84 |
84 |
89 000 |
65 000 |
1.21 |
GP9
|
22.5 : 2.5 |
6500 |
6000 |
1.17 |
56 |
61 |
109 000 |
74 500 |
1.21 |
GP10
|
25 : 25 |
9200 |
9300 |
1.21 |
74 |
76 |
147 000 |
106 000 |
1.33 |
GP11
|
50 : 50 |
20 400 |
10 400 |
1.22 |
63 |
63 |
303 000 |
130 000 |
1.33 |
GP12
|
12.5 : 12.5 |
5100 |
6100 |
1.17 |
78 |
80 |
54 000 |
39 300 |
1.16 |
In order to optimize the reaction conditions, the conversion of the oxazoline side chains and the temperature of the grafting-onto reaction were first evaluated. Fig. 4a shows the GPC traces of the graft-copolymers obtained by varying the conversion of the initial 2-oxazoline copolymers from 50% to full conversion, while keeping constant their composition (SteOx
:
EtOx = 12.5
:
12.5), their degree of polymerization (DP = 25), the reaction time of the grafting-onto step, and the ratio between the living oxazoline chain ends and the deprotonated carboxylic groups ([Ox+]
:
[COOH] = 14
:
20). In the case of GP4, the CROP of the oxazoline monomers was left reacting even after reaching full conversion in order to evaluate the effect of dead chains caused by possible side reactions on the final brush yield and to determine the limit of the grafting-onto reaction. As can be observed, all graft-copolymers have a narrow molecular weight distribution independently of the polyOx conversion. Moreover, the unreacted oxazoline copolymers in the reaction mixture is lower than 35% even when the oxazoline copolymer is over-reacted and, thus, more susceptible to undergo side reactions. This means that the grafting-onto reaction works with high brush yields even when the reaction conditions are not optimal. However, the graft-copolymers do not completely dissolve in the oil even at high temperatures, resulting in heterogeneous solutions. Nevertheless, as can be observed from the turbidity curves in Fig. 4b, they all show a UCST behaviour with transition temperatures between 50 and 40 °C. Since the graft-copolymer obtained using an 85% conversion polyOx resulted in the highest brush yield (∼80%), optimum 2–oxazoline conversion value at 85–90% was chosen for the synthesis of the graft-copolymers. In order to evaluate the effect of the temperature of the grafting-onto step on the grafting efficiency, the temperature of the second reaction was varied from 70 to 120 °C, while keeping constant the composition (SteOx
:
EtOx = 12.5
:
12.5) and the degree of polymerization (DP = 25) of the side chains, their conversion (85–90%), the grafting reaction time (1 h), and the ratio between the living oxazoline chain ends and the deprotonated carboxylic groups ([Ox+]
:
[COOH] = 14
:
20). Also, in this case the resulting graft-copolymers show narrow molecular weight distributions and high brush yields (Fig. 4c). However, at low (70, 80 °C) and high (120 °C) temperatures slightly more side reactions are observed. For this reason, a constant temperature of 100 °C was chosen for the synthesis of the graft-copolymers in order to carry on fast reactions in a still controlled manner. Moreover, even though the graft-copolymers show an extremely poor solubility in oil, a UCST-type behaviour can still be observed (Fig. 4d).
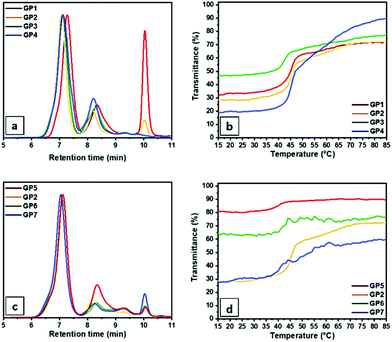 |
| Fig. 4 Effect of the conversion of the poly(2-alkyl-2-oxazoline) side chains and of the grafting-onto reaction temperature on the grafting efficiency: (a) GPC traces, and (b) turbidity measurements (5 mg mL−1 polymer solutions in Yubase 4 measured at λ = 600 nm) of the graft-copolymers obtained by varying the conversion of the initial oxazoline copolymers: 85% (GP1, red), 50% (GP2, yellow), 99% (GP3, green) conversion, and over-reacted (GP4, blue). (c) GPC traces, and (d) turbidity measurements (5 mg mL−1 polymer solutions in Yubase-4 measured at λ = 600 nm) of the graft-copolymers obtained by varying the temperature of the grafting-onto reaction: 100 (GP5, red), 120 (GP2, yellow), 80 (GP6, green), and 70 °C (GP7, blue). | |
Graft-copolymers with different side chain compositions have been synthetized in order to improve the overall solubility in oil. All graft-copolymers show narrow molecular weight distributions (Fig. 5a), with high brush yields for GP2 (SteOx
:
EtOx = 12.5
:
12.5, ∼80%), and GP8 (SteOx
:
EtOx = 17.5
:
7.5, ∼84%), while a decreased brush yield is observed for GP9 (SteOx
:
EtOx = 22.5
:
2.5, ∼60%). This is probably caused by the steric hindrance of the long alkyl groups of the SteOx units, which decreases the efficiency of the grafting-onto reaction. As expected, a higher content of SteOx and, thus, a higher order in the overall structure of the graft-copolymer, results in an increase in the crystallization temperature (i.e. 36 °C for GP9), while the other two samples show a similar Tc around 29 °C (Fig. 5b). This trend can also be seen in the turbidity measurements, in which GP9 exhibits the highest Ttrans at around 51 °C, while the other two graft-copolymers show a similar Ttrans at around 46 °C (Fig. 5c). In addition, an improvement in the oil solubility is observed for GP9 as can be deduced from the low transmittance values at low temperatures. Also in this case, the transition temperature in both heating and cooling cycles can be related to the crystallization temperature for all graft-copolymers, and the obtained data are in good agreement (Fig. 5d).
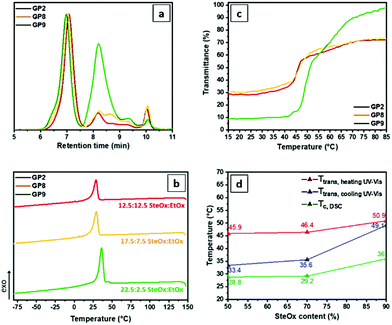 |
| Fig. 5 Effect of the side chains composition on the grafting efficiency: (a) GPC traces using THF as eluent against PMMA standards, (b) DSC curves of the third cooling cycle (exo up, normalised to the same heat flow per grams), (c) turbidity curves of the second heating cycle of polymer solutions in YuBase-4 (c = 5 mg mL−1) measured at λ = 600nm, (d) comparison between the transition temperatures of the heating (Ttrans,heating UV-Vis, red) and the cooling cycles (Ttrans,cooling UV-Vis, blue) obtained from turbidity measurements and the crystallization temperatures (Tc,DSC, green) obtained from the DSC cooling cycle of graft-copolymers with varying the SteOx : EtOx composition in the side chains: 12.5 : 12.5 (GP2, red), 17.5 : 7.5 (GP8, yellow), and 22.5 : 2.5 (GP9, green). | |
The effect of the degree of polymerization of the 2-oxazoline side chains on the grafting efficiency as well as on the overall oil solubility of the final graft-copolymers has been investigated by synthesizing graft-copolymers with oxazoline side chains characterized by different DP, but with the same SteOx
:
EtOx ratio. As can be observed from the GPC traces (Fig. 6a), even though the molecular weight distributions are still characterized by narrow dispersity values, the higher the DP of the oxazoline chains, the more pronounced is the peak shoulder at low retention times, and the lower is the overall brush yield (i.e. ∼80% for GP2, ∼75% for GP10, and ∼63% for GP11). In addition, GP10 and GP11 resulted to be completely insoluble in the oil even at high temperatures, making the determination of their thermoresponsive behaviour impossible. The DSC cooling curves (Fig. 6b) show a crystallization peak for GP2 and GP10, while no thermal transitions were observed for GP11 in the analysed temperature range. These results together with the obtained brush yields and the complete insolubility in oil might be explained with a poor grafting efficiency due to the stearic hindrance caused by the long oxazoline polymer chains, resulting in more free carboxylic groups in the graft-copolymers with DP 50 and DP 100 side chains compared to the one containing DP 25 polyOx side chains.
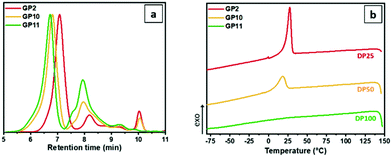 |
| Fig. 6 Effect of the degree of polymerization of the side chains on the grafting efficiency: (a) GPC traces using THF as eluent against PMMA standards, (b) DSC curves of the third cooling cycle (exo up, normalised to the same heat flow per grams) of graft-copolymers having 2-oxazoline side chains with different degrees of polymerization: DP 25 (GP2, red), DP 50 (GP10, yellow), and DP 100 (GP11, green). | |
The tunability of the grafting density on the final graft-copolymers was evaluated by further decrease the ratio between the polyOx living chain ends and the deprotonated carboxylic groups on the poly(xMA) backbone from 14
:
20 to 7
:
20. As can be observed from the GPC traces (Fig. 7a), both graft-copolymers have a narrow molecular weight distribution, which is lower for GP12, as expected. In addition, high brush yields were obtained for both polymers (∼80% for both GP2 and GP12). As predictable, GP12 was not soluble in oil due to the presence of a high amount of free carboxylic acid groups, and its thermoresponsive behaviour could not be evaluated. However, a small crystallization peak is observed in the DSC cooling curve (Fig. 7b). As expected, the Tc is lower compared to GP2 due to the presence of a lower number of side chains resulting in the formation of less crystalline domains. From the obtained results, it is possible to say that the grafting density can be tuned by simply varying the ratio between the living oxazoline chain ends and the carboxylic groups on the backbone, however, the ratio should be carefully chosen in order to have an oil soluble thermoresponsive graft-copolymer.
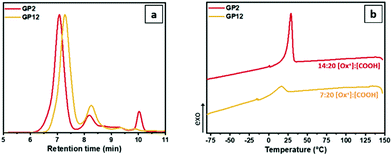 |
| Fig. 7 Effect of the grafting density on the grafting efficiency: (a) GPC traces using THF as eluent against PMMA standards, (b) DSC curves of the third cooling cycle (exo up, normalised to the same heat flow per grams) of graft-copolymers with different grafting density: [Ox+] : [COOH] = 14 : 20 (GP2, red), and 7 : 20 (GP12, yellow). | |
Conclusions
In conclusion, the aim of this research project was the synthesis of oil soluble graft-copolymers based on 2-oxazoline monomers showing thermoresponsiveness in pure non-aqueous systems and, in particular, a UCST-type phase transition in Yubase-4. Initially, linear homo and copolymers of 2-stearly-2-oxazoline and 2-ethyl-2-oxazoline were synthetized via CROP in bulk and their solution properties were investigated via turbidity measurements in a commercially available oil, and their thermal properties were evaluated by TGA and DSC analyses. In general, by increasing the overall order of the copolymer structure, an increase in the crystallization temperature as well as of the transition temperature in oil was observed due to a better alignment of the polymer chains, which can pack and form crystalline areas. In addition, it was possible to correlate the data obtained from thermal analyses and turbidity measurements, which were indeed in good agreement. Subsequently, graft-copolymers were obtained via the grafting-onto method: the multifunctional polymer forming the backbone consisted of a copolymer of methacrylic acid and 2-ethylhexyl methacrylate synthetized via RAFT polymerization, while the side chains were obtained via CROP in solution of SteOx and EtOx monomers. The reaction conditions of the grafting-onto step (i.e. reaction temperature, polyOx conversion) as well as parameters to increase the oil solubility of the final graft-copolymers (i.e. polyOx composition and DP, and grafting density) were evaluated. The UCST-type phase transition of the graft-copolymers was also analysed using turbidity and thermal measurements. A polyOx conversion between 85 and 90% and a temperature of 100 °C for the grafting-onto reaction appeared to be the optimal conditions to synthetized graft-copolymers in a fast and still controlled way. As expected, by increasing the amount of SteOx in the side chains composition, an increase in the oil solubility of the graft-copolymer was observed. However, the final brush yield was low, most likely due to the steric hindrance caused by the long alkyl groups on the SteOx units, which decreased the efficiency of the grafting reaction. The DP of the oxazoline side chains on the grafting efficiency as well as on the overall oil solubility was also evaluated. A decrease in the brush yield was observed with the increase in the polyOx DP. In addition, samples with DP 50 and DP 100 side chains resulted to be completely insoluble in oil probably due to a low grafting efficiency caused by the stearic hindrance between the long oxazoline polymer chains, which led to a higher amount of free carboxylic groups on the backbone and, thus, to a poor oil solubility. Finally, the tunability of the grafting density was explored by decreasing the ratio between the living oxazoline chain ends and the carboxylic groups on the backbone from 14
:
20 to 7
:
20. While it was observed that the grafting density could be simply varied by changing the [Ox+]
:
[COOH] ratio resulting in high-yielded, well-defined graft-copolymers, the presence of many free carboxylic groups resulted in the complete insolubility in oil of the synthetized graft-copolymer.
Conflicts of interest
There are no conflicts to declare.
References
- T. Kagiya, S. Narisawa, T. Maeda and K. Fukui, Ring-Opening Polymerization of 2-Substituted 2-Oxazolines, J. Polym. Sci., Part B: Polym. Lett., 1966, 4(7), 441–445 Search PubMed.
- W. Seeliger, E. Aufderhaar, W. Diepers, R. Feinauer, R. Nehring, W. Thier and H. Hellmann, Recent Syntheses and Reactions of Cyclic Imidic Esters, Angew. Chem., Int. Ed. Engl., 1966, 5(10), 875–888 Search PubMed.
- D. A. Tomalia and D. P. Sheetz, Homopolymerization of 2-alkyl- and 2-aryl-2-oxazolines, J. Polym. Sci., Part A-1: Polym. Chem., 1966, 4(9), 2253–2265 Search PubMed.
- T. G. Bassiri, A. Levy and M. Litt, Polymerization of Cyclic Imino Ethers. I. Oxazolines, J. Polym. Sci., Part B: Polym. Lett., 1967, 5(9), 871–879 Search PubMed.
- N. Adams and U. S. Schubert, Poly(2-oxazolines) in Biological and Biomedical Application Contexts, Adv. Drug Delivery Rev., 2007, 59(15), 1504–1520 Search PubMed.
- R. Hoogenboom, Poly(2-oxazoline)s: A Polymer Class with Numerous Potential Applications, Angew. Chem., Int. Ed., 2009, 48(43), 7978–7994 Search PubMed.
- O. Sedlacek, B. D. Monnery, S. K. Filippov, R. Hoogenboom and M. Hruby, Poly(2-Oxazoline)s – Are They More Advantageous for Biomedical Applications Than Other Polymers?, Macromol. Rapid Commun., 2012, 33(19), 1648–1662 Search PubMed.
- P. Wilson, P. C. Ke, T. P. Davis and K. Kempe, Poly(2-oxazoline)-Based Micro- and Nanoparticles: A Review, Eur. Polym. J., 2017, 88, 486–515 Search PubMed.
- B. Verbraeken, B. D. Monnery, K. Lava and R. Hoogenboom, The Chemistry of Poly(2-oxazoline)s, Eur. Polym. J., 2017, 88, 451–469 Search PubMed.
- S. Kobayashi, T. Igarashi, Y. Moriuchi and T. Saegusa, Block Copolymers from Cyclic Imino Ethers: A New Class of Nonionic Polymer Surfactant, Macromolecules, 1986, 19(3), 535–541 Search PubMed.
- T. Saegusa, Y. Chujo, K. Aoi and M. Miyamoto, One-Shot Block Copolymerization, Makromol. Chem., Macromol. Symp., 1990, 31(1), 1–10 Search PubMed.
- R. Hoogenboom, H. M. Thijs, M. W. Fijten and U. S. Schubert, Synthesis, characterization, and cross-linking of a library of statistical copolymers based on 2-“soy alkyl”-2-oxazoline and 2-ethyl-2-oxazoline, J. Polym. Sci., Part A: Polym. Chem., 2007, 45(23), 5371–5379 Search PubMed.
- R. Hoogenboom, Poly(2-Oxazoline)s: Alive and Kicking, Macromol. Chem. Phys., 2007, 208(1), 18–25 Search PubMed.
- E. Rossegger, V. Schenk and F. Wiesbrock, Design Strategies for Functionalized Poly(2-oxazoline)s and Derived Materials, Polymers, 2013, 5(3), 956–1011 Search PubMed.
- H. Schlaad, C. Diehl, A. Gress, M. Meyer, A. L. Demirel, Y. Nur and A. Bertin, Poly(2-Oxazoline)s as Smart Bioinspired Polymers, Macromol. Rapid Commun., 2010, 31(6), 511–525 Search PubMed.
- D. Pizzi, J. Humphries, J. P. Morrow, N. L. Fletcher, C. A. Bell, K. J. Thurecht and K. Kempe, Poly(2-Oxazoline) Macromonomers as Building Blocks for Functional and Biocompatible Polymer Architectures, Eur. Polym. J., 2019, 121, 109258 Search PubMed.
- S. I. Shoda, E. Masuda, M. Furukawa and S. Kobayashi, Synthesis and Surfactant Property of Copolymers Having a Poly(2-Oxazoline) Graft Chain, J. Polym. Sci., Part A: Polym. Chem., 1992, 30, 1489–1494 Search PubMed.
- J. Bühler, S. Muth, K. Fischer and M. Schmidt, Collapse of Cylindrical Brushes with 2-Isopropyloxazoline Side Chains Close to the Phase Boundary, Macromol. Rapid Commun., 2013, 34(7), 588–594 Search PubMed.
- C. Weber, C. R. Becer, W. Guenther, R. Hoogenboom and U. S. Schubert, Dual Responsive Methacrylic Acid and Oligo(2-Ethyl-2-Oxazoline) Containing Graft Copolymers, Macromolecules, 2010, 43(1), 160–167 Search PubMed.
- M. M. Bloksma, C. Weber, I. Y. Perevyazko, A. Kuse, A. Baumgärtel, A. Vollrath, R. Hoogenboom and U. S. Schubert, Poly(2-Cyclopropyl-2-Oxazoline): From Rate Acceleration by Cyclopropyl to Thermoresponsive Properties, Macromolecules, 2011, 44(11), 4057–4064 Search PubMed.
- C. Weber, M. Wagner, D. Baykal, S. Hoeppener, R. M. Paulus, G. Festag, E. Altuntas, F. H. Schacher and U. S. Schubert, Easy Access to Amphiphilic Heterografted Poly(2-Oxazoline) Comb Copolymers, Macromolecules, 2013, 46(13), 5107–5116 Search PubMed.
- J. Rueda, S. Zschoche, H. Komber, D. Schmaljohann and B. Voit, Synthesis and Characterization of Thermoresponsive Graft Copolymers of NIPAAm and 2-Alkyl-2-Oxazolines by the “Grafting from” Method, Macromolecules, 2005, 38(17), 7330–7336 Search PubMed.
- N. Zhang, S. Huber, A. Schulz, R. Luxenhofer and R. Jordan, Cylindrical Molecular Brushes of Poly(2-Oxazoline)s from 2-Isopropenyl-2-Oxazoline, Macromolecules, 2009, 42(6), 2215–2221 Search PubMed.
- N. Zhang, S. Salzinger, B. S. Soller and B. Rieger, Rare Earth Metal-Mediated Group-Transfer Polymerization: From Defined Polymer Microstructures to High-Precision Nano-Scaled Objects, J. Am. Chem. Soc., 2013, 135(24), 8810–8813 Search PubMed.
- G. D. Poe and C. L. McCormick, Synthesis, Complex Formation, and Dilute-Solution Associative Behavior of Linear Poly(Methacrylic Acid)-graft-Poly(2-Ethyl-2-Oxazoline), J. Polym. Sci., Part A: Polym. Chem., 2004, 42(10), 2520–2533 Search PubMed.
- B. Guillerm, V. Darcos, V. Lapinte, S. Monge, J. Coudaneb and J. J. Robin, Synthesis and Evaluation of Triazole-Linked Poly(ε-Caprolactone)-graft-Poly(2-Methyl-2-Oxazoline) Copolymers as Potential Drug Carriers, Chem. Commun., 2012, 48(23), 2879–2881 Search PubMed.
- X. Zheng, C. Zhang, L. Bai, S. Liu, L. Tan and Y. Wang, Antifouling Property of Monothiol-Terminated Bottle-Brush Poly (Methylacrylic Acid)-Graft-Poly (2-Methyl-2-Oxazoline) Copolymer on Gold Surfaces, J. Mater. Chem. B, 2015, 3(9), 1921–1930 Search PubMed.
- D. Rayeroux, C. Travelet, V. Lapinte, R. Borsali, J. J. Robin and C. Bouilhac, Tunable Amphiphilic Graft Copolymers Bearing Fatty Chains and Polyoxazoline: Synthesis and Self-Assembly Behavior in Solution, Polym. Chem., 2017, 8(29), 4246–4263 Search PubMed.
- R. B. Grubbs and R. H. Grubbs, 50th Anniversary Perspective: Living Polymerization-Emphasizing the Molecule in Macromolecules, Macromolecules, 2017, 50(18), 6979–6997 Search PubMed.
-
A. Rudin and P. Choi, Free-Radical Polymerization, in The Elements of Polymer Science & Engineering, Academic Press, 3rd edn, 2013, pp. 341–389 Search PubMed.
- D. Greszta, D. Mardare and K. Matyjaszewski, “Living” Radical Polymerization. 1. Possibilities and Limitations, Macromolecules, 1994, 27(3), 638–644 Search PubMed.
- J. Chiefari, Y. K. Chong, F. Ercole, J. Krstina, J. Jeffery, T. P. T. Le, R. T. A. Mayadunne, G. F. Meijs, C. L. Moad, G. Moad, E. Rizzardo and S. H. Thang, Living Free-Radical Polymerization by Reversible Addition-Fragmentation Chain Transfer: The RAFT Process, Macromolecules, 1998, 31(16), 5559–5562 Search PubMed.
- S. Perrier, 50th Anniversary Perspective: RAFT Polymerization—A User Guide, Macromolecules, 2017, 50(19), 7433–7447 Search PubMed.
- C. Weber, C. R. Becer, R. Hoogenboom and U. S. Schubert, Lower Critical Solution Temperature Behavior of Comb and Graft Shaped Poly[Oligo(2-Ethyl-2-Oxazoline)Methacrylate]s, Macromolecules, 2009, 42(8), 2965–2971 Search PubMed.
- A. Krieg, C. Weber, R. Hoogenboom, C. R. Becer and U. S. Schubert, Block Copolymers of Poly(2-Oxazoline)s and Poly(Meth)Acrylates: A Crossover between Cationic Ring-Opening Polymerization (CROP) and Reversible Addition–Fragmentation Chain Transfer (RAFT), ACS Macro Lett., 2012, 1(6), 776–779 Search PubMed.
- M. Kang, S. Y. Lee, H. H. Shin, Y. C. Yu and J. H. Youk, Microwave-Assisted Rapid One-Step Synthesis of Poly(2-Oxazoline)-Based Block Copolymers Using a Dual Initiator for CROP and RAFT Polymerization, Polymer, 2016, 87, 108–113 Search PubMed.
- I. Yildirim, T. Bus, M. Sahn, T. Yildirim, D. Kalden, S. Hoeppener, A. Traeger, M. Westerhausen, C. Weber and U. S. Schubert, Fluorescent Amphiphilic Heterografted Comb Polymers Comprising Biocompatible PLA and PEtOx Side Chains, Polym. Chem., 2016, 7(39), 6064–6074 Search PubMed.
- C. Giacomelli, V. Schmidt, K. Aissou and R. Borsali, Block Copolymer Systems: From Single Chain to Self-Assembled Nanostructures, Langmuir, 2010, 26(20), 15734–15744 Search PubMed.
- E. S. Gil and S. M. Hudson, Stimuli-Reponsive Polymers and Their Bioconjugates, Prog. Polym. Sci., 2004, 29(12), 1173–1222 Search PubMed.
- Y. J. Kim and Y. T. Matsunaga, Thermo-responsive polymers and their application as smart biomaterials, J. Mater. Chem. B, 2017, 5(23), 4307–4321 Search PubMed.
- F. Doberenz, K. Zeng, C. Willems, K. Zhang and T. Groth, Thermoresponsive polymers and their biomedical application in tissue engineering–a review, J. Mater. Chem. B, 2020, 8(4), 607–628 Search PubMed.
- N. Merlet-Lacroix, E. Di Cola and M. Cloitre, Swelling and Rheology of Thermoresponsive Gradient Copolymer Micelles, Soft Matter, 2010, 6(5), 984–993 Search PubMed.
- L. A. Fielding, J. A. Lane, M. J. Derry, O. O. Mykhaylyk and S. P. Armes, Thermo-Responsive Diblock Copolymer Worm Gels in Non-Polar Solvents, J. Am. Chem. Soc., 2014, 136(15), 5790–5798 Search PubMed.
- Y. Pei, O. R. Sugita, L. Thurairajah and A. B. Lowe, Synthesis of Poly(Stearyl Methacrylate-b-3-Phenylpropyl Methacrylate) Nanoparticles in n-Octane and Associated Thermoreversible Polymorphism, RSC Adv., 2015, 5(23), 17636–17646 Search PubMed.
- M. J. Derry, O. O. Mykhaylyk and S. P. Armes, A Vesicle-to-Worm Transition Provides a New High-Temperature Oil Thickening Mechanism, Angew. Chem., Int. Ed., 2017, 56(7), 1746–1750 Search PubMed.
- M. T. Savoji, D. Zhao, R. J. Muisener, K. Schimossek, K. Schoeller, T. P. Lodge and M. A. Hillmyer, Poly(Alkyl Methacrylate)-Grafted Polyolefins as Viscosity Modifiers for Engine Oil: A New Mechanism for Improved Performance, Ind. Eng. Chem. Res., 2018, 57(6), 1840–1850 Search PubMed.
- M. J. Rymaruk, C. T. O'Brien, S. L. Brown, C. N. Williams and S. P. Armes, Effect of Core Cross-Linking on the Physical Properties of Poly(Dimethylsiloxane)-Based Diblock Copolymer Worms Prepared in Silicone Oil, Macromolecules, 2019, 52(18), 6849–6860 Search PubMed.
- W. Fu, W. Bai, S. Jiang, B. T. Seymour and B. Zhao, UCST-Type Thermoresponsive Polymers in Synthetic Lubricating Oil Polyalphaolefin (PAO), Macromolecules, 2018, 51(5), 1674–1680 Search PubMed.
- L. P. Ratcliffe, B. E. McKenzie, G. M. Le Bouëdec, C. N. Williams, S. L. Brown and S. P. Armes, Polymerization-Induced Self-Assembly of All-Acrylic Diblock Copolymers via RAFT Dispersion Polymerization in Alkanes, Macromolecules, 2015, 48(23), 8594–8607 Search PubMed.
- J. S. Park and K. Kataoka, Comprehensive and Accurate Control of Thermosensitivity of Poly(2-Alkyl-2-Oxazoline)s via Well-Defined Gradient or Random Copolymerization, Macromolecules, 2007, 40(10), 3599–3609 Search PubMed.
- R. Hoogenboom, H. M. Thijs, M. J. Jochems, B. M. van Lankvelt, M. W. Fijten and U. S. Schubert, Tuning the LCST of Poly(2-Oxazoline)s by Varying Composition and Molecular Weight: Alternatives to Poly(N-Isopropylacrylamide)?, Chem. Commun., 2008,(44), 5758–5760 Search PubMed.
- H. M. L. Lambermont–Thijs, R. Hoogenboom, C. A. Fustin, C. Bomal–D'Haese, J. F. Gohy and U. S. Schubert, Solubility Behavior of Amphiphilic Block and Random Copolymers Based on 2-Ethyl-2-Oxazoline and 2-Nonyl-2-Oxazoline in Binary Water–Ethanol Mixtures, J. Polym. Sci., Part A: Polym. Chem., 2009, 47(2), 515–522 Search PubMed.
- M. Glassner, B. Verbraeken, V. V. Jerca, K. Van Hecke, J. Tsanaktsidis and R. Hoogenboom, Poly (2-Oxazoline)s with Pendant Cubane Groups, Polym. Chem., 2018, 9(39), 4840–4847 Search PubMed.
- I. Muljajew, C. Weber, I. Nischang and U. S. Schubert, PMMA-g-OEtOx Graft Copolymers: Influence of Grafting Degree and Side Chain Length on the Conformation in Aqueous Solution, Materials, 2018, 11(4), 528 Search PubMed.
- C. Weber, A. Krieg and R. M. Paulus, Lambermont–Thijs, H. M., Becer, C. R., Hoogenboom, R., Schubert, U. S., Thermal Properties of Oligo(2-Ethyl-2-Oxazoline) Containing Comb and Graft Copolymers and their Aqueous Solutions, Macromol. Symp., 2011, 308(1), 17–24 Search PubMed.
- H. M. L. Lambermont-Thijs, H. P. C. van Kuringen, J. P. W. van der Put, U. S. Schubert and R. Hoogenboom, Temperature Induced Solubility Transitions of Various Poly(2-Oxazoline)s in Ethanol-Water Solvent Mixtures, Polymers, 2010, 2(3), 188–199 Search PubMed.
- R. Hoogenboom, H. M. Lambermont-Thijs, M. J. Jochems, S. Hoeppener, C. Guerlain, C. A. Fustin, J. F. Gohy and U. S. Schubert, A Schizophrenic Gradient Copolymer: Switching and Reversing Poly(2-Oxazoline) Micelles Based on UCST and Subtle Solvent Changes, Soft Matter, 2009, 5(19), 3590–3592 Search PubMed.
- R. Hoogenboom, H. M. L. Thijs, D. Wouters, S. Hoeppener and U. S. Schubert, Tuning Solution Polymer Properties by Binary Water–Ethanol Solvent Mixtures, Soft Matter, 2008, 4(1), 103–107 Search PubMed.
- K. I. Seno, A. Date, S. Kanaoka and S. Aoshima, Synthesis and solution properties of poly (vinyl ether) s with long alkyl chain, biphenyl, and cholesteryl pendants, J. Polym. Sci., Part A: Polym. Chem., 2008, 46(13), 4392–4406 Search PubMed.
- T. Yoshida, K. I. Seno, S. Kanaoka and S. Aoshima, Stimuli-Responsive Reversible Physical Networks. I. Synthesis and Physical Network Properties of Amphiphilic Block and Random Copolymers with Long Alkyl Chains by Living Cationic Polymerization, J. Polym. Sci., Part A: Polym. Chem., 2005, 43(6), 1155–1165 Search PubMed.
- R. Hoogenboom, M. W. Fijten, H. M. Thijs, B. M. van Lankvelt and U. S. Schubert, Microwave-assisted synthesis and properties of a series of poly (2-alkyl-2-oxazoline)s, Des. Monomers Polym., 2005, 8(6), 659–671 Search PubMed.
Footnote |
† Electronic supplementary information (ESI) available: EtOx–SteOx copolymerization kinetics, TGA of copolymers, calibration method for determination of brush yield, tables containing graft-copolymers details. See DOI: 10.1039/d1py00534k |
|
This journal is © The Royal Society of Chemistry 2021 |
Click here to see how this site uses Cookies. View our privacy policy here.