DOI:
10.1039/D0PY01512A
(Paper)
Polym. Chem., 2021,
12, 991-1001
Synthesis and characterization of fully biobased polyesters with tunable branched architectures†
Received
28th October 2020
, Accepted 19th January 2021
First published on 21st January 2021
Abstract
A series of sugar-derived triols and biobased diacids were combined to prepare fully biobased branched polyesters with different structural features by melt polycondensation. By applying the BiMolecular Non-Linear Polymerization methodology (BMNLP), the molar ratio of diacid/triols was varied to access branched polyesters bearing either hydroxyl or carboxyl moieties as end groups. The structural features of the resulting polymers were scrutinized by 1H/13C NMR, and FT-IR spectroscopies, whereas DSC and TGA were used to investigate their thermal properties. The structure–property relationship of the synthesized polyesters was correlated to the structure of the triols and diacids and their molar ratio. Both prepared carboxyl-ended and hydroxyl-ended branched polyesters were amorphous with relatively low glass transition temperatures ranging between −57 and −18 °C for hydroxyl terminated polyesters while for carboxyl terminated ones, they oscillate between −37 and 19 °C. All these polyesters exhibit good heat resistance with onset degradation temperature Td,5% ranging from 180 to 268 °C and from 168 to 236 °C for COOH- and OH-end groups-bearing polymers series, respectively. The structural features and properties of the resulting branched fully biobased polyesters make them not only potential candidates for a wide range of applications but also as intermediate substrates for further chemical modifications and/or chain extension to access a wide range of functional (co)polymer materials.
1. Introduction
Bio-based polymers have witnessed a spiralling growth of interest, shaping thus the future of sustainable plastics as one of the most interesting domains in materials science.1–5 The development of this type of eco-friendly polymers will not only alleviate our overwhelming reliance on fossil feedstock, but providing also new materials with a significantly better environmental footprint and enhanced performances with respect to those of their petroleum-based counterparts.6–8 Among the different classes of polymers, polyesters are the most rapidly growing with the largest market share of bioplastics.9 Linear biobased polyesters, either aliphatic or aromatic, are one of the most actively investigated polymer families. This stems primarily from their interesting properties, transparency, reusability and low cost and they have received a tremendous level of interest.10,11 A distinguished bunch of biobased polyesters such as poly(L-lactic acid) (PLA), furanoate co/polyesters,12–21 poly(alkylene succinates)22–28 and others, such as poly(3-hydroxy butyrates) (PHA), poly(hydroxyl alkanoate)s (PHAs), are commercially available nowadays in various forms witnessing for the future importance of such polymers.29–31
(Hyper)-branched polyesters are a class of polymers that is attracting a tremendous level of interest due to their unique three-dimensional molecular structures that can be easily tuned by controlling the branching degree as well as their functional end groups endowing them with a wide range properties.32 These properties make them promising candidates for several applications such as supports for catalysts, and viscosity modifiers, as well as targeted drug delivery systems.33,34 As relevant example, an interesting recent review highlighting the synthetic procedures of polyglycerol hyperbranched polyesters as well as their applications in pharmaceutical technology and in biomedical applications was published by Boccaccini and Bikiaris groups.35 In this context along with the relatively good availability of suitable building blocks derived from renewable resources, particular interest has been devoted to access biobased branched-type polyesters.34,36–43 Such interest is expected to further increase under the developments currently made in the production of building blocks that will bring forth new entirely biomass-derived monomers (triols, and diacids) through optimized processes.44 This is due to the attractive features that can be easily tuned by controlling their functionality from hydroxyl to carboxylic end groups leading to very distinct properties and thus multiple uses.45–47
Hemicelluloses constitute an important feedstock for the preparation of sustainable building blocks including diols, polyols, diacids, diamines etc., through catalytic and biotechnological pathways.48,49 Polyols are the main building blocks necessary; 1,2,6-hexanetriol is such a biobased triol, which readily prepared by hydrogenolytic ring-opening of 5-hydroxymethylfurfural (HMF) which can be in turn prepared through sequential hydrolysis, isomerization, and dehydration of sugars derived from hemicellulose.50 1,2,4-Butanetriol, another eco-friendly valuable polyol extensively exploited in polyurethane foams production, is being obtained from xylose.51 A major breakthrough involving a catalytic conversion of lignocellulosic biomass, corn stover, into adipic acid has been recently reported by Han.52 In this green process, hemicellulose and cellulose derived δ-valerolactone was used as solvent and an intermediate platform chemical. Succinic acid is a further example of a highly promising biobased C4 monomer that has been identified as one of the top twelve sugar-based building blocks by the US Department of Energy.53 This renewable bifunctional acid can be prepared from biomass-derived furan compounds through a metal-free oxidative synthesis using a heterogeneous acid catalyst with hydrogen peroxide.53
To the best of our knowledge, the investigation of structure–properties relationship of branched polyesters prepared from hemicellulose-derived building blocks, mentioned above, is examined herein for the first time. Apart from these sugar-derived diacid, a vegetable oil-derived bifunctional diacid monomer, namely azelaic acid, was also used to shed light on the effect of the chain length of the acid part on the properties of the polyesters. This diacid is extracted from plant source or prepared from oleic acid through its ozonolysis.54,55 In this context, the aim of this work was to prepare through melt polycondensation fully biobased branched polyesters, wherein the effect of structural features of the used building blocks, namely aliphatic diacid with triols on the final properties the resulting polymers, were deeply investigated.
2. Experimental section
2.1. Materials
1,2,6-Hexanetriol (HTO, purum 96%), 1,2,4-butanetriol (BTO, purum > 90%), succinic acid (SucA, purum 99%), adipic acid (AdiA, purum 99.5%) and azelaïc acid (AzeA, purum 98%), reagents, tertrabutyl titanate (TBT) catalyst, were purchased from Sigma-Aldrich. All other solvents and materials used were of analytical grade.
2.2. Polymer synthesis
The BiMolecular Non-Linear Polymerization methodology (BMNLP) was used to set the conditions for the synthesis of BPE by step-growth polymerization approach, in order to adjust their structural features particularly their end groups.56 As relevant reported examples, but not limited to, BMNLP strategy has been used to synthesize various hyperbranched polyurethanes, polyesters, polyethers, polyamides, as well as polycarbosiloxanes and polysiloxanes.57–62 Developed by Flory and Stockmayer,63,64 this method allows the prediction of a number of properties of the branching reaction which can undergo gelation by establishing the criterion for the gelation of the reactive system in terms of molar ratios of the reactive groups. In this method, the critical conditions for gelation to accommodate arbitrary functionalities of the reactive monomers in an [aAx + bBy] system where x ≥ 2 and y ≥ 3 are generalized in the following equation:
with r as the ratio of the number of A and B groups initially present in the system and pA and pB the probability that that groups A or B respectively have reacted between them at any stage of the reaction.
Hence, if A is the minor component a soluble branched material is obtained when
and if B is the minor component, it will obtained when
pB2/
r < 1/(
x − 1)(
y − 1).
Thus, for example if all B groups present in the aAx + bBy system react, a branched polymer having A end-groups will be formed without gelation if: r > (x − 1)(y − 1) with r = ax/by.
And if all A groups react, a soluble branched polymer with B end-groups will be formed if
Based on this method it is assumedly possible to predict the chain termination of branched polyesters. Thus, polyesters derived from BTO or HTO with AzeA, SucA or AdiA with either hydroxyl or carboxyl end-chain end groups were synthetized and characterized. In the present work, A is corresponding to di-carboxylic acid compounds such as AzeA, SucA or AdiA (x = 2) and B to multifunctional alcohols such as BTO and HTO (y = 3). Hence, the reaction system corresponds to [aA2 + bB3], and therefore it is expected to form a branched polyester material by a step-growth polymerization reaction in equimolar quantities of functional groups.
2.2.1 BPE bearing carboxyl end groups.
BPE samples bearing COOH end-groups were synthesized via the two-stage polycondensation procedure (esterification and polycondensation) in an autoclave reactor equipped with mechanical stirrer. Predetermined amount of SucA, AdiA or AzeA diacid and BTO or HTO aliphatic triol with molar ratio of diacid
:
triol of 4
:
1 were fed into the reactor with 400 ppm of TBT catalyst. In the first stage, esterification reaction was carried out under a mild nitrogen flow at the temperature range of 160–190 °C. This reaction lasted for 4 h (1.5 h at 160 °C, 1 h at 170 °C, 1 h at 180 °C, and for an additional 0.5 h at 190 °C). In the second step, the polycondensation was performed under a high vacuum of 100 mbar slowly applied over a time of 20 min to minimize the oligomers sublimation. The temperature of the mixture was raised to 210–230 °C stepwise (1.5 h at 210 °C, 1 h at 220 °C, and for 0.5 h at 230 °C). To purify the resulting BEPs bearing carboxylic acid end groups by removing unreacted monomers, after cooling down the system to room temperature, the produced BPEs were recovered and dissolved in DMSO and then precipitated in large volume of water (10 times the volume of DMSO) and recovered by filtration. This operation is repeated three times to ensure the elimination of all unreacted monomers and finally the recovered polymers were thoroughly washed by water to remove any residual DMSO before being dried to constant weight at 80 °C under vacuum. The four BPEs derived from AzeA and AdiA showed waxy-like, while the other two SucA-based BPEs were obtained in powder form. The resulting BPE samples are labelled as follow: SucA/BTO (–COOH), SucA/HTO (–COOH), AdiA/BTO (–COOH), AzeA/BTO (–COOH), AdiA/HTO (–COOH), and AzeA/HTO (–COOH).
2.2.2. BPEs bearing hydroxyl end groups.
BPE products with free –OH end-groups were prepared using exactly the same procedure applied for carboxyl end groups-bearing BPEs. The only difference in synthesis protocol is the molar ratio of the introduced reagents, in which the molar feed amounts of diacid/triol used was 1/3. The synthesized PBEs bearing hydroxyl end groups were purified using similar procedure as for BEPs bearing carboxylic end groups. Briefly they were first dissolved in DMSO followed by precipitation in water (using 10 times volume of DMSO) and then filtration. They were then washed by water to remove any residual DMSO and dried to constant weight under vacuum at 80 °C. These BPE samples, obtained as highly viscous liquid, are tagged as follow: SucA/BTO (–OH), SucA/HTO (–OH), AdiA/BTO (–OH), AzeA/BTO (–OH), AdiA/HTO (–OH), and AzeA/HTO (–OH).
2.3. Polymer characterization
2.3.1. Nuclear magnetic resonance (NMR).
1H and 13C NMR spectra were recorded at a frequency of 600 MHz on an AVANCE III HD Bruker spectrometer. Connectivity of the compounds was studied using COSY, HSQC and HMBC 2D NMR spectroscopy. Polyesters were prepared by dissolving the sample in deuterated dimethyl sulfoxide (DMSO-d6). Proton and carbon NMR chemical shifts are referenced to that of tetramethylsilane (TMS).
2.3.2. Gel permeation chromatography (GPC).
GPC was carried out to ascertain the average molecular weights (Mn) and dispersity (PDI = Mw/Mn) of all prepared polyesters. A 1260 Infinity II gel permeation chromatograph (GPC, Agilent Technologies, USA) was used, equipped with a refractive index detector at 35 °C, using an Agilent PLgel MIXED-C column. DMF was used as eluent (0.5 ml min−1) at 35° C for branched polyesters. Universal calibration was made by using polystyrene standards (Agilent EasiVial PS-M and PSS Polymer Standards), with Mp oscillating from 160 to 1
500
000.
2.3.3. Differential scanning calorimetry (DSC).
Thermal transition behaviour of all prepared polyesters was recorded with a METTLER TOLEDO instrument. Approximately 5–7 mg of sample, sealed in aluminium pan, was heated under nitrogen from −80 to 150 °C at a heating rate of 10 °C min−1. The sample was then cooled down to −80 °C at 10 °C min−1 and held at this temperature for 10 min to erase any thermal history and heated again for the second heating scan from −80 °C to 200 °C with a heating rate of 10 °C min−1.
2.3.4. Thermo-gravimetric analysis (TGA).
Thermogravimetry analysis measurements were performed using a METTLER TOLEDO instrument. Polyesters samples were heated under nitrogen flow from room temperature to 700 °C at heating rate of 10 °C min−1.
3. Results and discussion
3.1. Synthesis and structural characterization of branched polyesters (BPE)
The main synthesis routes used to prepare branched polyesters could be divided into two major categories,65 that are, single-monomer methodology (SMM) and double-monomer methodoly (DMM). Polycondensation of ABn monomers, proton-transfer polymerization (PTP), self-condensing ring-opening polymerization (SCROP), and self-condensing vinyl polymerization (SCVP) are some of the specific approaches of the first synthetic technique, SMM.64 In contrast, DMM category involves the direct polymerization of two monomers types in order to generate branched polymers.64 As presented in Scheme 1, branched polyester samples with either hydroxyl or carboxyl end groups were synthesized in the bulk according to Bimolecular Non-Linear Polymerization (BMNLP) approach. In the esterification stage, predetermined amounts of the diacid (SucA, AdiA or AzeA) and triol (BTO or HTO) were charged in presence of TBT catalyst into an equipped reactor with a mechanical stirrer. The mixture was heated in the 160–190 °C temperature range and the reaction was proceeded for 4 h under nitrogen atmosphere. In the second step of synthesis, the polycondensation stage was conducted at 210–230 °C and was kept proceeding for 3 h under high vacuum condition of 100 mbar. A molar ratio of diacid/triol of 4/1 or 1/3 was used to prepare BPEs bearing carboxyl and hydroxyl end groups, respectively.
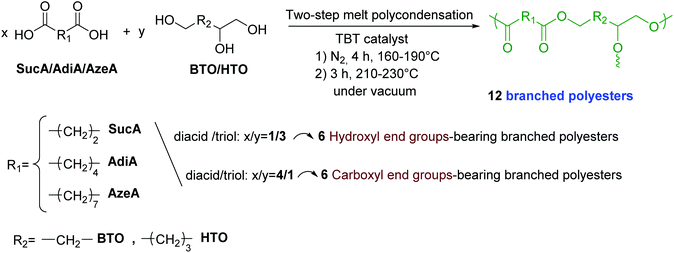 |
| Scheme 1 Synthesis route of branched polyester-type oligomers. | |
The yields of the branched polyesters materials obtained herein ranged between 80–85% for those bearing hydroxyl-end groups while the yields were lower (60–67%) for carboxyl-ended ones owing the lower solubility and loss of materials during the purification step. Both kind of prepared BPEs showed low molecular weights to be in 2730–4860 g mol−1 range (Table 1), regardless their terminal units’ type (COOH or OH). These results were speculated owing to the unique macromolecular architectures of the resulting branched networks. High dispersity indices in the 2.8–3.9 range were obtained for both hydroxyl and carboxyl terminated branched polyesters. These high PDI values presented in Table 1 are typical of branched structures.66,67 It is worth mentioning that these Mn values determined by GPC in DMF give only estimations rather than absolute values giving the lack of standard references for such kind of polymers.
Table 1 Molecular weights, dispersity indices, and yields of branched polyesters with carboxyl and hydroxyl end-groups
Polymers samples |
Yield (%) |
M
n
(g mol−1) |
PDIb |
Determined by GPC analysis.
Determined as Mw/Mn.
|
SucA/BTO (–COOH) |
67 |
4250 |
3.1 |
SucA/HTO (–COOH) |
64 |
4300 |
3.7 |
AdiA/BTO (–COOH) |
65 |
4720 |
2.8 |
AdiA/HTO (–COOH) |
60 |
3850 |
3.9 |
AzeA/BTO (–COOH) |
66 |
4860 |
3.4 |
AzeA/HTO (–COOH) |
61 |
3350 |
2.9 |
SucA/BTO (–OH) |
83 |
2980 |
3.9 |
SucA/HTO (–OH) |
85 |
3540 |
3.7 |
AdiA/BTO (–OH) |
80 |
3070 |
3.3 |
AdiA/HTO (–OH) |
80 |
3680 |
3.0 |
AzeA/BTO (–OH) |
84 |
2730 |
2.9 |
AzeA/HTO (–OH) |
82 |
3190 |
3.4 |
The chemical structure of all carboxyl and hydroxyl-terminated branched polyester samples was characterized by FT-IR spectroscopy, and the results are illustrated in Fig. 1. The obtained data serves as supportive and complementary evidence for the 1H and 13C NMR results (to be discussed later). FTIR spectra of both branched polyesters series either with terminal –COOH groups or –OH end groups-bearing polyesters showed two absorption bands at 2940 and 2870 cm−1 assigned to the aliphatic C–H symmetric and asymmetric stretching. Another typical band near 1170 cm−1 is due to C–O–C stretching mode of the ester group. The main difference in FTIR spectra of all branched polyesters lies in the fact that the hydroxyl-terminated samples revealed a wide absorption band centered at around 3370 cm−1. The latter is due to the stretching vibration of hydroxyl group (Fig. 1b). In contrast, for carboxyl-terminated BPEs samples, the aforementioned peak at 3370 cm−1 assigned to terminal (–OH) has disappeared and replaced by a broadened detectable band between 3100 and 3350 cm−1, originating from the free carboxyl functional groups (Fig. 1a). Comparison with the carboxyl-terminated branched polyesters illustrates that the characteristic absorption bands originated from ester carbonyl stretching (vC
O) in hydroxyl-terminated BPEs were shifted to higher wavenumbers (from 1720 to 1727 cm−1). This is understandable owing to the formation of strong hydrogen-bonding interactions between the carbonyl of the ester moiety and the free terminal –COOH groups in carboxyl-terminated BPEs networks.68 This hence leads to a pronounced downshift of 13–18 cm−1 in (vC
O) stretching frequency. Importantly, for all COOH-terminated BPEs, in addition to the characteristic ester carbonyl band at 1720 cm−1, an additional band typical of the free carboxylic acid carbonyl appeared at around 1733 cm−1. The presence of the latter, which overlapped with the main ester bond (vC
O), prominently proves the successful synthesis of BPEs materials with carboxyl end groups. Similar finding was described in literature.69
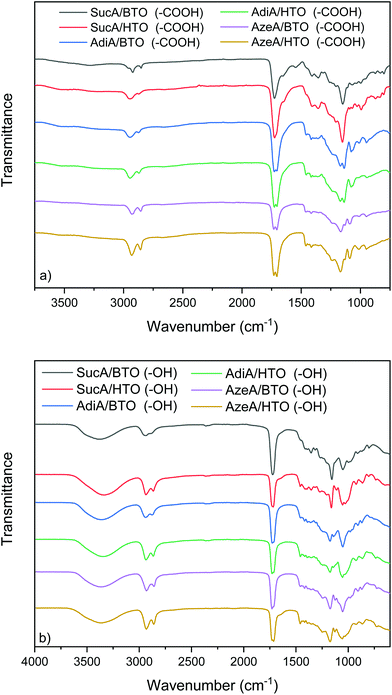 |
| Fig. 1 FT-IR spectra of branched polyesters bearing: (a) terminal carboxyl, and (b) terminal hydroxyl groups. | |
In order to gain better insight into their fine chemical structure, all the novel branched polyesters developed in the present work were studied by 1D and 2D NMR spectroscopy. The individual spin systems of the alcohols and acids could be identified using COSY and multiplicity edited HSQC allows to differentiate the alcohols’ CH, CH2 and free OH groups. The connectivity between the carbon atoms and in particular between the acid and ester carbonyl groups was ascertained using HMBC. All the 1D and 2D spectra are given in the SI (Fig. S1–S60†). The 1H and 13C NMR chemical shifts of attributed to all protons and carbons in the acid and hydroxyl-ended branched polyesters samples are reported in Tables S1–4.†
In order to explain the assignment process, the spectrum of AdiA/BTO (–COOH) was considered as example. Both the 1H (Fig. 2 top) and 13C (Fig. 2 left) feature multiple signals for most atoms due to the multiple isomeric forms present. There are several signals in the carbonyl region, at 174.7, as well as beween 173.2 and 172.5 ppm, the most deshielded of which corresponds to the acid function, while the others are esters. This is confirmed by the cross peaks between triol resonances and the high-field signal in the HMBC (Fig. 2, green). On this basis both α and α′ resonances can be assigned to the signals at 2.3 and 2.2 ppm, respectively. The absence of remaining unreacted diacid can be warranted on the basis of the integrals for these two latter signals. The signals for at least the major forms could be identified on the basis of the HSQC (Fig. 2, red and blue), HMBC (Fig. 2, green) and COSY (Fig. S13†) cross-peaks.
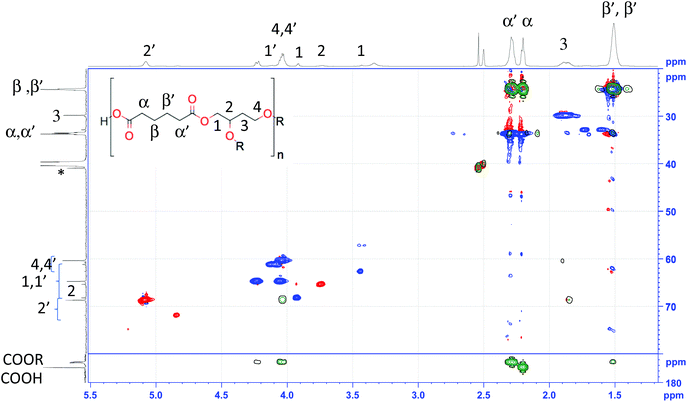 |
| Fig. 2 Superposition of HSQC (red and blue) and HMBC (green) spectra of AdiA/BTO(–COOH) (recorded in DMSO-d6) and assignments to 1H (top trace) and 13C (bottom trace) (R = H or AdiA). | |
In the case of hydroxyl-ended polyesters, AzeA/HTO(–OH) was taken as example for peaks assignments. The multiplicity edited HSQC spectrum (Fig. 3) features 2 groups of both methine and methylene signals. The HMBC spectrum (Fig. S60†) shows that the methine signals in the range 4.7–5.0 ppm 1H/70–76 ppm 13C and the methylene signals in the range between 3.8–4.2 ppm 1H/63–70 ppm 13C correlate with 13C ester signals around 173 ppm, therewith establishing that these methine and methylene signals correspond to esterified species. On the other hand, the methine signals in the range between 3.3–3.7 ppm 1H/65–73 ppm 13C and the methylene signals in the range between 3.2–3.5 ppm 1H/60–68 ppm 13C do not show any such correlations and therefore correspond to non-esterified groups. This fact is corroborated by the COSY cross-peaks these latter resonances show with the hydroxyl signals at 4.3–4.5 ppm (see Fig. S58†). The hydroxy signals are clearly identified as such by the absence of any cross-peak at these positions in the HSQC spectrum (Fig. 3).
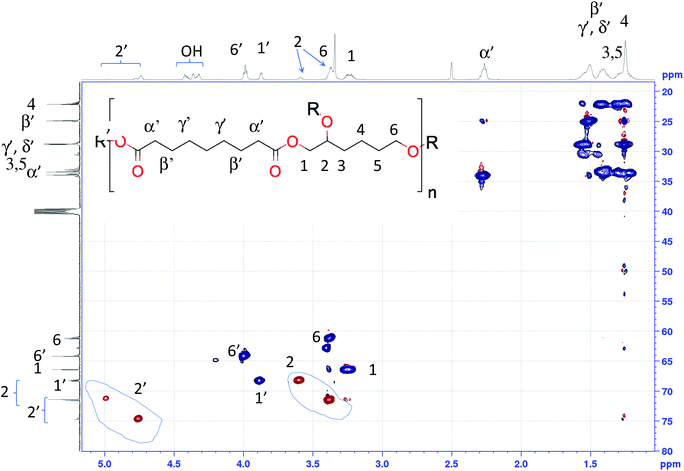 |
| Fig. 3 HSQC NMR spectra of AzeA/HTO(–OH) (recorded in DMSO-d6) and assignments to 1H (top trace) and 13C (left trace) spectra (R = H or AzeA, R′ = H or HTO). | |
The estimation of the –COOH end groups was performed by the integration of their respective peaks in the NMR spectra. The results showed that AdiA/BTO and AdiA/HTO have respectively a molar fraction of 0.56 and 0.68 of –COOH end groups of the total diacid units. AzeA based BPEs showed a higher amount of –COOH end groups as the molar fraction of COOH end groups was around 0.9 for both AzeA/BTO and AzeA/HTO. However, in the case of SucA-based BPEs, the estimation of COOH end groups was not possible because of the strong overlapping signals between those corresponding to esterified units and free end groups of this diacid.
Similarly, to COOH-terminated BEPs the estimation of the –OH end groups in hydroxyl terminated BEPs was also performed by the integration of the peaks of OH end groups in the NMR spectra. The results showed that the molar fraction of OH terminal groups ranged from 0.42 to 0.65. Indeed, the values of the molar fraction were as following: 0.57 for SucA/BTO, 0.62 for SucA/HTO, 0.65 AdiA/BTO, 0.42 for AdiA/HTO, 0.62 for AzeA/BTO and 0.56 for AzeA/HTO.
3.2. Thermal properties of polyesters
The thermal properties of the produced branched polyesters were analysed by DSC and TGA to thoroughly investigate the thermal properties and stability. The DSC analyses of the purified BPE samples with –COOH end groups showed that neither crystallization nor melting endotherms can be detected during the heating scans, hence confirming as expected the wholly amorphous nature of the resulting materials. The recorded data related to glass transition are collected in Table 2. It is worth noting that regardless of the used diacid type, BTO-based polyesters possess slightly higher glass transition temperatures than their counterpart derived from HTO, wherein Tg values of 19.7 vs. 18.5, −23 °C vs. −28.3 °C, and −37.1 vs. −37.7 °C were found for the samples derived from SucA, AdiA, and AzeA diacid, respectively. As expected, DSC results revealed an obvious correspondence between the Tg value and the chain length of the diacids incorporated into the synthesized polyesters. For BTO-based BPE with terminal carboxyl groups, Tg of polymers was dramatically shifted to a much higher temperature from −37.1 °C to −23 °C, and then to 19.7 °C with replacing AzeA with AdiA and SucA diacid, respectively. In fact, we also noticed that the Tg of the polyesters derived from HTO showed a very similar trend of those for BTO-based ones, where polymer containing shorter-chain dicarboxylic acid (AdiA or SucA) exhibited much higher Tg than that of the counterpart prepared from longer-chain diacid (AzeA). In other words, these findings reported herein mainly arise from the replacement of a highly flexible diacid moiety by a more rigid diacid structure which, by reducing chain mobility, results in a remarkable increase in Tg. To sum up, interestingly to highlight that the effect of the diacid type with different chain length on Tg increase was much more pronounced than that of triol type used. As it can be seen from DSC trace of AzeA/HTO (–COOH) branched polyester, an endothermic peak at temperature above the Tg (−21.4 °C) was clearly spotted. This behaviour may be ascribed to the physical aging phenomenon by enthalpy relaxation owing to a non-equilibrium state (instability) of the amorphous material in the glassy state.70 For the sake of comparison with their linear polyesters analogues, the three carboxyl-terminated branched polyesters made from BTO showed significantly higher Tg values than their linear analogues prepared from the same diacids but using 1,4-butanediol (BDO).71,72 Indeed, the linear polyesters combining SucA/BDO, AdiA/BDO and AzeA/BDO exhibited Tg values of −36 °C, −76 °C and −62 °C, respectively (vs. 19.7 °C, −23 °C, and −37.1 °C for BTO-based BPEs, respectively). Moreover, the linear polyesters were semicrystalline contrarily to the branched ones prepared herein that were amorphous. Similarly, branched carboxylic-terminated polyesters based on HTO in combination with SucA or AdiA showed much higher values with respect to their linear counterparts derived from 1,6-hexanediol (HDO).73,74 The Tg of the linear SucA/HDO and AdiA/HDO based polyesters were −40 °C and −28.3 °C respectively against 18.5 °C and −28.3 °C for BPEs based on SucA/HTO and AdiA/HTO respectively.
Table 2 Thermal properties of prepared branched polyesters bearing carboxyl and hydroxyl end-groups
Samples |
T
d,5%
|
T
d,10%
|
R
500 °C (%) |
 (°C) |
SucA/BTO (–COOH) |
216.8 |
249 |
6.9 |
19.7 |
SucA/HTO (–COOH) |
227.3 |
252.2 |
6.0 |
18.5 |
AdiA/BTO (–COOH) |
180.5 |
264.6 |
5.3 |
−23.0 |
AdiA/HTO (–COOH) |
268.3 |
303 |
3.4 |
−28.3 |
AzeA/BTO (–COOH) |
203.6 |
249.5 |
2.9 |
−37.1 |
AzeA/HTO (–COOH) |
229.1 |
260.3 |
1.8 |
−37.7 |
SucA/BTO (–OH) |
187.8 |
226.5 |
4.1 |
−18.9 |
SucA/HTO (–OH) |
213.5 |
229.7 |
1.0 |
−53.2 |
AdiA/BTO (–OH) |
168.3 |
203.0 |
4.3 |
−52.1 |
AdiA/HTO (–OH) |
226.3 |
257.5 |
3.0 |
−56.7 |
AzeA/BTO (–OH) |
193.1 |
221.0 |
2.5 |
−57.2 |
AzeA/HTO (–OH) |
236.7 |
257.7 |
1.7 |
−57.9 |
The thermal degradation behaviour and stability of the resulting carboxyl-terminal BPEs were assessed by TGA analysis under nitrogen atmosphere. The typical profiles are shown in Fig. 4 and the 5%, 10% decomposition temperatures as well as the residue at 500 °C are given in Table 2. It was found that all the prepared fully biobased polyesters, except AdiA/HTO (–COOH), revealed high thermal stability over 200 °C with onset decomposition temperatures (Td,5%) oscillating from 180.5 to 268.3 °C, leaving just 1.8–6.9% of residual weight upon heating to 500 °C. Only a single stage thermal degradation was observed for the two AdiA-based polymers, while the other four polyesters samples degraded by multi-stage process. It was found that polyester derived from HTO was much more thermally stable than the BTO-based sample. Indeed, polymers prepared from HTO showed a much higher Td,5% value of 221.7 vs. 216.8 °C (for BTO-based sample), 268.3 vs. 180.5 °C, 229.1 vs. 203.6 °C, respectively for SucA, AdiA, and AzeA-based polyesters. This finding is in good agreement with the reported TGA results in Barrett study,75 where polyesters derived from HTO showed a much higher stability compared with BTO-based samples. It seems that the presence of a long-chain hexamethylene unit (HTO) into the branched polyester backbone having –COOH functional end groups play the main role in the important delay of the onset of degradation. As shown above in Table 2, the HTO-based polyester prepared from AdiA diacid exhibited much higher heat resistance than its homologue obtained with shorter chain SucA diacid. Hence, a notable difference in Td,5% values of 41 °C was obtained for these two samples using HTO. It is worthy to note that BTO-based polymers present a similar trend as HTO-based samples, where polyester having the longest aliphatic diacid (AzeA) revealed higher thermal stability than other polyesters with shorter spacer length of diacid, AdiA (Td,5% values of 203.6 vs. 180.5 °C for AzeA/BTO (–COOH), and AdiA/BTO (–COOH), respectively). This is most likely due to the presence of a long-chain aliphatic diacid incorporated into the polyesters’ macromolecular chain, which obviously improved their thermal stability by delaying the onset of degradation Td,5%. This outcome is consistent with a previous study reporting that the longer straight aliphatic diacid chain is, the more vulnerable to thermal degradation is.76 To sum up, the above results highlighted that the superior thermal stability of the six carboxyl-terminated BPEs, derived from fully renewable resources, ensures their safe thermal processing at temperature much higher than their glass transition temperatures. This characteristic makes them suitable candidates (as polymer blend component or as co-units in copolyesters) for heat processing applications such injection molding.
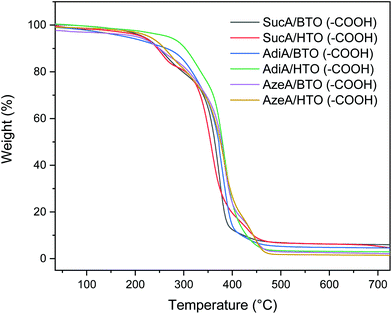 |
| Fig. 4 TGA thermograms of carboxyl-terminated branched polyesters. | |
The six synthesized branched polyesters bearing hydroxyl end groups were also analyzed by DSC to examine the effects of the flexible chains length of used both diacids and triols on their thermal behaviour characteristics. Table 2 gathers the recorded glass transition temperatures for the produced polyesters. It can be concluded from the latter that as expected all resulting hydroxyl end groups-bearing BPEs polymers did not show any crystallization or melting peaks demonstrating their amorphous nature. The new fully renewable materials exhibited a very wide glass transition temperature (Tg) range oscillating from −57.9 to −18.9 °C. As expected from the structure of BTO and HTO having different length alkane chains, we found that for the same diacid, Tg values of polymers samples derived from BTO are higher than those of the polyesters made from HTO (exhibiting values of −18.9 vs. −53.2 °C, −52.1 vs. −56.7 °C, and −57.2 vs. −57.9 °C for SucA, AdiA, and AzeA-based materials, respectively). Such outcome can be mainly ascribed to the rigidity of the obtained polyester, where one aliphatic triol moiety (HTO) is substituted by a stiffer triol moiety (BTO). This endows less mobility to the chain, resulting thereby to higher Tg value. Comparing the thermal properties results of the sustainable BPEs with –OH and –COOH end groups, a great difference could be readily spotted independently from the used biobased triol (BTO or HTO). Interestingly, much higher Tg were recorded for all synthesized BPEs with terminal carboxyl groups compared to those of the same samples bearing –OH end groups. SucA-based polyesters have been used as relevant example for illustrative purposes. Indeed, the Tg value of SucA/HTO sample dramatically increased from −53.2 to 18.5 °C with changing the end groups type from –OH to –COOH ones, respectively. We noticed that SucA/BTO polyesters showed also the same discernible trend as that in SucA/HTO sample, but the hydroxyl groups-terminated polymer revealed a much lower Tg value compared to the same branched polyester having carboxyl functions as terminal units (−18.9 vs. 19.7 °C). As seen from DSC data, the other AdiA and AzeA-based polyesters also show the same tendency. Once the hydroxyl end groups were replaced by carboxyl terminal ones into the same polymer sample, a remarkable increment of their Tg is observed regardless the involved triol (BTO or HTO). In fact, a huge difference in the Tg at least by 20 °C was detected between BPE samples terminated with OH and COOH functional end groups. This significant Tg increase in carboxyl-terminated polymers could be explained by the formation of hydrogen bonding between the free carboxyl end groups of polyesters. This promotes much greater intermolecular cohesion than dipole–dipole interaction, thereby obtaining higher Tg values in branched polyesters bearing –COOH end groups. This finding is in good agreement with what was reported in a previous work in literature.77 To recapitulate, the aforementioned upshots proved that the Tg of all twelve prepared branched polyesters strongly depends on the nature of the end-groups (–OH or –COOH).
Thermal stability of the novel branched polyesters samples bearing hydroxyl end groups were investigated by thermogravimetric analysis as depicted in Fig. 5. All prepared polymers exhibit a classical two-step weight loss. The pertinent decomposition parameters including the degradation temperatures at 5% and 10% weight loss as well as the residue at 500 °C are listed in Table 2. A significant difference in thermal stability was observed among them across the whole temperature range. Like branched polymers bearing carboxyl end-groups, it was found that hydroxyl-terminated polyesters derived from HTO were also much more thermally stable than the BTO-based samples. These HTO-based polymers, characterized by good resistance to heat, exhibited onset degradation temperature (Td,5%) values ranging between 213.5 and 236.7 °C (vs. much lower Td,5% values appearing in the 168.3–193.1 °C range for the BTO-based samples). In more detail, a difference in Td,5% of about 25 °C, 58 °C, and 43 °C is obtained between HTO and BTO-based polymer for SucA, AdiA, and AzeA-based polyesters, respectively. The six renewable resources-based materials developed displayed low residual weight percentage at 500 °C, leaving a 1% and 4.3% of degradation residues. To recapitulate, it is worthy to highlight that one of the most outstanding features of these new branched polyesters bearing OH end groups is their very wide temperature window of processing up to 294 °C above their Tg. This feature opens the door for their exploitation as new class of thermally stable biobased materials with unique macromolecular architectures in a wide range of potential applications such as injection molding and extrusion.
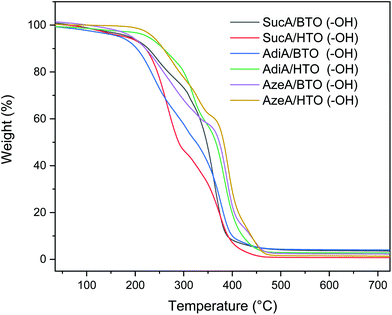 |
| Fig. 5 TGA thermograms of hydroxyl-terminated branched polyesters. | |
4. Conclusion
In this study, biobased aliphatic polyols bearing three hydroxyl groups (triols) as well as dicarboxylic acids that can be derived from renewable feedstock particularly hemicellulosic sugars were used to prepare branched polyesters through melt polycondensation method. A systematic combination of various building blocks having different structures allowed the synthesis of polymers with different structural features enabling, for the first time to our knowledge, the establishment of the structure/property relationship for such fully biobased polyesters. The understanding of these links particularly in term of thermal properties that are fundamental to determine their processability and application domains was established. The study reveals furthermore the importance of the use of sugar-derived intermediate as promising precursors for the synthesis of high-performance eco-friendly materials in various structure. This methodical study can be used as the basis for the selection of suitable building blocks and their combination to access fully biobased branched polyester with giving properties for targeted applications. Undoubtedly the potential of the synthesized final materials for various uses is undeniable but the extension to other similar building blocks as well as the various combination through co-polymerization, chain extension and/or crosslinking, etc. can be pursued paving thus the way to wide range of functional polyesters with broad application domains.
Conflicts of interest
There are no conflicts to declare.
Acknowledgements
The authors are grateful to the Luxembourg National Research Fond (FNR) for financial support (CATBIOSE project INTER/ANR 15/9903334). We would like to acknowledge Coraline Sirot, Régis Vaudemont and Benoît Marcolini who continuously provide a friendly and supportive environment to help us conduct our work.
References
- A. Gandini, Polymers from Renewable Resources: A Challenge for the Future of Macromolecular Materials, Macromolecules, 2008, 41(24), 9491–9504 CrossRef CAS.
- A. Gandini, The irruption of polymers from renewable resources on the scene of macromolecular science and technology, Green Chem., 2011, 13(5), 1061–1083 RSC.
- T. Iwata, Biodegradable and Bio-Based Polymers: Future Prospects of Eco-Friendly Plastics, Angew. Chem., Int. Ed., 2015, 54(11), 3210–3215 CrossRef CAS PubMed.
- M. A. R. Meier, J. O. Metzger and U. S. Schubert, Plant oil renewable resources as green alternatives in polymer science, Chem. Soc. Rev., 2007, 36(11), 1788–1802 RSC.
- C. Vilela, A. F. Sousa, A. C. Fonseca, A. C. Serra, J. F. J. Coelho, C. S. R. Freire and A. J. D. Silvestre, The quest for sustainable polyesters – insights into the future, Polym. Chem., 2014, 5(9), 3119–3141 RSC.
- V. Guillard, S. Gaucel, C. Fornaciari, H. Angellier-Coussy, P. Buche and N. Gontard, The Next Generation of Sustainable Food Packaging to Preserve Our Environment in a Circular Economy Context, Front. Nutr., 2018, 5(121), 1–13 Search PubMed.
- S. Lambert and M. Wagner, Environmental performance of bio-based and biodegradable plastics: the road ahead, Chem. Soc. Rev., 2017, 46(22), 6855–6871 RSC.
- F. Luzi, L. Torre, J. M. Kenny and D. Puglia, Bio- and Fossil-Based Polymeric Blends and Nanocomposites for Packaging: Structure–Property Relationship, Materials, 2019, 12(3), 471 CrossRef CAS PubMed.
- European bioplastics. Institute for Bioplastics and Biocomposites, https://www.european-bioplastics.org.
- Z. Wang, B. Kastern, K. Randazzo, A. Ugrinov, J. Butz, D. W. Seals, M. P. Sibi and Q. R. Chu, Linear polyester synthesized from furfural-based monomer by photoreaction in sunlight, Green Chem., 2015, 17(10), 4720–4724 RSC.
- C. Lavilla, A. Alla, A. Martínez de Ilarduya and S. Muñoz-Guerra, High Tg Bio-Based Aliphatic Polyesters from Bicyclic d-Mannitol, Biomacromolecules, 2013, 14(3), 781–793 CrossRef CAS PubMed.
- P. A. Klonos, L. Papadopoulos, G. Z. Papageorgiou, A. Kyritsis, P. Pissis and D. N. Bikiaris, Interfacial Interactions, Crystallization, and Molecular Dynamics of Renewable Poly(Propylene Furanoate) In Situ Filled with Initial and Surface Functionalized Carbon Nanotubes and Graphene Oxide, J. Phys. Chem. C, 2020, 124(18), 10220–10234 CrossRef CAS.
- H. Hu, R. Zhang, W. B. Ying, Z. Kong, K. Wang, J. Wang and J. Zhu, Biodegradable Elastomer from 2,5-Furandicarboxylic Acid and ε-Caprolactone: Effect of Crystallization on Elasticity, ACS Sustainable Chem. Eng., 2019, 7(21), 17778–17788 CrossRef CAS.
- J. C. Morales-Huerta, A. Martínez de Ilarduya, S. León and S. Muñoz-Guerra, Isomannide-Containing Poly(butylene 2,5-furandicarboxylate) Copolyesters via Ring Opening Polymerization, Macromolecules, 2018, 51(9), 3340–3350 CrossRef CAS.
- D. G. Papageorgiou, N. Guigo, V. Tsanaktsis, S. Exarhopoulos, D. N. Bikiaris, N. Sbirrazzuoli and G. Z. Papageorgiou, Fast Crystallization and Melting Behavior of a Long-Spaced Aliphatic Furandicarboxylate Biobased Polyester, Poly(dodecylene 2,5-furanoate), Ind. Eng. Chem. Res., 2016, 55(18), 5315–5326 CrossRef CAS.
- G. Z. Papageorgiou, D. G. Papageorgiou, Z. Terzopoulou and D. N. Bikiaris, Production of bio-based 2,5-furan dicarboxylate polyesters: Recent progress and critical aspects in their synthesis and thermal properties, Eur. Polym. J., 2016, 83, 202–229 CrossRef CAS.
- G. Z. Papageorgiou, V. Tsanaktsis, D. G. Papageorgiou, K. Chrissafis, S. Exarhopoulos and D. N. Bikiaris, Furan-based polyesters from renewable resources: Crystallization and thermal degradation behavior of poly(hexamethylene 2,5-furan-dicarboxylate), Eur. Polym. J., 2015, 67, 383–396 CrossRef CAS.
- M. J. Soares, P.-K. Dannecker, C. Vilela, J. Bastos, M. A. R. Meier and A. F. Sousa, Poly(1,20-eicosanediyl 2,5-furandicarboxylate), a biodegradable polyester from renewable resources, Eur. Polym. J., 2017, 90, 301–311 CrossRef CAS.
- N. Poulopoulou, A. Pipertzis, N. Kasmi, D. N. Bikiaris, D. G. Papageorgiou, G. Floudas and G. Z. Papageorgiou, Green polymeric materials: On the dynamic homogeneity and miscibility of furan-based polyester blends, Polymer, 2019, 174, 187–199 CrossRef CAS.
- Z. Terzopoulou, L. Papadopoulos, A. Zamboulis, D. G. Papageorgiou, G. Z. Papageorgiou and D. N. Bikiaris, Tuning the Properties of Furandicarboxylic Acid-Based Polyesters with Copolymerization: A Review, Polymers, 2020, 12(6), 1209 CrossRef CAS PubMed.
- Z. Terzopoulou, V. Tsanaktsis, D. N. Bikiaris, S. Exarhopoulos, D. G. Papageorgiou and G. Z. Papageorgiou, Biobased poly(ethylene furanoate-co-ethylene succinate) copolyesters: solid state structure, melting point depression and biodegradability, RSC Adv., 2016, 6(87), 84003–84015 RSC.
- D. N. Bikiaris, G. Z. Papageorgiou and D. S. Achilias, Synthesis and comparative biodegradability studies of three poly(alkylene succinate)s, Polym. Degrad. Stab., 2006, 91(1), 31–43 CrossRef CAS.
- N. Bosq, N. Guigo, D. Aht-Ong and N. Sbirrazzuoli, Crystallization of Poly(butylene succinate) on Rapid Cooling and Heating: Toward Enhanced Nucleation by Graphene Nanosheets, J. Phys. Chem. C, 2017, 121(21), 11915–11925 CrossRef CAS.
- M. Gigli, M. Fabbri, N. Lotti, R. Gamberini, B. Rimini and A. Munari, Poly(butylene succinate)-based polyesters for biomedical applications: A review, Eur. Polym. J., 2016, 75, 431–460 CrossRef CAS.
- D. N. Bikiaris, G. Z. Papageorgiou, S. A. Papadimitriou, E. Karavas and K. Avgoustakis, Novel biodegradable polyester poly(propylene succinate): synthesis and application in the preparation of solid dispersions and nanoparticles of a water-soluble drug, AAPS PharmSciTech, 2009, 10(1), 138–146 CrossRef CAS PubMed.
- J. C. Morales-Huerta, A. Martínez de Ilarduya and S. Muñoz-Guerra, Modulating the Tg of Poly(alkylene succinate)s by Inserting Bio-Based Aromatic Units via Ring-Opening Copolymerization, Polymers, 2017, 9(12), 701 CrossRef PubMed.
- G. Z. Papageorgiou and D. N. Bikiaris, Biodegradable poly(alkylene succinate) blends: Thermal behavior and miscibility study, J. Polym. Sci., Part B: Polym. Phys., 2006, 44(3), 584–597 CrossRef CAS.
- G. Z. Papageorgiou and D. N. Bikiaris, Synthesis, Cocrystallization, and Enzymatic Degradation of Novel Poly(butylene-co-propylene succinate) Copolymers, Biomacromolecules, 2007, 8(8), 2437–2449 CrossRef CAS PubMed.
- Z. Li, J. Yang and X. J. Loh, Polyhydroxyalkanoates: opening doors for a sustainable future, NPG Asia Mater., 2016, 8, e265 CrossRef CAS.
- J. Xu and B.-H. Guo, Poly(butylene succinate) and its copolymers: Research, development and industrialization, Biotechnol. J., 2010, 5(11), 1149–1163 CrossRef CAS PubMed.
- T. Yokohara, K. Okamoto and M. Yamaguchi, Effect of the shape of dispersed particles on the thermal and mechanical properties of biomass polymer blends composed of poly(L-lactide) and poly(butylene succinate), J. Appl. Polym. Sci., 2010, 117(4), 2226–2232 CrossRef CAS.
- K. K. Jena, K. V. S. N. Raju, B. Prathab and T. M. Aminabhavi, Hyperbranched Polyesters: Synthesis, Characterization, and Molecular Simulations, J. Phys. Chem. B, 2007, 111(30), 8801–8811 CrossRef CAS PubMed.
- L. Y. Qiu and Y. H. Bae, Polymer Architecture and Drug Delivery, Pharm. Res., 2006, 23(1), 1–30 CrossRef CAS PubMed.
- H. Zhang, A. Patel, A. K. Gaharwar, S. M. Mihaila, G. Iviglia, S. Mukundan, H. Bae, H. Yang and A. Khademhosseini, Hyperbranched Polyester Hydrogels with Controlled Drug Release and Cell Adhesion Properties, Biomacromolecules, 2013, 14(5), 1299–1310 CrossRef CAS PubMed.
- A. Zamboulis, E. A. Nakiou, E. Christodoulou, D. N. Bikiaris, E. Kontonasaki, L. Liverani and A. R. Boccaccini, Polyglycerol Hyperbranched Polyesters: Synthesis, Properties and Pharmaceutical and Biomedical Applications, Int. J. Mol. Sci., 2019, 20(24), 6210 CrossRef CAS.
- T. Gurunathan, S. Mohanty and S. K. Nayak, Hyperbranched Polymers for Coating Applications: A Review, Polym.-Plast. Technol. Eng., 2016, 55(1), 92–117 CrossRef CAS.
- S. Ma, J. Qian, Q. Zhuang, X. Li, W. Kou and S. Peng, Synthesis and application of water-soluble hyperbranched polyester modified by trimellitic anhydride, J. Macromol. Sci., Part A: Pure Appl.Chem., 2018, 55(5), 414–421 CrossRef CAS.
- X. Zhang, Modifications and applications of hyperbranched aliphatic polyesters based on dimethylolpropionic acid, Polym. Int., 2011, 60(2), 153–166 CrossRef CAS.
- L.-M. Tang, T. Qiu, X.-L. Tuo, X.-L. Zhang and D.-S. Liu, Synthesis, Morphology and Application of Alkylaryl Hyperbranched Polyesters, Polym. J., 2002, 34(3), 112–116 CrossRef CAS.
- M. Johansson, E. Malmström, A. Jansson and A. Hult, Novel concept for low temperature curing powder coatings based on hyperbranched polyesters, J. Coat. Technol., 2000, 72(906), 49–54 CrossRef CAS.
- D. Schmaljohann, B. I. Voit, J. F. G. A. Jansen, P. Hendriks and J. A. Loontjens, New coating systems based on vinyl ether- and oxetane-modified hyperbranched polyesters, Macromol. Mater. Eng., 2000, 275(1), 31–41 CrossRef CAS.
- Q. Wan, S. R. Schricker and B. M. Culbertson, Methacryloyl derivitized hyperbranched polyester. 1. Synthesis, characterization, and copolymerization, J. Macromol. Sci., Part A: Pure Appl.Chem., 2000, 37(11), 1301–1315 CrossRef.
- G. Belge, D. Beyerlein, C. Betsch, K.-J. Eichhorn, G. Gauglitz, K. Grundke and B. Voit, Suitability of hyperbranched polyester for sensoric applications – investigation with reflectometric interference spectroscopy, Anal. Bioanal. Chem., 2002, 374(3), 403–411 CrossRef CAS PubMed.
- A. Gandini and T. M. Lacerda, From monomers to polymers from renewable resources: Recent advances, Prog. Polym. Sci., 2015, 48, 1–39 CrossRef CAS.
- T. T. Hsieh, C. Tiu and G. P. Simon, Melt rheology of aliphatic hyperbranched polyesters with various molecular weights, Polymer, 2001, 42(5), 1931–1939 CrossRef CAS.
- T.-T. Hsieh, C. Tiu and G. P. Simon, Rheological behaviour of polymer blends containing only hyperbranched polyesters of varying generation number, Polymer, 2001, 42(18), 7635–7638 CrossRef CAS.
- C. M. Nunez, B.-S. Chiou, A. L. Andrady and S. A. Khan, Solution Rheology of Hyperbranched Polyesters and Their Blends with Linear Polymers, Macromolecules, 2000, 33(5), 1720–1726 CrossRef CAS.
- F. H. Isikgor and C. R. Becer, Lignocellulosic biomass: a sustainable platform for the production of bio-based chemicals and polymers, Polym. Chem., 2015, 6(25), 4497–4559 RSC.
-
X.-J. Ji, H. Huang, Z.-K. Nie, L. Qu, Q. Xu and G. T. Tsao, in Fuels and Chemicals from Hemicellulose Sugars. In Biotechnology in China III: Biofuels and Bioenergy, ed. F.-W. Bai, C.-G. Liu, H. Huang and G. T. Tsao, Springer Berlin Heidelberg, Berlin, Heidelberg, 2012, pp. 199–224 Search PubMed.
-
J. Ohyama and A. Satsuma, Reductive Conversion of 5-Hydroxymethylfurfural in Aqueous Solutions by Furan Ring Opening and Rearrangement, in Production of Biofuels and Chemicals with Bifunctional Catalysts, ed. Z. Fang, R. L. Smith Jr and H. Li, Springer Singapore, Singapore, 2017, pp. 159–185 Search PubMed.
- A. Lachke, Biofuel fromD-xylose—The second most abundant sugar, Resonance, 2002, 7(5), 50–58 CrossRef CAS.
- J. Han, A bio-based ‘green’ process for catalytic adipic acid production from lignocellulosic biomass using cellulose and hemicellulose derived γ-valerolactone, Energy Convers. Manage., 2016, 129, 75–80 CrossRef CAS.
- H. Choudhary, S. Nishimura and K. Ebitani, Metal-free oxidative synthesis of succinic acid from biomass-derived furan compounds using a solid acid catalyst with hydrogen peroxide, Appl. Catal., A, 2013, 458, 55–62 CrossRef CAS.
- A. Köckritz and A. Martin, Synthesis of azelaic acid from vegetable oil-based feedstocks, Eur. J. Lipid Sci. Technol., 2011, 113(1), 83–91 CrossRef.
- A. Soutelo-Maria, J.-L. Dubois, J.-L. Couturier and G. Cravotto, Oxidative Cleavage of Fatty Acid Derivatives for Monomer Synthesis, Catalysts, 2018, 8(10), 464 CrossRef.
- P. J. Flory, Fundamental principles of condensation polymerization, Chem. Rev., 1946, 39(1), 137–197 CrossRef CAS PubMed.
-
P. R. M. Dvornic, J. Hu, D. J. Meier, R. M. Nowak and P. L. Parham, Hyperbranched polyureas, polyurethanes, polyamidoamines, polyamides and polyesters, US Pat., US6534600B2, 2003 Search PubMed.
- J.-F. Stumbé and B. Bruchmann, Hyperbranched Polyesters Based on Adipic Acid and Glycerol, Macromol. Rapid Commun., 2004, 25(9), 921–924 CrossRef.
-
P. B. M. Smith, B. A. Howell and C. Zhang, Sustained release composition using biobased biodegradable hyperbranched polyesters, WO2015130602A2, 2015.
- N. Xu, E. J. Stark, P. R. Dvornic, D. J. Meier, J. Hu and C. Hartmann-Thompson, Hyperbranched Polycarbosiloxanes and Polysiloxanes with Octafunctional Polyhedral Oligomeric Silsesquioxane (POSS) Branch Points, Macromolecules, 2012, 45(11), 4730–4739 CrossRef CAS.
-
P. R. Dvornic and D. J. Meier, Hyperbranched Silicon-Containing Polymers via Bimolecular Non-linear Polymerization, in Silicon-Containing Dendritic Polymers, ed. P. R. Dvornic and M. J. Owen, Springer Netherlands, Dordrecht, 2009, pp. 401–419 Search PubMed.
- J. Hu, P. I. Carver, D. J. Meier, E. J. Stark, N. Xu, T. Zhang, C. Hartmann-Thompson and P. R. Dvornic, Hyperbranched polycarbosiloxanes and polycarbosilanes via bimolecular non-linear hydrosilylation polymerization, Polymer, 2012, 53(24), 5459–5468 CrossRef CAS.
- C. W. Macosko and D. R. Miller, A New Derivation of Average Molecular Weights of Nonlinear Polymers, Macromolecules, 1976, 9(2), 199–206 CrossRef CAS.
- C. Gao and D. Yan, Hyperbranched polymers: from synthesis to applications, Prog. Polym. Sci., 2004, 29(3), 183–275 CrossRef CAS.
-
S. R. Marinović, Synthesis and Applications of Hyperbranched Polyesters: A Review, in Hyperbranched Polymers: Properties, Synthesis and Applications, ed. C. R. Lewis, Nova Science Publishers, Inc., 2017, pp. 65–79 Search PubMed.
- R. K. Roy and S. Ramakrishnan, Control of Molecular Weight and Polydispersity of Hyperbranched Polymers Using a Reactive B3 Core: A Single-Step Route to Orthogonally Functionalizable Hyperbranched Polymers, Macromolecules, 2011, 44(21), 8398–8406 CrossRef CAS.
- D. P. Bernal, L. Bedrossian, K. Collins and E. Fossum, Effect of Core Reactivity on the Molecular Weight, Polydispersity, and Degree of Branching of Hyperbranched Poly(arylene ether phosphine oxide)s, Macromolecules, 2003, 36(2), 333–338 CrossRef CAS.
- B. Nie, J. Stutzman and A. Xie, A vibrational spectral maker for probing the hydrogen-bonding status of protonated Asp and Glu residues, Biophys. J., 2005, 88(4), 2833–2847 CrossRef CAS PubMed.
- B. A. J. Noordover, R. Duchateau, R. A. T. M. van Benthem, W. Ming and C. E. Koning, Enhancing the Functionality of Biobased Polyester Coating Resins through Modification with Citric Acid, Biomacromolecules, 2007, 8(12), 3860–3870 CrossRef CAS PubMed.
- M. W. Khemici, N. D. A. Gourari and M. Bendaoud, Contribution to the Study of the Enthalpy Relaxation of Polyesters by DSC Experiments, Int. J. Polym. Anal. Charact., 2012, 17(5), 358–370 CrossRef CAS.
- X. Kong, H. Qi and J. M. Curtis, Synthesis and characterization of high-molecular weight aliphatic polyesters from monomers derived from renewable resources, J. Appl. Polym. Sci., 2014, 131(15), 40579 CrossRef.
- M. S. Nikolic and J. Djonlagic, Synthesis and characterization of biodegradable poly(butylene succinate-co-butylene adipate)s, Polym. Degrad. Stab., 2001, 74(2), 263–270 CrossRef CAS.
- E. M. Woo, Y.-H. Chou, W.-J. Chiang, I. T. Chen, I. H. Huang and N.-T. Kuo, Amorphous phase behavior and crystalline morphology in blends of poly(vinyl methyl ether) with isomeric polyesters: poly(hexamethylene adipate) and poly(ε-caprolactone), Polym. J., 2010, 42(5), 391–400 CrossRef CAS.
- Z. Bai, Y. Liu, T. Su and Z. Wang, Effect of Hydroxyl Monomers on the Enzymatic Degradation of Poly(ethylene succinate), Poly(butylene succinate), and Poly(hexylene succinate), Polymers, 2018, 10(1), 90 CrossRef PubMed.
- D. G. Barrett and M. N. Yousaf, Poly(triol α-ketoglutarate) as Biodegradable, Chemoselective, and Mechanically Tunable Elastomers, Macromolecules, 2008, 41(17), 6347–6352 CrossRef CAS.
- M. Kwiecień, I. Kwiecień, I. Radecka, V. Kannappan, M. R. Morris and G. Adamus, Biocompatible terpolyesters containing polyhydroxyalkanoate and sebacic acid structural segments – synthesis and characterization, RSC Adv., 2017, 7(33), 20469–20479 RSC.
- S.-W. Kuo, H. Xu, C.-F. Huang and F.-C. Chang, Significant glass-transition-temperature increase through hydrogen-bonded copolymers, J. Polym. Sci., Part B: Polym. Phys., 2002, 40(19), 2313–2323 CrossRef CAS.
Footnote |
† Electronic supplementary information (ESI) available. See DOI: 10.1039/d0py01512a |
|
This journal is © The Royal Society of Chemistry 2021 |