DOI:
10.1039/D1NA00633A
(Paper)
Nanoscale Adv., 2022,
4, 150-162
Prominence of Cu in a plasmonic Cu–Ag alloy decorated SiO2@S-doped C3N4 core–shell nanostructured photocatalyst towards enhanced visible light activity†
Received
18th August 2021
, Accepted 17th October 2021
First published on 10th November 2021
1. Introduction
Photocatalysis with nanostructured semiconductors1 has been receiving worldwide attention for environmental remediation and solar energy conversion, akin to photocatalytic H2 generation,2 which is considered a clean and sustainable process to solve the energy crisis. After the discovery of H2 fuel generation over TiO2 in 1972,3 this method provided a new direction in the field of clean energy production. However, the UV activity4 of TiO2, due to its large band gap (3.2 eV) and its high recombination rate of photoinduced electron–hole pairs, severely hinders its practical application. To address the above-described properties of TiO2, a number of semiconductors, such as BiVO4 (ref. 5) and Ag3PO4,6 have occasionally been demonstrated as visible light-active photocatalysts. In this context, graphitic carbon nitride7 (g-C3N4) is gaining enormous attention in the field of visible light H2 generation. Metal-free polymeric catalysts8 have evoked interest due to their facile fabrication using small organic molecules such as urea or melamine, thermal stability, and solidity in diversified pH media. The suitable conduction band position of the polymer is responsible for the production of H2 upon light irradiation. The simultaneous fabrication of crystalline and N vacancy-rich g-C3N4 showed an increase in photocatalytic H2 generation by the metal free polymeric catalyst.9 A two-dimensional PCN/2D Ti3C2 MXene interface heterojunction showed an improved hydrogen evolution rate of approximately 2181 μmol−1 g−1 in comparison to the 3D bulk.10 The significantly boosted visible-light H2 generation over CN nanosheets has been observed through loading with co-catalysts such as Ni3B/Ni(OH)2, which enhances the charge separation as well as the reduction overpotential.11 The effort to replace the state-of-the-art cocatalyst, i.e. Pt, with cost-effective and abundant transition metal bimetallic alloys such as Pt–M (M = Co, Ni, and Fe) showed enhanced photocatalytic H2 generation by the polymer and by loading a co-catalyst, such as Co1.4Ni0.6P, an H2 generation value of up to 195 μmol h−1 g−1 has been achieved.12 Similarly, a Ni cocatalyst with a shell-layer of 15 nm thickness in a Ni3C@Ni/g-C3N4 photocatalyst produced 16 times higher photocatalytic H2 generation compared to Ni3C/g-C3N4.13 However, bulk g-C3N4 suffers14 rapid charge recombination owing to the π–π stacking present between the aromatic layers. Again, the stacking of tri-s-triazine layers is accountable for the lower specific surface area and consequently for the lower number of active sites for H2 production. Therefore, some attempts have been made by scientists to fine tune the band gap and enhance the rate of electron–hole pair separation so that the apparent quantum efficiency of the photocatalyst can be enhanced.
Noble metal nanoparticles such as Au and Ag are extensively studied owing to their characteristic localized surface plasmon resonance phenomenon15 (LSPR). By fine tuning the size and shape of metal nanoparticles,16 the SPR wavelength can be controlled from the visible to the near infrared region of the solar spectrum. The nanoparticles can competently harvest solar energy and widen the light absorption range so that photoinduced electrons can transfer to the semiconductor through so-called direct energy transfer17 or resonance energy transfer.18 The hot electrons19 generated due to LSPR excitation can be transferred into the neighboring adsorbent or return to the ground state after releasing excess energy to the surroundings. In the case of bimetal20 plasmonic alloy, an excited hot electron (having an energy of 1–3 eV, which is not in thermal equilibrium with the system) can migrate to the interface depending upon the electronegativity of the metal counterpart. Thus, the bimetal alloy is responsible for the storage of excited electrons, resulting in an increase in electron density that is subsequently useful for enhanced photocatalytic activity. Moreover, synthesis of bimetal alloys can provide a profitable path for the design of cost-effective photocatalysts, where the content of high-cost noble metal nanoparticles can be reduced. Due to the synergistic effect,21 there is a great improvement in their physical and chemical properties as compared to their monometallic constituents. The formation mechanism22,23 of alloy nanoparticles is simply the homogeneous mixing of two metals on the atomic scale and is differentiated by their metal to metal bonds. Density functional theory predicts that the synergistic effect24 of nanoalloys is due to the surface electronic state arising because of the effective atomic coordination number on the surface.25 Moreover, the construction of the core–shell nanostructure26 provides a unique platform for the loading of bimetal alloy onto a semiconductor surface.27 By fine tuning the core size and shell thickness,28 a number of core–shell nanoparticles can be explored with enhanced photocatalytic activity.29–33 Core–shell nanostructured photocatalysts are a unique class of nanomaterial possessing a core (inner material) and a shell (outer material) with nanoscale dimensions. The catalytic activity (both photocatalytic and electro-catalytic) depends on the synergistic interaction between the core and shell. Recently, various plasmonic nanoalloys have been demonstrated for photocatalytic processes, such as Au–Ag, Au–Cu, Au–Cu nanostars, and Pt–Au nano alloy. Nalluri et al. demonstrated the solvent-free synthesis of an Fe2O3@Au–Ag34 alloy core–shell nanostructured photocatalyst that showed excellent H2 generation compared to its monometallic Au counterparts. Wang et al. synthesized layered double hydroxide supported Au–Cu alloy nanoparticles,35 which show efficient esterification of benzyl alcohol with methanol. Liu et al. fabricated Au–Cu triangular nanostars on CdS36 by tuning the atomic ratio of Cu, which showed around threefold H2 generation compared to CdS. Au–Pt bimetal alloy37 was decorated on TiO2 nanorods via photo-deposition techniques, and its photocatalytic activity towards benzyl alcohol oxidation was explored. In order to explore the effect of the plasmonic phenomenon on the photocatalytic activity with varying amounts of nickel and silver, Ni and Ag co-modified g-C3N4 nanocomposites were fabricated using a photoreduction approach in a mixture of solvent media such as methanol and water, where the 3Ni–1Ag/CN sample produced 2137.5 μmol g−1 h−1 of H2.38 Spinel ZnFe2O4 microspheres coupled with plasmonic Ag/TiO2 nanorods were demonstrated to fabricate a Z-scheme heterojunction via a facile hydrothermal approach to explore the photocatalytic reduction of CO2 to CO.39 Plasmonic Cu-NPs were loaded with a 1D/2D composite of carbon nanotubes modified with protonated carbon nitrides (CNTs/pCN) for photocatalytic conversion of CO2 to CO.40 Similarly, plasmonic Au-NPs-embedded Z-scheme WO3/TiO2 hetero-junctions were fabricated for photocatalytic H2 evolution from a glycerol–water mixture, and 0.5% Au-loaded WO3/TiO2 produced 4.46-fold higher H2 evolution compared to WO3/TiO2.41 Further, various photocatalytic systems containing Ag–Cu bimetal alloy as their active plasmonic component are given in Table S1.†
This work demonstrates the fabrication of a series of SiO2@S-doped graphitic carbon nitride core–shell nanostructured photocatalysts in which Cu–Ag alloy was decorated between the junctions (Scheme 1). The physicochemical properties of the as-synthesized photocatalysts were well characterized using PXRD, HRTEM, FESEM, UVDRS, PL and photocurrent measurements. Further, DFT calculations were performed in order to determine the mechanism behind the water splitting reaction, and the role of Cu in Cu–Ag alloy towards H2 fuel generation has been explored. The as-synthesized photocatalysts were tested for photocatalytic H2 fuel generation. The synthesis procedure, characterization, photocatalytic activities and mechanistic insight are discussed in detail.
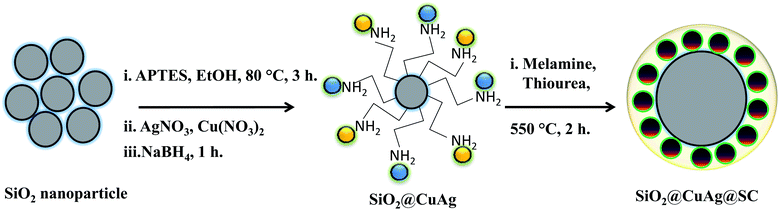 |
| Scheme 1 Synthesis of Ag–Cu bimetal alloy on the SiO2 surface and fabrication of few-layer SC wrapped over the bimetal alloy. | |
2. Experimental section
2.1 Reagents
Tetraethyl orthosilicate (TEOS), (3-aminopropyl)triethoxysilane (APTES), sodium borohydride (NaBH4), silver nitrate (AgNO3), copper nitrate hexahydrate (Cu(NO3)2·6H2O), melamine, thiourea, triethanolamine (TEOA), and sodium sulphate (Na2SO4) of analytical grade were obtained from Merck India and used as received. Double distilled water was used throughout the reaction.
2.2 Synthesis of SiO2 nanoparticle
SiO2 nanoparticles were prepared using a modified Stober's method described elsewhere.6 For amine functionalization, 2 g of the as-prepared SiO2 nanoparticles were dispersed in 100 mL isopropanol, to which 2 mL APTES was added. The resulting solution was refluxed for 2 h, centrifuged and dried at 80 °C overnight.
2.3 Synthesis of SiO2@AgCu nanoalloy
For the synthesis of SiO2@AgCu nanoalloy (Ag–Cu-1
:
3), 0.5 g of as-synthesized APTES-functionalized SiO2 were dispersed in 200 mL water. 20 mg of AgNO3 and 144 mg of Cu(NO3)2 were added to the above solution, and it was stirred for 15 min to achieve proper dispersion of metal ions. Thereafter, 5 mL of 0.1 M NaBH4 solution was added, which made the colorless solution acquire a light grayish color. The solution was aged for 2 h and then centrifuged to obtain the desired product. The material was dried in an oven overnight. In a similar way, other alloys, such as those with 1
:
1 and 3
:
1 ratios of Ag–Cu, as well as neat Ag and Cu were also loaded on SiO2.
2.4 Synthesis of SiO2@AgCu@S-doped C3N4
The as-obtained SiO2@AgCu (100 mg), 300 mg melamine and 300 mg thiourea were ground properly using a mortar and pestle. The as-obtained solid powder was calcined for 2 h at 550 °C in nitrogen atmosphere using a tubular furnace. The as-obtained material was labeled as SCSC-1
:
3. Other alloys containing 1
:
1 and 3
:
1 Ag to Cu ratios were also synthesized by following the same procedure and were labeled as SCSC-1
:
1 and SCSC-3
:
1 for SiO2@AgCu(1
:
1)@S-doped C3N4 and SiO2@AgCu(3
:
1)@S-doped C3N4, respectively. The synthesis protocol is described in Scheme 1. The monometallic counterparts containing only Ag and Cu were synthesized following the same procedure and are labeled SCS and SCC for SiO2@Ag@S-doped C3N4 and SiO2@Cu@S-doped C3N4, respectively. Neat S-doped g-C3N4 was fabricated on SiO2 without loading of the metallic component and was designated as SC.
2.5 Procedure for the photocatalytic reaction
The water splitting reaction was accomplished by dispersing 20 mg of each catalyst in 20 mL water containing 10 vol% triethanolamine (TEOA). The experiment was performed in a homemade quartz batch reactor of 100 mL capacity using a 150 W xenon lamp as the illumination source with a 420 nm cutoff filter. The photoreactor was thoroughly purged with N2 gas for half an hour prior to irradiation and stirred using a magnetic stirrer for the good dispersion of the catalyst. By adopting the downward displacement of water, the gas evolved from the reactor was collected and analyzed by a gas chromatograph using a thermal conductivity detector. The gas was identified to be H2.
2.6 Characterization
PXRD of the synthesized materials was performed using a Rigaku MiniFlex diffractometer fitted with Cu Kα radiation (l = 1.45 Å), scanned in the 2θ range from 10 to 80° with a rate of 2° min−1. An HRTEM (JEOL-JEM 2010, Japan) equipped with an energy dispersive X-ray spectrometer was used for the analysis of the morphologies and elemental compositions of the samples. A Krato Axis 165 instrument fitted with a Mg-Kα source was used for the measurement of the XPS spectra of the samples. UVDRS spectra and photoluminescence (PL) spectra were performed on a JASCO UV-Vis spectrophotometer and JASCO-FP-8300 spectrofluorometer in that order. All the electrochemical experiments were performed on an IVIUM-n-STAT instrument, where the electrochemical setup consisted of Pt and Ag/AgCl electrodes as the counter and reference electrode, respectively. For the preparation of the working electrode, the electrophoretic deposition technique was adopted, where 20 mg of every catalyst and iodine were spread in 20 mL acetone and sonicated for 15 min. Fluorine-doped tin oxide (FTO) was dipped in the dispersed medium, and 60 V bias was applied for 6 min for the fabrication of the catalyst on the FTO surface. Prior to conducting the electrochemical experiment, the FTOs were calcined at 200 °C for 2 h in N2 atmosphere. Linear sweep voltammetry (LSV) was performed by applying potentials from −0.6 to −1.5 V while adapting a scan rate of 25 mV s−1. The photocurrent measurements were performed using a 150 W xenon lamp.
2.7 Computational details
DFT calculations were carried out using the Gaussian 09 program42 with the B3LYP functional.43 The 6-31G* basis set44 was employed for all atoms except silver and copper, for which the effective core potential LanL2DZ basis set45,46 was used. Harmonic frequency calculations were performed for all stationary points to confirm them as local minima or transition states. Thermal correction and zero point vibration energies were incorporated in the ΔG values. The solvent effect of water was also taken into consideration by using the polarizable continuum model (PCM) for all the calculations.
3. Results and discussion
3.1 Crystal structure and morphology
Fig. 1(a) shows the typical PXRD pattern of the as-synthesized samples, where SCS shows peaks at 37.8, 43.8, 64.1, and 77.2° that were assigned to the (111), (200), (220), (311) planes of cubic silver (JCPDS-87-0717), respectively. In the case of SCC, peaks were observed at 43.1, 50.3, and 73.9° and were attributed to the (111), (200), and (220) planes of cubic copper (JCPDS-01-1241), in that order. When Cu–Ag nanoalloy was decorated on the SiO2 substrate and SC was warped on the alloy, the diffraction peaks shifted more towards the right upon silver incorporation, as found in the case of SCSC-3
:
1, whereas copper incorporation in the alloy shifted the 2θ value slightly towards the right. The peaks were shifted towards the right of the PXRD plot, suggesting the successful fabrication47 of Cu–Ag alloy on SiO2. The Ag (111) and Ag (220) planes were for fcc (Ag) cubic phase, with a lattice parameter a = 4.076 Å; moreover, the peak intensity of Ag (111) was found to be strongest, suggesting that the favorable facet for the Cu–Ag nanoalloy is (111). The broad peak at 2θ = 23.3° corresponds to the amorphous structure of SiO2.48,49 The crystallite sizes were calculated and found to be 47.7, 38.4, and 32.7 nm in the cases of SCSC-3
:
1, SCSC-1
:
1, and SCSC-1
:
3, respectively. In order to explore the morphology,50 high resolution transmission electron microscopy (HRTEM) was conducted. From Fig. 1(b), it can be confirmed that SiO2 nanoparticles around 120 nm in size were successfully synthesized, and few layers of SC with a thickness of around 3–5 nm were warped over it, forming a core–shell nanostructure. Fig. 1(c and d) shows the uniform distribution of the Ag–Cu nanoalloy on the SiO2 and SC nanojunction, and the particle size of the alloy is 1.3 nm. The homogeneous distribution of Ag–Cu nanoalloy on the SiO2 surface as well as the close contact between Ag–Cu and SC plays an important role in the enhancement of the photocatalytic activity of SCSC-1
:
3. Fig. 1(e) demonstrates that there was close contact between the SC and nanoalloy, favoring greater exciton separation efficiency. Again, (111) of Ag was the predominant face of the Cu–Ag alloy, and the lattice fringe was 0.23 nm, which is consistent with the PXRD data. The SAED pattern of SCSC-1
:
3 indicates ED spots of the (111) and (220) facets of Ag and (111) of Cu. The SAED data led us to conclude that the alloy particles were not amorphous but polycrystalline in nature (Fig. 1(f)). The weight percent of SCSC-1
:
3 was 2.7 (Ag
:
Cu-0.5
:
2.2), as depicted in the EDX spectra (Fig. S1a†). The eutectic temperature40 of the Ag–Cu alloy was 779.8 °C in N2 atmosphere, which confirms the thermal stability of the alloy in this work. The XRD and TEM results suggest the successful formation of the core–shell nanostructure, where Ag–Cu alloy was embedded inside the SiO2 and SC.
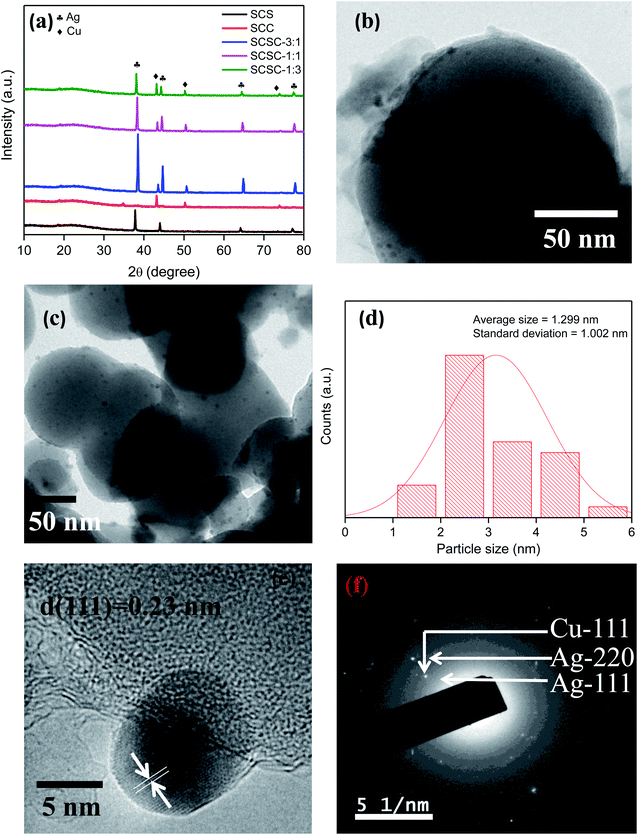 |
| Fig. 1 (a) PXRD of the as-synthesized core–shell nanostructured photocatalyst; (b and c) HRTEM images of SCSC-1 : 3, where SiO2 as the core and a few layers of SC act as the shell and Cu–Ag bimetal alloy is embedded between the SiO2 core and SC shell. (d–f) Particle size distribution of Ag–Cu alloy, lattice fringe width of SCSC-1 : 3 matching the d values of the bimetal alloy, and SAED pattern of SCSC-1 : 3. | |
3.2 Chemical state analysis
The XPS survey spectrum51 shows diverse peaks of Si, O, C, N, S, Ag and Cu related to the successful formation of the photocatalyst (Fig. S1b†). The peak at 284.5 eV was considered to be the reference peak for carbon, and peaks were assigned accordingly for the other elements. The XPS peaks of various elements were further deconvoluted using CASA XPS. The core level of C 1s consists of peaks at 284.5 and 287.4 eV, assigned as the adventitious carbon species by the instrument itself and carbon attached to the nitrogen center of carbon nitride, in that order (Fig. 2(a)). Three peaks for N 1s at 397.1, 398.1 and 399.6 eV were attributed to nitride or cyanide, nitrogen attached to the carbon center of carbon nitride, and nitrogen present in the organic matrix, respectively (Fig. S1c†). The core Si 2p has two peaks at 102.3, and 103.2 eV corresponds to the Si–O–Si linkage and Si–O bonding in SiO2. Similarly, two peaks are present at 531.1 and 532.6 eV for the O 1s core, related to the oxides of Cu and SiO2, respectively (Fig. 2(b and c)). The S 2p spectrum contains a single peak upon deconvolution at 162.6 eV, which corresponds to the C–S coordination present in the carbon nitride framework (Fig. 2(d)). In the case of the core level of Ag 3d, two peaks were present at 368.1 and 374.1 eV, related to the 3d5/2, and 3d3/2 spin states. The single peak at 3d5/2 corresponds to the Ag nanoparticles present in the alloy (Fig. 2(e)). Similarly, the XPS core spectrum of Cu 2p shows two peaks at 933.0 and 942.2 eV, which are ascribed to Cu 2p3/2 and Cu 2p1/2. The peak present at 942.2 eV is the satellite peak.52 Cu 2p3/2 upon deconvolution gives two peaks at 933.0 and 935.3 eV, related to the metallic and +2 oxidation states of Cu, respectively (Fig. 2(f)). The atomic weight percent of Ag–Cu was 1.8% (Ag
:
Cu-0.45
:
1.03). A greater amount of Cu reduction is possible in the Ag–Cu bimetal alloy compared to the single monometallic Cu nanoparticles due to the alloying effect, as reported in other systems such as ZrO2.53
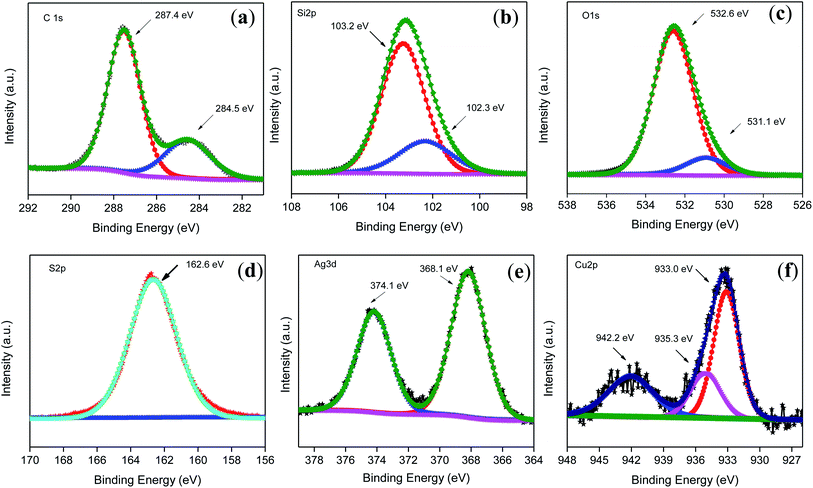 |
| Fig. 2 XPS spectra of SCSC-1 : 3: (a) C 1s, (b) Si 2p, (c) O 1s, (d) S 2p, (e) Ag 3d, (f) Cu 2p. | |
3.3 Photophysical properties
The optical response of the as-synthesized photocatalysts is portrayed in Fig. 3(a and b). The absorption maxima for SCS and SCC have shifted to the more visible region of the solar spectrum as compared to the bare SC, with a band gap of 2.64 eV. The shifting in the UV-Vis peak is due to the surface plasmon resonance phenomenon (SPR) of Ag and Cu.54,55 Similarly, in the case of the alloy nanostructures SCSC-3
:
1, SCSC-1
:
1 and SCSC-1
:
3, the absorption peaks were shifted to the more visible region of the solar spectrum, i.e. the absorption band edges for SCS, SCC, SCSC-3
:
1 and SCSC-1
:
1 were 489.0, 567.2, 604.5, and 690.0 nm, respectively, and SCSC-1
:
3 has more visible light absorption, covering the absorption of all the photocatalysts. This represents the full spectrum solar light absorption from UV to Vis to NIR by SCSC-1
:
3, which relates to its excellent catalytic activity. The broad peak in the case of the STSCs is evidence for the formation of a bimetal alloy and not a core–shell structure between Ag and Cu, as the SPR peaks did not appear distinctly for Ag and Cu. The greater photocatalytic activity by the SCSC-1
:
3 catalysts is due to their high visible light absorption, simultaneous alloying effect and surface plasmon resonance phenomenon. Photoluminescence spectroscopy is an important tool55,56 in order to probe the photo-induced electron–hole-pair generation and separation at the interface of a photocatalyst. Photoluminescence spectroscopy was performed for all photocatalysts at an excitation wavelength of 330 nm and portrayed in Fig. 3(b). The spectra showed a broad peak at 450 nm in the case of SC, which relates to the band to band transitions. The PL intensity decreased in the order of SC > SCS > SCC > SCSC-3
:
1 > SCSC-1
:
1 > SCSC-1
:
3, as shown in Fig. S1d.† The trends in the PL intensity suggest that the intensity decreases upon an increase in the amount of Cu. The lesser the PL intensity, the greater the electron–hole pair separation and the better the activity of the photocatalyst. The photo-physical properties, including UVDRS and photoluminescence spectroscopy, suggest that SCSC-1
:
3 is the best photocatalyst among all.
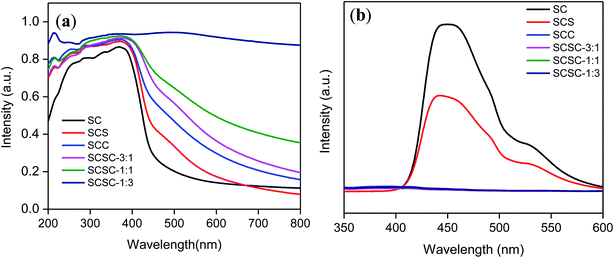 |
| Fig. 3 (a) UV-Vis spectra, (b) photoluminescence spectra of the as-synthesized photocatalysts. | |
3.4 DFT calculations
To gain further insight into the mechanism towards photocatalytic H2 generation, DFT calculations were carried out for the best proposed photocatalyst using Ag1Cu3 as a model. A rhombic Ag1Cu3 model is predicted, with a ground state spin multiplicity of 1 (Table S2†). All attempts to optimize a tetrahedral ground state of Ag1Cu3 failed and converged to a rhombic geometry. As the H2 evolution catalytic process occurs in water medium, the ability of the Ag1Cu3 catalyst to bind with H+/H as well as water was evaluated alongside natural bond orbital (NBO) analysis (Fig. S2†). From Fig. S2,† it is clear that Cu2 and Cu3 are structurally and electronically similar. Both are potentially the best sites for water and H+/H binding. However, H+/H binding is thermodynamically much more favorable compared to water binding, according to the binding energy values. The highest negative charge density on Cu1 atom, as per the NBO analysis, may be the reason for its inability to bind with water and H. Considering that the reaction is occurring in water medium, the possibility of water splitting along with the H2 evolution mechanism has been investigated. The optimized model of water bound to the Cu3 atom of Ag1Cu3 is shown in Fig. 4(a) (left). Here, the water molecule can go on to interact with the neighbor atoms Ag4 as well as Cu1 via a 4-membered transition state. Both of the possible transition states are shown in Fig. 4(a) (right), and it was found that the kinetic barrier for water to interact with the Cu1 atom is 2 kcal mol−1 lower than that of Ag4 atom. Hence, the final product of water split into OH− and H+ will proceed through the interaction with Cu1 atom via a 4-membered transition state, yielding a tetrahedral geometry where OH− is bound to Cu3 and H+ is bound to Cu1.
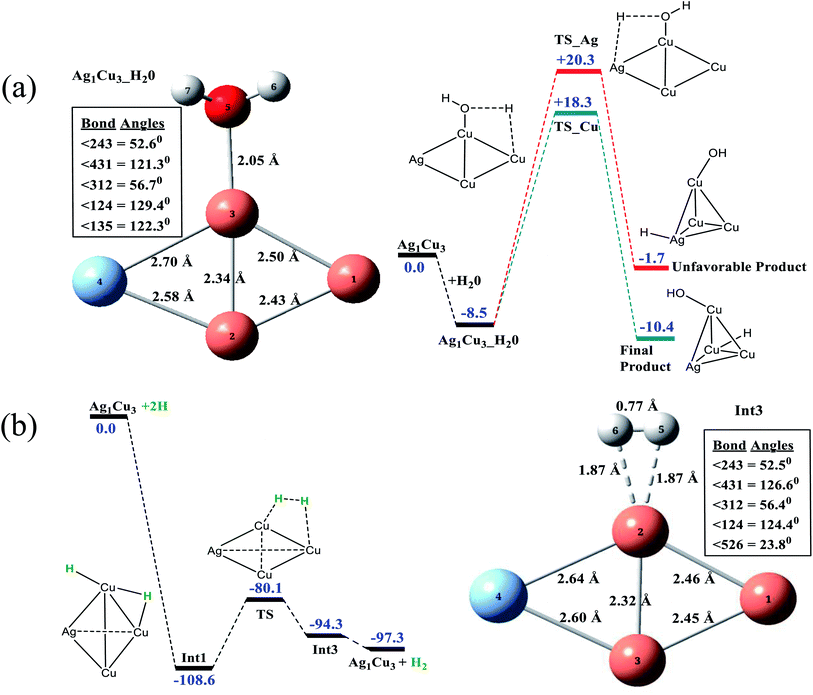 |
| Fig. 4 (a) Left: optimized model of water bound to Cu3 of Ag1Cu3 with the bond parameters. Right: free energy pathway showing water splitting with relative ΔG values (in kcal mol−1). (b) Right: optimized model of Int3 (H2 bound to Cu2 of Ag1Cu3) with the bond parameters, left: free energy pathway showing H2 evolution with relative ΔG values (in kcal mol−1). | |
The H2 evolution mechanism for the Pt–Sn system was reported by Chaokong et al. We found similar pathways for the Ag1Cu3 system (Fig. 4(b)). Two H+ are adsorbed on the Cu2 atom of Ag1Cu3 and reduced to form Int1 (intermediate-1), which then undergoes a 4-membered transition state with a barrier of 28.5 kcal mol−1 to break the interaction between H–Cu1 and result in the formation of Int3 (intermediate-3), as shown in Fig. 4(b) (right). The release of loosely bound H2 from Int3 is found to be thermodynamically favorable by 3 kcal mol−1.
3.5 Photoelectrochemistry
Taking a classical three-electrode system with 0.1 M Na2SO4 solution as the electrolyte, photoelectron chemistry of all the synthesized materials was performed. The linear sweep voltammetry (LSV) plot (Fig. 5(a)) suggests that SCSC-1
:
3 generated 12 mA cm−2 current in the cathodic direction. Similarly, other photocatalysts, such as SC, SCS, SCC, SCSC-3
:
1, and SCSC-1
:
1, show 0.04, 1.9, 2.4, 5.7, and 6.3 mA cm−2 current in the cathodic direction upon irradiation, respectively. Moreover, the catalyst SCSC-1
:
3 showed current at an onset potential of −1.00 V as compared to the other photocatalysts, which showed current at potentials of −1.45, −1.43, −1.07 and −1.05 for SCS, SCC, SCSC-3
:
1, and SCSC-1
:
1, in that order. The excellent photocurrent generation in the case of SCSC-1
:
3 is attributed to the smaller particle size of bimetal alloy, on the order of 1.3 nm. Gratzel has shown that the particle size is the most important parameter for the photo-electrochemical properties of a semiconductor. A space charge region is not formed when the particle size becomes very small, leading to no exciton separation in the semiconductor. In this case, the photocurrent generation is due to the mobility of positive and negative charge carriers. Due to the small size of Ag–Cu, the applied potential was reasonably low for the enhancement in the efficiency of the charge carrier transfer process at the electrode/electrolyte interface. Again, the coating of SC on SiO2 can act as a channelizer for generation of hot electrons by Ag–Cu and subsequent production of hot holes bellow the Fermi level of Ag–Cu. These hot electrons and holes have sufficient energy to surpass the Schottky barrier, as a result of which Cu–Ag/SC nanojunctions can generate more photocurrent in the cathodic direction. The excellent current generation at lower overpotential by SCSC-1
:
3 relates to its best photocatalytic activity towards H2 fuel generation.
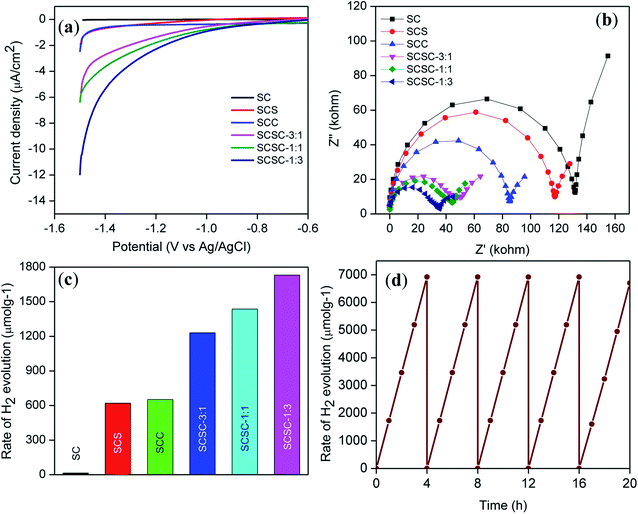 |
| Fig. 5 (a) Linear sweep voltammetry and (b) electrochemical impedance spectra of the as-synthesized photocatalysts. (c) H2 generation values for all the photocatalysts. (d) Stability test for photocatalytic H2 evolution. | |
Electrochemical impedance spectroscopy57 is a very important tool to explore the charge transfer resistance and ease of electron flow in the semiconductor electrolyte interface as well as the mechanism of the electrochemical reaction. 0.1 M Na2SO4 solution was used as an electrolyte and impedance spectroscopy was performed at an applied potential of 0.0 V. The Nyquist plot contains the imaginary part Z′ and real part Z′′ as the abscissa and ordinate,5 which relate to the resistance and reactance of the semiconductor,58 respectively. Fig. 5(b) describes the Nyquist plots of all the photocatalysts, and their values were found to be 131.9, 117.5, 85.0, 47.4, 44.2 and 35.5 Ω for SC, SCS, SCC, SCSC-3
:
1, SCSC-1
:
1 and SCSC-1
:
3, in that order. The larger arc in the case of SC relates to the high charge transfer resistance, which leads to poor separation of photo-generated electron–hole pairs. The smaller arc in the case of SCSC-1
:
3 relates to the lower charge transfer resistance and higher electrical conductivity. The higher electrical conductivity of the photocatalyst can be corroborated from the LSV data. The decrease in the charge transfer resistance and increase in the electrical conductivity are attributed to the allocation of hot electrons from the Ag–Cu bimetal alloy to SC, thereby inhibiting charge carrier recombination; in all probability, this subsidizes the excellent improvement in the activity. The CB potential of SC55 has been calculated from the Mott–Schottky plot, and the corresponding VB potential was calculated using the band gap of SC.
3.6 Photocatalytic activity
The catalytic activities of the photocatalysts were explored for the production of H2 through the water splitting reaction and are depicted in Fig. 5(c). The procedure follows the dispersion of each catalyst in 20 mL of water containing TEOA in a sealed quartz batch reactor. The photocatalysts SC, SCS, SCC, SCSC-3
:
1, SCSC-1
:
1 and SCSC-1
:
3 were able to generate H2 amounts of 15.2, 620, 652, 1230, 1436, and 1730 μmol h−1 g−1, in that order. It can be deduced from the H2 generation figures that SCSC-1
:
3 can more effectively produce H2 compared to the other catalysts. Quenching experiments were explored in order to identify the involvement of active species (Fig. S3†). The experiments were performed in the presence of AgNO3, isopropyl alcohol, TEOA and p-benzoquinone as scavengers for electrons, hydroxyl radical, holes and superoxide radical, respectively. The results suggest that electrons are the main active species for the reduction of protons to H2, and the catalyst was also stable up to 4 runs (Fig. 5(d)). The apparent quantum yield was estimated to be 5.2% (band pass filter > 420 nm, 0.3 W irradiatometer). The formula for the apparent quantum yield calculation is
The excellent photocatalytic activities of SCSC-1
:
3 were due to the succeeding evidence. From the UV-Vis and PL spectra, it is clear that the photocatalyst can absorb more visible light and exhibit a lower electron–hole recombination rate, respectively. The generation of the high photocurrent value is due to the production of hot electrons through the LSPR process. The interfacial charge transfer process is very high due to the close contact between the Ag–Cu and SC in the case of SCSC-1
:
3, as portrayed from the Nyquist plot. Further, the reusability test of the catalyst towards the water splitting reaction proves the stability of the material, as suggested by PXRD and HRTEM (Fig. S4a–c†).
The size of the plasmonic nanoparticles plays a vital role in the plasmonic performance, and this holds especially true for low band gap semiconductors, where interband excitations occur in wavelength regions which generally overlap with plasmonic resonances. BiVO4 films were decorated with AuNPs of varying size from 10 to 80 nm to study the size dependence of the plasmonic effect.59 For the excellent visible light photocatalytic activity of Au/TiO2, it was shown that the gold loading and particle size of the gold nanoparticles play important roles in the enhancement of the hydrogen evolution activity with a catalyst containing 0.2 wt% gold with 1.87 nm average particle size.60 Generally, the plasmonic phenomenon and subsequent ejection of hot electrons occur upon irradiation. In this case, a single semiconductor (S-doped C3N4) is present; therefore, a Schottky junction is established between the alloy and semiconductor. The CB/VB of the semiconductor remains same in the dark as well as upon irradiation. The point is more significant when two different semiconductors (the alloy is not a semiconductor here) are present. Hence, we have shown the pinning of hot electrons upon light irradiation for the nanoalloy as well as silver and copper nanoparticles. We have focused only on the proton reduction reaction, whose reduction potential also remains intact before and after irradiation. On the basis of the high photocatalytic activity of the catalyst, a plausible mechanism can be expected and is described in Scheme 2. The mechanism is explained for the individual cases of Ag and Cu and related to the Ag–Cu alloy case. In the SCS system, the Schottky barrier height is estimated to be 0.7 eV, calculated by the difference between the work function of Ag61 (ϕ = 4.0 eV) and the electronegativity of the conduction band of SC (χ = 4.7 eV), which is high and subsequently leads to lower photocatalytic activity of SCS (Scheme S1†). In the case of SCC, the Schottky barrier62 was found to be 0.2 eV (work function of Cu, ϕ = 4.5 eV), which demonstrates the higher photocatalytic activity of the material by pinning hot electrons via LSPR. The excellent photocatalytic activity of SCSC-1
:
3 is explained by the appropriate height of the Schottky barrier63 (Scheme 2). The work function64 of the bimetal alloy lies between the work functions of Ag and Cu, which facilitates better electron–hole pair separation by encouraging smooth transfer of hot electrons from the alloy to SC. The VB and CB potentials for SC were calculated according to the equation EVB = X − Ee + 0.5Eg, ECB = EVB − Eg, where X is the geometric mean of the electronegativity of the constituent atoms, Ee is the energy of a free electron (4.5 eV), and Eg is the band gap energy. The Schottky barrier height was estimated by the difference in the work function of plasmonic metal and the electronegativity of the conduction band of SC, and the mechanism for the photocatalytic activity followed similarly, as described in the literature.65 Again, the electronegativity of Cu is greater than that of Ag, which leads to charge separation in the alloy; subsequently, electron donation takes place from Ag to Cu. Further, DFT studies revealed the role of Cu in Ag3Cu9, which plays an important role in the generation of H2 fuel from water. The decrease in the photocatalytic activities in the case of SCSC-3
:
1 is due to the presence of a higher amount of Ag. As described in the mechanism, the greater amount of Ag in the bimetal alloy is responsible for the enhancement of the Schottky barrier height and subsequently makes the electron shuttling process difficult. This is due to incomplete mixing of the metal components, which leads to improper band alignment. The photocatalytic activity data suggested that an alloy containing proper amounts of Ag and Cu that are mixed homogeneously can tune the H2 evolution to a peak as compared to the other synthesized alloys in this work. The molecular mechanism is as follows.
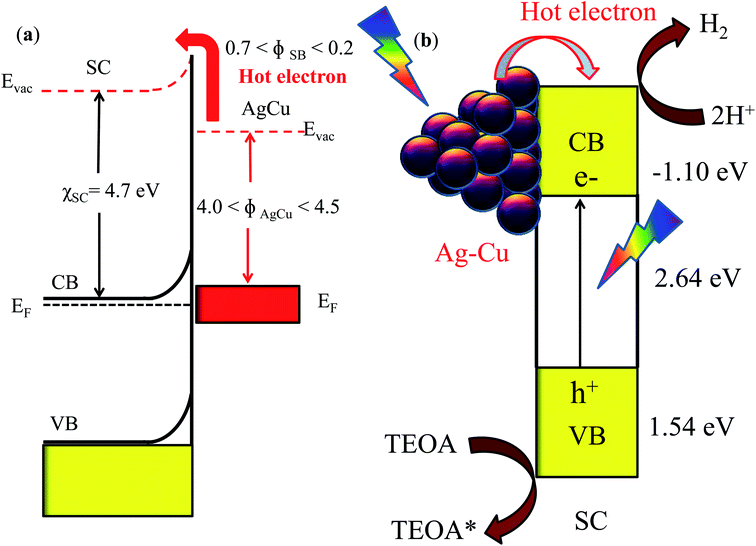 |
| Scheme 2 (a) Energy band diagram of SCSC-1 : 3, where EF, Evac, χSC, CB, VB, ϕSB, and ϕAgCu represent the Fermi energy level, vacuum energy level, electronegativity of the conduction band of SC, conduction band and valence band of SC, Schottky barrier height, and work function of Ag–Cu nanoalloy, respectively (b) hot electron generation and their participation in proton reduction to produce H2 fuel. | |
4. Conclusion
In conclusion, a few layers of SC were warped on the surface of SiO2 to embed Ag–Cu bimetal alloys (with varying Ag to Cu ratios) between them to form a core–shell nanostructure. Out of the various synthesized photocatalysts, SCSC-1
:
3, showed excellent photocatalytic activity towards H2 fuel (1730 μmol h−1 g−1) upon illumination. SCSC-1
:
3 showed excellent visible light absorption due to the synergistic effect of the alloy and plasmonic phenomena. The lowest intensity in the PL spectrum and lowest arc in the Nyquist plot confirmed the better electron–hole pair charge separation and higher electrical conductivity for SCSC-1
:
3, respectively. LSV measurements suggest that SCSC-1
:
3 can produce 6.2 times more photocurrent in the cathodic direction compared to SCS. Moreover, DFT studies proposed that the Cu atom of Ag1Cu3 plays the pivotal role in the generation of H2, and the reaction proceeds via a 4-membered transition state. The excellent activity was accredited to the compact nanojunction between Ag–Cu and SC, where the hot electrons could easily channelize to SC, lowering electron–hole pair recombination. This work provides a suitable demonstration of the design of cost-effective plasmonic bimetallic alloys and their activities towards energy generation.
Author contribution
PB has carried out synthesis, characterization and activity of the photocatalyst and written the manuscript. SRD has performed the DFT calculation. AB has provided assistance during the synthesis. TV and AA have carried out HRTEM analysis. KMP provides overall guidance.
Conflicts of interest
The authors declare no competing financial interest.
Acknowledgements
All the authors are very much thankful to Siksha ‘O’ Anusandhan, Bhubaneswar for infrastructure. SRD acknowledges the Centre of Excellence in Scientific Computing (COESC), CSIR-NCL, Pune for providing computational facilities.
References
- M. R. Hoffman, S. T. Martin, W. Choi and D. W. Bahnemann, Photocatalysis over semiconductors, Chem. Rev., 1995, 95, 69, DOI:10.1021/cr00033a004.
- X. Chen, S. Shen, L. Guo and S. S. Mao, Semiconductor-based photocatalytic hydrogen generation, Chem. Rev., 2010, 110(11), 6503–6570, DOI:10.1021/cr1001645.
- B. O'regan and M. Grätzel, A low-cost, high-efficiency solar cell based on dye-sensitized colloidal TiO2 films, Nature, 1991, 353(6346), 737–740, DOI:10.1038/353737a0.
- B. Naik, K. M. Parida and C. S. Gopinath, Facile synthesis of N-and S-incorporated nanocrystalline TiO2 and direct solar-light-driven photocatalytic activity, J. Phys. Chem. C, 2010, 114(45), 19473–19482, DOI:10.1021/jp1083345.
- P. Babu, S. Mohanty, B. Naik and K. Parida, Serendipitous assembly of mixed phase BiVO4 on B-doped g-C3N4: an appropriate p–n heterojunction for photocatalytic O2 evolution and Cr (VI) reduction, Inorg. Chem., 2019, 58(18), 12480–12491, DOI:10.1021/acs.inorgchem.9b02309.
- S. Mohanty, P. Babu, K. Parida and B. Naik, Surface-plasmon-resonance-induced photocatalysis by core–shell SiO2@AgNCs@Ag3PO4 toward water-splitting and phenol oxidation reactions, Inorg. Chem., 2019, 58(15), 9643–9654, DOI:10.1021/acs.inorgchem.9b00218.
- X. Wang, K. Maeda, A. Thomas, K. Takanabe, G. Xin, J. M. Carlsson, K. Domen and M. Antonietti, A metal-free polymeric photocatalyst for hydrogen production from water under visible light, Nat. Mater., 2009, 8(1), 76–80, DOI:10.1038/nmat2317.
- Y. Wang, X. Wang and M. Antonietti, Polymeric graphitic carbon nitride as a heterogeneous organocatalyst: from photochemistry to multipurpose catalysis to sustainable chemistry, Angew. Chem., 2012, 51(1), 68–89, DOI:10.1002/anie.201101182.
- J. Huang, J. Du, H. Du, G. Xu and Y. Yuan, Control of Nitrogen Vacancy in g-C3N4 by Heat Treatment in an Ammonia Atmosphere for Enhanced Photocatalytic Hydrogen Generation, Acta Phys.-Chim. Sin., 2020, 36(7), 1905056 Search PubMed.
- H. Xu, R. Xiao, J. Huang, Y. Jiang, C. Zhao and X. Yang, In situ construction of protonated g-C3N4/Ti3C2 MXene Schottky heterojunctions for efficient photocatalytic hydrogen production, Chin. J. Catal., 2021, 42(1), 107–114 CrossRef CAS.
- L. Xinyong, J. Xie, S. Liu, A. Adamski, X. Chen and X. Li, Low-cost Ni3B/Ni(OH)2 as an ecofriendly hybrid cocatalyst for remarkably boosting photocatalytic H2 production over g-C3N4 nanosheets, ACS Sustainable Chem. Eng., 2018, 6(10), 13140–13150 CrossRef.
- W. Liang, C. Zhu, L. Yin and W. Huang, Construction of Pt-M (M= Co, Ni, Fe)/g-C3N4 composites for highly efficient photocatalytic H2 generation, Acta Phys.-Chim. Sin., 2020, 36, 1907001, DOI:10.3866/pku.whxb201907001.
- S. Rongchen, W. Liu, D. Ren, J. Xie and X. Li, Co1.4Ni0.6P cocatalysts modified metallic carbon black/g-C3N4 nanosheet Schottky heterojunctions for active and durable photocatalytic H2 production, Appl. Surf. Sci., 2019, 466, 393–400 CrossRef.
- S. Rongchen, K. He, A. Zhang, N. Li, Y. Hau Ng, P. Zhang, J. Hu and X. Li, In-situ construction of metallic Ni3C@Ni core–shell cocatalysts over g-C3N4 nanosheets for shell-thickness-dependent photocatalytic H2 production, Appl. Catal., B, 2021, 291, 120104 CrossRef.
- S. Cao, J. Low, J. Yu and M. Jaroniec, Polymeric photocatalysts based on graphitic carbon nitride, Adv. Mater., 2015, 27(13), 2150–2176, DOI:10.1002/adma.201500033.
- C. Clavero, Plasmon-induced hot-electron generation
at nanoparticle/metal-oxide interfaces for photovoltaic and photocatalytic devices, Nat. Photonics, 2014, 8(2), 95–103, DOI:10.1038/nphoton.2013.238.
- S. E. Lohse and C. J. Murphy, The quest for shape control: a history of gold nanorod synthesis, Chem. Mater., 2013, 25(8), 1250–1261 CrossRef CAS.
- S. K. Cushing, J. Li, F. Meng, T. R. Senty, S. Suri, M. Zhi, M. Li, A. D. Bristow and N. Wu, Photocatalytic activity enhanced by plasmonic resonant energy transfer from metal to semiconductor, J. Am. Chem. Soc., 2012, 134(36), 15033–15041 CrossRef CAS.
- J. Li, S. K. Cushing, F. Meng, T. R. Senty, A. D. Bristow and N. Wu, Plasmon-induced resonance energy transfer for solar energy conversion, Nat. Photonics, 2015, 9(9), 601–607 CrossRef CAS.
- Y. K. Lee, C. H. Jung, J. Park, H. Seo, G. A. Somorjai and J. Y. Park, Surface plasmon-driven hot electron flow probed with metal-semiconductor nanodiodes, Nano Lett., 2011, 11(10), 4251–4255, DOI:10.1021/nl2022459.
- J. Y. Park, Y. Zhang, M. Grass, T. Zhang and G. A. Somorjai, Tuning of catalytic CO oxidation by changing composition of Rh−Pt bimetallic nanoparticles, Nano Lett., 2008, 8(2), 673–677, DOI:10.1021/nl073195i.
- I. Mondal, S. Y. Moon, H. Lee, H. Kim and J. Y. Park, Two-dimensional FeS2-encapsulated Au: A quasi-epitaxial heterojunction for synergistic catalytic activity under photoelectrocatalytic water reduction, J. Mater. Chem. A, 2019, 7(33), 19258–19268, 10.1039/C9TA02065A.
- A. K. Singh and Q. Xu, Synergistic catalysis over bimetallic alloy nanoparticles, ChemCatChem, 2013, 5(3), 652–676, DOI:10.1002/cctc.201200591.
- B. Tudu, N. Nalajala, K. Reddy, P. Saikia and C. S. Gopinath, Electronic integration and thin film aspects of Au–Pd/rGO/TiO2 for improved solar hydrogen generation, ACS Appl. Mater. Interfaces, 2019, 11(36), 32869–32878, DOI:10.1021/acsami.9b07070.
- R. Majee, A. Kumar, T. Das, S. Chakraborty and S. Bhattacharyya, Tweaking nickel with minimal silver in a heterogeneous alloy of decahedral geometry to deliver platinum-like hydrogen evolution activity, Angew. Chem., 2019, 59(7), 2881–2889, DOI:10.1002/anie.201913704.
- K. Laasonen, E. Panizon, D. Bochicchio and R. Ferrando, Competition between icosahedral motifs in AgCu, AgNi, and AgCo nanoalloys: a combined atomistic–DFT study, J. Phys. Chem. C, 2013, 117(49), 26405–26413, DOI:10.1021/jp410379u.
- S. H. Bae, J. E. Kim, H. Randriamahazaka, S. Y. Moon, J. Y. Park and I. K. Oh, Seamlessly conductive 3D nanoarchitecture of core–shell Ni-Co nanowire network for highly efficient oxygen evolution, Adv. Energy Mater., 2017, 7(1), 1601492, DOI:10.1002/aenm.201601492.
- S. W. Lee, J. W. Hong, H. Lee, D. H. Wi, S. M. Kim, S. W. Han and J. Y. Park, The surface plasmon-induced hot carrier effect on the catalytic activity of CO oxidation on a Cu2O/hexoctahedral Au inverse catalyst, Nanoscale, 2018, 10(23), 10835–10843, 10.1039/C8NR00555A.
- M. B. Gawande, A. Goswami, T. Asefa, H. Guo, A. V. Biradar, D. L. Peng, R. Zboril and R. S. Varma, Core–shell nanoparticles: synthesis and applications in catalysis and electrocatalysis, Chem. Soc. Rev., 2015, 44(21), 7540–7590, 10.1039/C5CS00343A.
- H. Lee, J. Lim, C. Lee, S. Back, K. An, J. W. Shin, R. Ryoo, Y. Jung and J. Y. Park, Boosting hot electron flux and catalytic activity at metal–oxide interfaces of PtCo bimetallic nanoparticles, Nat. Commun., 2018, 9(1), 1–8, DOI:10.1038/s41467-018-04713-8.
- B. Naik, S. M. Kim, C. H. Jung, S. Y. Moon, S. H. Kim and J. Y. Park, Enhanced H2 generation of Au-loaded, nitrogen-doped TiO2 hierarchical nanostructures under visible light, Adv. Mater. Interfaces, 2014, 1(1), 1300018, DOI:10.1002/admi.201300018.
- M. Kumar, K. Soni, B. Satpati, C. S. Gopinath and S. Deka, Exploration of magnetically separable Ag@AgxNiy core/graded-alloy-shell nanostructures, Chem. Commun., 2016, 52(56), 8737–8740, 10.1039/C6CC04087J.
- A. B. Vysakh, K. J. Shebin, R. Jain, P. Sumanta, C. S. Gopinath and C. Vinod, Surfactant free synthesis of Au@Ni core-shell nanochains in aqueous medium as efficient transfer hydrogenation catalyst, Appl. Catal., A, 2019, 575, 93–100, DOI:10.1016/j.apcata.2019.01.017.
- S. R. Nalluri, R. Nagarjuna, D. Patra, R. Ganesan and G. Balaji, Large scale solid-state synthesis of catalytically active Fe3O4@M (M= Au, Ag and Au-Ag alloy) core-shell nanostructures, Sci. Rep., 2019, 9(1), 1–11, DOI:10.1038/s41598-019-43116-7.
- X. Wang, R. Wang, J. Wang, C. Fan and Z. Zheng, The synergistic role of the support surface and Au–Cu alloys in a plasmonic Au–Cu@LDH photocatalyst for the oxidative esterification of benzyl alcohol with methanol, Phys. Chem. Chem. Phys., 2020, 22(3), 1655–1664, 10.1039/C9CP05992J.
- Q. Y. Liu, Y. Zhong, Z. Z. Jiang, K. Chen, S. Ma, F. Wang, W. Wang, L. Zhou and Q. Wang, Controlled growth of triangular AuCu alloy nanostars and high photocatalytic activities of AuCu@CdSheterostars, J. Mater. Chem. C, 2020, 8(14), 4869–4875, 10.1039/D0TC00098A.
- X. F. Zhang, Z. Wang, Y. Zhong, J. Qiu, X. Zhang, Y. Gao, X. Gu and J. Yao, TiO2 nanorods loaded with AuPt alloy nanoparticles for the photocatalytic oxidation of benzyl alcohol, J. Phys. Chem. Solids, 2019, 126, 27–32, DOI:10.1016/j.jpcs.2018.10.026.
- S. Fahad, M. Tahir and H. Alias, Synergistic effect of photo-reduced Ni-Ag loaded g-C3N4 nanosheets for efficient visible Light Driven photocatalytic hydrogen evolution, Mater. Sci. Semicond. Process., 2022, 137, 106187 CrossRef.
- T. Muhammad, Well-designed ZnFe2O4/Ag/TiO2 nanorods heterojunction with Ag as electron mediator for photocatalytic CO2 reduction to fuels under UV/visible light, J. CO2 Util., 2020, 37, 134–146 CrossRef.
- T. Muhammad, B. Tahir, M. G. M. Nawawi, M. Hussain and A. Muhammad, Cu-NPs embedded 1D/2DCNTs/pCN heterojunction composite towards enhanced and continuous photocatalytic CO2 reduction to fuels, Appl. Surf. Sci., 2019, 485, 450–461 CrossRef.
- T. Muhammad, M. Siraj, B. Tahir, M. Umer, H. Alias and N. Othman, Au-NPs embedded Z–scheme WO3/TiO2 nanocomposite for plasmon-assisted photocatalytic glycerol-water reforming towards enhanced H2 evolution, Appl. Surf. Sci., 2020, 503, 144344 CrossRef.
-
M. J. T. Frisch, G. W. Trucks, H. B. Schlegel, G. E. Scuseria, M. A. Robb, J. R. Cheeseman, G. Scalmani, V. Barone, B. Mennucci, G. A. Petersson, H. Nakatsuji, M. Caricato, X. Li, H. P. Hratchian, A. F. Izmaylov, J. Bloino, G. Zheng, J. L. Sonnenberg, M. Hada, M. Ehara, K. Toyota, R. Fukuda, J. Hasegawa, M. Ishida, T. Nakajima, Y. Honda, O. Kitao, H. Nakai, T. Vreven, J. A. Montgomery Jr, J. E. Peralta, F. Ogliaro, M. Bearpark, J. J. Heyd, E. Brothers, K. N. Kudin, V. N. Staroverov, R. Kobayashi, J. Normand, K. Raghavachari, A. Rendell, J. C. Burant, S. S. Iyengar, J. Tomasi, M. Cossi, N. Rega, J. M. Millam, M. Klene, J. E. Knox, J. B. Cross, V. Bakken, C. Adamo, J. Jaramillo, R. Gomperts, R. E. Stratmann, O. Yazyev, A. J. Austin, R. Cammi, C. Pomelli, J. W. Ochterski, R. L. Martin, K. Morokuma, V. G. Zakrzewski, G. A. Voth, P. Salvador, J. J. Dannenberg, S. Dapprich, A. D. Daniels, Ö. Farkas, J. B. Foresman, J. V. Ortiz, J. Cioslowski and D. J. Fox, Gaussian 09, Revision A.1, Gaussian, Inc., Wallingford CT, 2009 CrossRef CAS; A. D. Becke, A half-half theory of density functionals, J. Chem. Phys., 1993, 98, 1372 CrossRef CAS; C. Lee, W. Yang and R. G. Parr, Development of the Colle-Salvetti correlation-energy formula into a functional of the electron density, Phys. Rev. B: Condens. Matter Mater. Phys., 1988, 37(2), 785 CrossRef.
-
(a) P. C. Hariharan and J. A. Pople, The influence of polarization functions on molecular orbital hydrogenation energies, Theor. Chim. Acta, 1973, 28(3), 213–222 CrossRef CAS;
(b) R. H. W. J. Ditchfield, W. J. Hehre and J. A. Pople, Self-consistent molecular-orbital methods. IX. An extended Gaussian-type basis for molecular-orbital studies of organic molecules, J. Chem. Phys., 1971, 54(2), 724–728 CrossRef CAS.
- D. Andrae, U. Haeussermann, M. Dolg, H. Stoll and H. Preuss, Energy-adjusted ab initio pseudopotentials for the second and third row transition elements, Theor. Chim. Acta, 1990, 77(2), 123–141 CrossRef CAS.
-
(a) P. J. Hay and W. R. Wadt, Ab initio effective core potentials for molecular calculations. Potentials for the transition metal atoms Sc to Hg, J. Chem. Phys., 1985, 82(1), 270–283 CrossRef CAS;
(b) W. R. Wadt and P. J. Hay, Ab initio effective core potentials for molecular calculations. Potentials for main group elements Na to Bi, J. Chem. Phys., 1985, 82(1), 284–298 CrossRef CAS;
(c) W. R. Wadt and P. J. Hay, J. Chem. Phys., 1985, 82(1), 284 CrossRef CAS.
-
(a) J. Tomasi and M. Persico, Molecular interactions in solution: an overview of methods based on continuous distributions of the solvent, Chem. Rev., 1994, 94(7), 2027–2094 CrossRef CAS;
(b) M. Cossi, G. Scalmani, N. Rega and V. Barone, New developments in the polarizable continuum model for quantum mechanical and classical calculations on molecules in solution, J. Chem. Phys., 2002, 117(1), 43–54 CrossRef CAS;
(c) J. Tomasi, B. Mennucci and R. Cammi, Quantum mechanical continuum solvation models, Chem. Rev., 2005, 105(8), 2999–3094 CrossRef CAS.
- M. K. Singh, P. Manda, A. K. Singh and R. K. Mandal, Localized surface plasmon behavior of Ag-Cu alloy nanoparticles stabilized by rice-starch and gelatin, AIP Adv., 2015, 5(10), 107108, DOI:10.1063/1.4933072.
- Y. Liang, J. Ouyang, H. Wang, W. Wang, P. Chui and K. Sun, Synthesis and characterization of core–shell structured SiO2@YVO4: Yb3+, Er3+ microspheres, Appl. Surf. Sci., 2012, 258(8), 3689–3694, DOI:10.1016/j.apsusc.2011.12.006.
- B. Lin, C. Xue, X. Yan, G. Yang, G. Yang and B. Yang, Facile fabrication of novel SiO2/g-C3N4 core–shell nanosphere photocatalysts with enhanced visible light activity, Appl. Surf. Sci., 2015, 357, 346–355, DOI:10.1016/j.apsusc.2015.09.041.
-
(a) T. Vijayaraghavan, M. Bradha, P. Babu, K. M. Parida, G. Ramadoss, S. Vadivel, A. Selvakumar and A. Ashok, Influence of secondary oxide phases in enhancing the photocatalytic properties of alkaline earth elements doped LaFeO3 nanocomposites, J. Phys. Chem. Solids, 2020, 140, 109377 CrossRef CAS;
(b) J. Sopousek, O. Zobac, V. Vykoukal, J. Bursik, P. Roupcova, P. Broz, J. Pinkas and J. Vrestal, Temperature Stability of AgCu Nanoparticles, J. Nanopart. Res., 2015, 17(12), 478–484 CrossRef.
- P. Babu and B. Naik, Cu–Ag Bimetal Alloy Decorated SiO2@TiO2 Hybrid Photocatalyst for Enhanced H2 Evolution and Phenol Oxidation under Visible Light, Inorg. Chem., 2020, 59(15), 10824–10834 CrossRef CAS.
- Y. Wu, Y. Lin and J. Xu, Synthesis of Ag–Ho, Ag–Sm, Ag–Zn, Ag–Cu, Ag–Cs, Ag–Zr, Ag–Er, Ag–Y and Ag–Co metal organic nanoparticles for UV-Vis-NIR wide-range bio-tissue imaging, Photochem. Photobiol. Sci., 2019, 18(5), 1081–1091, 10.1039/C8PP00493E.
- Z. Liu, Y. Huang, Q. Xiao and H. Zhu, Selective reduction of nitroaromatics to azoxy compounds on supported Ag–Cu alloy nanoparticles through visible light irradiation, Green Chem., 2016, 18(3), 817–825, 10.1039/C5GC01726B.
- J. Guo, H. Yu, F. Dong, B. Zhu, W. Huang and S. Zhang, High efficiency and stability of Au–Cu/hydroxyapatite catalyst for the oxidation of carbon monoxide, RSC Adv., 2017, 7(72), 45420–45431, 10.1039/C7RA08781K.
- P. Babu, S. Mohanty, B. Naik and K. Parida, Synergistic effects of boron and sulfur Co-doping into graphitic carbon nitride framework for enhanced photocatalytic activity in visible light driven hydrogen generation, ACS Appl. Energy Mater., 2018, 1(11), 5936–5947 CrossRef.
- R. Patil, S. Kelkar, R. Naphade and S. Ogale, Low temperature grown CuBi2O4 with flower morphology and its composite with CuOnanosheets for photoelectrochemical water splitting, J. Mater. Chem. A, 2014, 2(10), 3661–3668, 10.1039/C3TA14906D.
- D. Nelli and R. Ferrando, Core–shell vs. multi-shell formation in nanoalloy evolution from disordered configurations, Nanoscale, 2019, 11(27), 13040–13050, 10.1039/C9NR02963J.
- S. K. Dutta, S. K. Mehetor and N. Pradhan, Metal semiconductor heterostructures for photocatalytic conversion of light energy, J. Phys. Chem. Lett., 2015, 6(6), 936–944, DOI:10.1021/acs.jpclett.5b00113.
- L. Zhang, L. O. Herrmann and J. J. Baumberg, Size dependent plasmonic effect on BiVO4 photoanodes for solar water splitting, Sci. Rep., 2015, 5(1), 1–12 Search PubMed.
- C. Gomes Silva, R. Juarez, T. Marino, R. Molinari and H. Garcia, Influence of excitation wavelength (UV or visible light) on the photocatalytic activity of titania containing gold nanoparticles for the generation of hydrogen or oxygen from water, J. Am. Chem. Soc., 2011, 133(3), 595–602 CrossRef CAS.
- D. E. Eastman, Photoelectric work functions of transition, rare-earth, and noble metals, Phys. Rev. B: Solid State, 1970, 2(1), 1, DOI:10.1103/PhysRevB.2.1.
- R. T. Tung, The physics and chemistry of the Schottky barrier height, Appl. Phys. Rev., 2014, 1(1), 011304, DOI:10.1063/1.4858400.
- J. Tersoff, Schottky barrier heights and the continuum of gap states, Phys. Rev. Lett., 1984, 52(6), 465, DOI:10.1103/PhysRevLett.52.465.
- C. S. Gworek, P. Phatak, B. T. Jonker, E. R. Weber and N. Newman, Pressure dependence of Cu, Ag, and Fe/n-GaAs Schottky barrier heights, Phys. Rev. B: Condens. Matter Mater. Phys., 2001, 64(4), 045322, DOI:10.1103/PhysRevB.64.045322.
- D. Tsukamoto, A. Shiro, Y. Shiraishi, Y. Sugano, S. Ichikawa, S. Tanaka and T. Hirai, Photocatalytic H2O2 production from ethanol/O2 system using TiO2 loaded with Au–Ag bimetallic alloy nanoparticles, ACS Catal., 2012, 2(4), 599–603 CrossRef CAS.
Footnote |
† Electronic supplementary information (ESI) available: EDX spectra, XPS survey spectra of SCSC-1 : 3, N 1s XPS, PL spectra, literature survey, Ag1Cu3 model structure, Cartesian coordinate, effect of radical scavenger, PXRD and HRTEM images after reusability, energy band diagram of SCS and SCS, spectral pattern of 150 W xenon lamp. See DOI: 10.1039/d1na00633a |
|
This journal is © The Royal Society of Chemistry 2022 |