DOI:
10.1039/D1NA00257K
(Review Article)
Nanoscale Adv., 2021,
3, 3708-3729
Recent advances in graphite carbon nitride-based nanocomposites: structure, antibacterial properties and synergies
Received
5th April 2021
, Accepted 27th May 2021
First published on 28th May 2021
Abstract
Bacterial infections and transmission threaten human health and well-being. Graphite carbon nitride (g-C3N4), a promising photocatalytic antibacterial nanomaterial, has attracted increasing attention to combat bacterial transmission, due to the outstanding stability, high efficiency and environmental sustainability of this material. However, the antibacterial efficiency of g-C3N4 is affected by several factors, including its specific surface area, rapid electron/hole recombination processes and optical absorption properties. To improve the efficiency of the antibacterial properties of g-C3N4 and extend its range of applications, various nanocomposites have been prepared and evaluated. In this review, the advances in amplifying the photocatalytic antibacterial efficiency of g-C3N4-based nanocomposites is discussed, including different topologies, noble metal decoration, non-noble metal doping and heterojunction construction. The enhancement mechanisms and synergistic effects in g-C3N4-based nanocomposites are highlighted. The remaining challenges and future perspectives of antibacterial g-C3N4-based nanocomposites are also discussed.
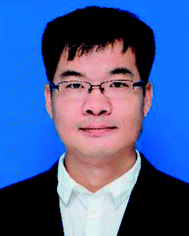 Kai Yan | Kai Yan is a lecturer at the College of Bioresources Chemical and Materials Engineering in Shaanxi University of Science and Technology. He received his PhD degree from Fudan University in 2017. His current research interest is mainly focused on multifunctional nanomaterials for biological applications. |
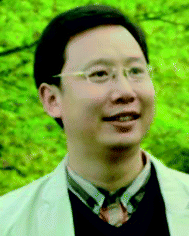 Lingjie Meng | Lingjie Meng is a professor at the School of Chemistry in Xi'an Jiaotong University. He received his PhD degree from Xi'an Jiaotong University in 2005 and was a lecturer in Shanghai Jiaotong University from 2005 to 2010. He became an associate professor in 2011. He was a visiting scholar at the University of Texas at Austin from 2011 to 2012. In 2013, he was appointed as a Professor in Xi'an Jiaotong University. His research interests are focused on the nanomaterials for theranostics. |
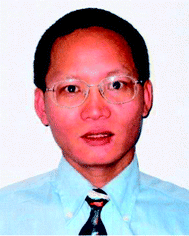 Zhaofu Fei | Zhaofu Fei is a senior research scientist in the Institute of Chemical Sciences and Engineering at the Ecole Polytechnique Fédérale de Lausanne (EPFL). He received his PhD degree from the Technical University of Braunschweig, Germany in 1999. After postdocs in the UK and Germany he joined EPFL in 2002. His research interests are focused on design and synthesis of ionic liquids and related nanomaterials. |
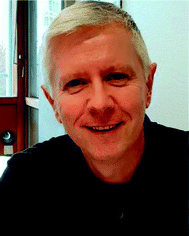 Paul J. Dyson | Paul J. Dyson is a professor in the Institute of Chemical Sciences and Engineering at the EPFL. He received his PhD degree from the University of Edinburgh in 1993 and subsequently held positions at Imperial College of Science, Technology and Medicine and the University of York. His research interests are focused on synthesis and properties of compounds and materials with applications in catalysis and medicine. |
1. Introduction
Bacterial infections and their transmission pose a considerable threat to human health, usually leading to delayed wound healing and chronic intestinal diseases.1,2 Furthermore, pathogenic bacteria frequently contaminate water supplies and the soil, resulting in the death of animals and plants through environmental contamination.3 To address these problems, antibiotics are widely used to kill bacteria, but over-utilization of antibiotics can bring about resistance and secondary contamination.4–6 Therefore, the development of new strategies to inactivate bacteria without using antibiotics is urgently required as is the inactivation of drug-resistant bacteria. In recent years, semiconductor photocatalysis has attracted significant interest for applications in pollutant degradation7 and antimicrobial applications.8 Under sunlight irradiation, semiconductor photocatalysts react with water and oxygen to form reactive oxygen species (ROS), such as hydrogen peroxide (H2O2), hydroxyl radicals (˙OH) and superoxide (O2˙−), which are able to inactivate bacteria by oxidizing the phospholipid membrane, proteins and nucleic acids.9–13 Common photocatalysts include metallic oxides, sulfides, nitrides, and phosphides, and graphene and its derivatives,14,15 which show bactericidal activity against both Gram-positive and Gram-negative bacteria, as demonstrated in many studies. However, relatively narrow spectral absorption ranges result in low efficiencies.16 Together with other problems such as facile aggregation, potential toxicity, and low biocompatibility, practical application of these common photocatalysts is limited. As an emerging non-metallic photocatalyst, graphitic carbon nitride (g-C3N4) is easy to prepare and has an appropriate band structure and good biocompatibility,17 thus showing considerable potential as antibacterial materials.
Similar to the layered structure of graphene, g-C3N4 is a polymeric layered material which consists of carbon and nitrogen with some hydrogen (impurity). The conduction band (CB) and valence band (VB) position of g-C3N4 are ∼1.13 and 1.57 eV, respectively. The appropriate band gap is about 2.70 eV, i.e. it is a medium band gap semiconductor, readily obtained from the pyrolysis of melamine, dicyandiamide or urea. Benefiting from the connection of tri-s-triazine units through tertiary amines (Fig. 1), g-C3N4 has a two-dimensional flake structure. The adjacent g-C3N4 flakes interact weakly with each other via van der Waals forces, displaying a layer gap of about 0.33 nm.18–22 Thus, when such forces between the bulk g-C3N4 layers are broken, paper-like g-C3N4 nanosheets are obtained.23–27 From a molecular prospective, C3N4 is considered to have two main molecular structures. One comprises a triazine with a tertiary nitrogen atom in the center of a planar triangle (Fig. 1a) that connects three separate triazine rings in an infinitely repeating pattern.28 Another is a tri-s-triazine structurally connected to form g-C3N4 (Fig. 1b). This more stable tri-s-triazine structure is commonly used as the structural unit of g-C3N4.29 These excellent structural features and properties make C3N4 a promising photocatalytic material. Since the first study of the photocatalytic activity of g-C3N4 by Wang et al. in 2009, it has become a prevalent photocatalytic material.30 Due to the merits of the non-metallic g-C3N4 material, such as a wide visible light absorption range, excellent chemical stability and low toxicity, it has been widely studied to tackle environmental and energy related problems.31–35 Specifically, g-C3N4 has been used as a catalyst for photocatalytic water reduction and oxidation, contaminant degradation and carbon dioxide reduction.36–41 For photocatalytic degradation, the photo-produced electrons (e−) and holes (h+) can accelerate reduction and oxidation degradation reactions.42–47 In addition, the e− and h+ can react with surrounding H2O and O2 to generate ROS such as ˙O2− and ˙OH−. The generated ROS can further degrade pollutants, combining to achieve the removal of contaminants.48–51
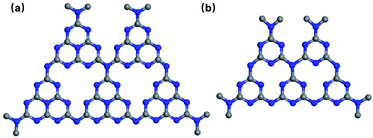 |
| Fig. 1 (a) Tri-s-triazine and (b) s-triazine structure of g-C3N4. | |
In this review, several tactics for enhancing the antimicrobial efficiency of g-C3N4-based nanocomposites are discussed (Fig. 2), including the design of different g-C3N4 topologies,52–55 noble metal decoration,56–58 non-noble metal doping and heterojunction construction.59–61 These approaches have been shown to effectively boost the antibacterial activity of g-C3N4.62–67 The enhancement mechanisms and synergistic effects of g-C3N4-based nanocomposites is highlighted. Additionally, photocatalytic mechanisms have been elucidated by analyzing the interactions between the nanomaterials and bacteria. Lastly, this review concludes by defining future prospects, opportunities and challenges in this exciting field.
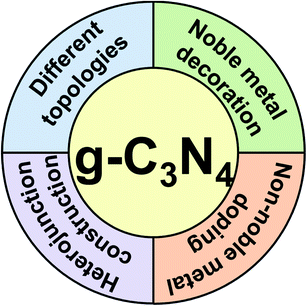 |
| Fig. 2 Schematic illustration of antimicrobial enhancement of g-C3N4-nanomaterials. | |
2. g-C3N4-based materials and their photocatalytic antibacterial
Both the nitrogen and carbon atoms in g-C3N4 are sp2 hybridized to form a conjugated structure with delocalized π-electrons, giving g-C3N4 the lowest bandgap compared to other phases of C3N4.68 Compared to other conventional photocatalytic materials, g-C3N4 has a narrow band gap (2.7 eV), resulting in a wider spectral absorption range of up to 460 nm, and improving the photocatalytic window.69 The photocatalytic reactions of g-C3N4 affect its antibacterial performance. When the energy of the visible light illuminating g-C3N4 is larger than the band gap energy of g-C3N4, e− are promoted from the valence band (VB) to the conduction band (CB), producing active e− and h+. However, the e−/h+ can recombine on the g-C3N4 surface. Alternatively, the e−/h+ pairs diffuse or are transported to the g-C3N4 interface by an electric field and undergo redox reactions with the surroundings. As shown in Fig. 3, under visible light illumination, g-C3N4 produces ROS that can destroy the bacterial membranes, causing cell membrane permeability, structural degradation and ultimately killing the bacterial.70
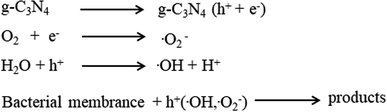 |
| Fig. 3 Plausible reactions between bacterial and g-C3N4-based nanocomposite generated ROS. | |
2.1 Influence of topology on g-C3N4-based antibacterial photocatalysts
The antibacterial activity of g-C3N4 is influenced by its topology, in particular, the efficiency of bulk g-C3N4 is restricted by its small superficial area and rapid rate of recombination of photogenerated carriers.71–75 In contrast, mesoporous g-C3N4, g-C3N4 nanotubes and nanosheets absorb visible light more effectively and provide a larger contact area for reactants because of their larger specific surface areas and a larger number of actives sites.76,77 In addition, these structures decrease the distance required for the transfer of the charge to the surface of the material, decreasing charge recombination.78,79 Moreover, due to the quantum size effects, g-C3N4 nanosheets are suited to charge transfer and separation processes.80,81 Therefore, g-C3N4 nanosheets and their composite materials show promising photocatalytic antibacterial properties. Li et al. developed a self-cleaning antibacterial membrane by simply filtering g-C3N4 nanosheets into polyacrylonitrile porous substrates (Fig. 4a), then forming a stable coating by cross-linking polyvinyl alcohol and glutaraldehyde.82 In contrast to membranes without g-C3N4 nanosheets, the membranes containing the g-C3N4 nanosheets (0.45 wt%) completely inactivated 1 × 106 cfu mL−1E. coli under irradiation with visible light (Fig. 4b and c). The high superficial area of the g-C3N4 nanosheets in the membrane provides more active sites that produce ROS for sterilization. Meanwhile, the membranes with g-C3N4 nanosheets also showed good permeability to water and degraded dyes. Compared to g-C3N4 nanosheets, nanotubes have high aspect ratios, a topology that favors the migration of e− along the axial direction and inhibits the lateral transfer of e−, thereby inhibiting the recombination of photogenerated carriers. Moreover, nanotubes usually have large specific surface areas, providing a higher density of actives sites at their surface, which improves photocatalytic antibacterial performance.83,84 Xu et al. successfully synthesized microtubular nanoporous g-C3N4 with a layered structure and nitrogen defects using molecular self-assembly methods.85 The hexagonal tubular structure promotes the multiple use of light, and provides a larger density active sites and a directional transfer channel for e−. Moreover, the nanoporosity of the material increases the specific surface area to provide rich charge transport paths. In addition, the nitrogen vacancies improve the light harvesting properties of the material (λ > 450 nm) and promote charge separation by trapping charge. Hence, microtubular nanoporous g-C3N4 completely inactivated 5 × 106 cfu mL−1E. coli after 4 h of light illumination, compared to only 74% of E. coli sterilized by bulk g-C3N4. Hollow porous microspheres not only promote light penetration within the material and light absorption at the pore edges, but also provide sufficient contact area to accelerate interfacial charge transfer. In addition, the thinner pore wall structure reduces the distance (and time) required for charge transfer within the material, decreasing the recombination of photogenerated carriers.86 Yang et al. successfully fabricated a self-cleaning, antimicrobial and antifouling membrane by integrating mesoporous g-C3N4 (MCN) into polyvinylidene fluoride (PVDF).87 The mesoporous structure promotes multiple reflections of incident light and enhances the capacity of the material for light capture, leading to an enhancement in the generation of h+ and ROS. The MCN-PVDF membrane showed a significant reduction in the number of E. coli colonies under illumination with visible light over 4 hours, with approximately 3
log deactivation of E. coli.88,89 In contrast, an analogous experiment using a membrane-free material showed no significant decrease in E. coli communities over the same time period. Additionally, under visible light, MCN-PVDF degrades the antibiotic cefotaxime (CFX) with a degradation rate of over 97% over five cycles.
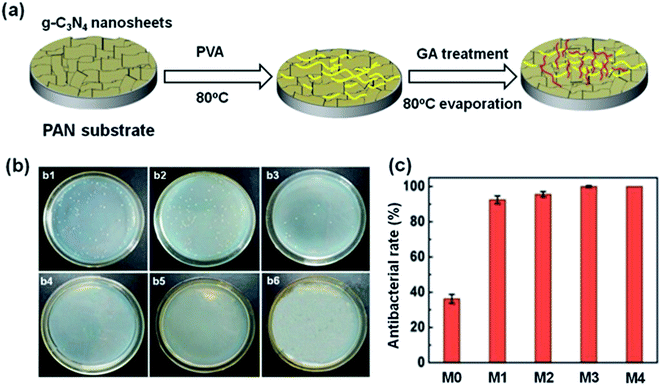 |
| Fig. 4 (a) Preparation of g-C3N4 nanosheet-functionalized composite membranes. The concentration of g-C3N4 nanosheets in the membrane are 0, 0.15 wt%, 0.3 wt%, 0.45 wt%, 0.6 wt% in M0, M1, M2, M3, M4, respectively. (b) Antimicrobial activities against E. coli of (b1) control, (b2) M0, (b3) M1, (b4) M2, (b5) M3, (b6) M4. (c) Antibacterial rate of membranes M0, M1, M2, M3, M4. Reproduced from ref. 82 with permission from Royal Society of Chemistry, copyright 2017. | |
When g-C3N4 has a large specific surface area its photocatalytic performance is enhanced, e.g. in g-C3N4 nanosheets and nanotubes, which have a high density active sites. Furthermore, g-C3N4 nanocomposites inhibit e−/h+ pair recombination and have high charge transfer efficiency due to enhanced visible light absorption. Hence, discrepant topologies of g-C3N4 should have outstanding antibacterial performance and the discrepant topologies of g-C3N4-based materials and their corresponding antibacterial properties are summarized in Table 1.
Table 1 Antibacterial properties of g-C3N4 based materials with different topologies
Material |
Preparation |
Bacteria |
Effect |
Ref. |
g-C3N4 nanosheets |
Acid etching and ultrasound |
E. coli
|
∼100% |
82
|
Microtubular nanoporous g-C3N4 |
Molecular self-assembly |
E. coli
|
99.2% |
85
|
g-C3N4 nanosheets |
Freezing and microwave-assisted |
E. coli
|
100% |
90
|
Porous g-C3N4 nanosheets |
Template-free |
E. coli
|
100% |
91
|
g-C3N4 nanosheets |
Bacterial etching |
E. coli
|
3.65 log |
92
|
g-C3N4 nanosheets |
Ultrasound |
E. coli O157:H7
|
0.82 log |
93
|
S. aureus
|
0.85 log |
g-C3N4 nanosheets |
Chemical exfoliation |
E. coli K-12
|
6.5 log |
94
|
g-C3N4 nanosheets |
Ultrasound |
E. coli
|
99% |
95
|
bare g-C3N4 |
Calcination |
MS2
|
100% |
96
|
Mesoporous g-C3N4 |
Immersion-precipitation phase transformation |
E. coli
|
3 log |
87
|
Mesoporous g-C3N4 |
Template method |
E. coli K-12
|
99% |
97
|
Mesoporous g-C3N4 |
Thermal polymerization and selective dialysis approach |
E. coli
|
99% |
98
|
S. aureus
|
90% |
Ag2WO4/mesoporous g-C3N4 |
Polymerization |
E. coli
|
100% |
99
|
GO quantum dots/oxidized nanoporous g-C3N4 |
Self-assembly |
E. coli
|
99.6% |
100
|
Nanomesh g-C3N4 |
Template method |
E. coli K-12
|
85% |
101
|
CuInSe2:Zn/g-C3N4/TiO2 nanowire |
In situ growth |
S. aureus
|
90% |
102
|
Mesoporous g-C3N4 |
Thermal, polycondensation |
E. coli
|
Effective |
103
|
Porous g-C3N4 |
Calcination |
S. aureus
|
99% |
104
|
Mesoporous g-C3N4 |
Calcination |
E. coli
|
Effective |
105
|
Nanostructured g-C3N4 |
Calcination |
E. coli O157:H |
97.1% |
106
|
S. aureus
|
93.7% |
Mesoporous g-C3N4 |
Hydrothermal |
E. coli
|
Effective |
107
|
g-C3N4 powder |
Calcination |
E. coli
|
Effective |
108
|
S. epidermidis
|
2.2 Noble metal decorated g-C3N4 nanocomposites
Another strategy used to increase the antibacterial performance of g-C3N4 involves modification with noble metal nanoparticles, including silver and gold nanoparticles.109,110 Surface Plasmon Resonance (SPR) of nanoparticles enhances antibacterial efficiency, by extending the spectral absorption range and promoting the formation of photogenerated carriers in g-C3N4.111–114 Additionally, noble metal nanoparticles act as electron traps, capturing free e− and thus inhibiting the recombination of photogenerated carriers.115–118 Ma et al. developed Ag/g-C3N4 nanocomposites by combining thermal polymerization with photo-assisted reduction.119 A synergistic antibacterial efficiency was achieved with superior sterilization activity of the Ag/g-C3N4 nanocomposite compared to pure g-C3N4 (Fig. 5f). Ag(0.3 wt%)/g-C3N4 exhibited prominent antibacterial performance and suppressed E. coli replication (7.41
log) with only 1.25 h of visible light illumination. In contrast, pure g-C3N4 displayed very low inactivation, with only about (0.4
log) E. coli killed following 1.5 h of illumination by visible light (Fig. 5g). Notably, the loading of noble Ag nanoparticles on the g-C3N4 nanosheets significantly increases the visible light absorption region due to the SPR effect of the Ag nanoparticles and the charge transfer between the Ag and the g-C3N4 nanosheets.120 Similarly, the strong and unique surface plasmon resonance (SPR) absorption of gold nanoparticles covers a wide range of spectra, including the visible and near-infrared light (NIR).121,122 As shown in Fig. 5a, Dai et al. utilized 5–10 nm sized Au nanoparticles to modify g-C3N4via liquid-phase exfoliation of g-C3N4 combined with the photo-deposition of Au nanoparticles.123 When a mixture of E. coli and the Au/g-C3N4 nanocomposite were irradiated at 670 nm the resulting ROS effectively kill the bacteria. The viability of the bacteria continually diminishes over the illumination period (Fig. 5b–e). The incorporation of Au nanoparticles into the g-C3N4 nanosheets strikingly improves photocatalytic ROS generation, due to the application of 670 nm light.124 In general, noble metal/g-C3N4 nanocomposites significantly outperform unmodified g-C3N4 in antimicrobial experiments, and provide a viable photocatalytic disinfection method, see Table 2 for a summary.
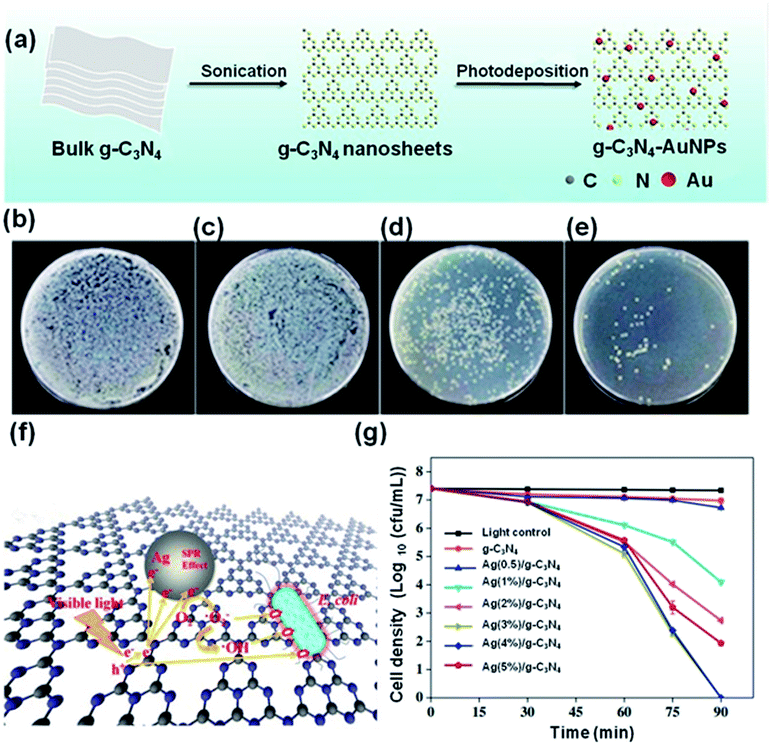 |
| Fig. 5 (a) Preparation of g-C3N4-Au nanoparticle nanocomposties. (b) E. coli bacteria + control sample. (c) E. coli bacteria + g-C3N4-1.0% Au. (d) E. coli bacteria + g-C3N4-1.0% Au + 10 min irradiation. (e) E. coli bacteria + g-C3N4-1.0% Au + 20 min irradiation. Reproduced from ref. 123 with permission from American Chemical Society, copyright 2019. (f) The mechanism of E. coli inactivation in the presence of Ag/g-C3N4 under visible light. (g) Disinfection efficiencies of E. coli by the samples under visible light irradiation. Reproduced from ref. 119 with permission from Elsevier, copyright 2019. | |
Table 2 Antibacterial properties of noble metal decoration and non-noble metal doped g-C3N4 nanocomposites
Main component |
Material |
Preparation |
Bacteria |
Effect |
Ref. |
Noble metal decoration |
Ag/g-C3N4 |
Thermal polymerization and photo-assisted reduction |
E. coli
|
7.41 log |
119
|
Ag/g-C3N4 |
Single-pot, microemulsion |
E. coli
|
9.95 log |
166
|
Ag/P/g-C3N4 |
Pyrolysis and green reduction. |
E. coli
|
7 log |
167
|
Ag/polydopamine/g-C3N4 |
Ultrasound and freeze-drying |
E. coli
|
Effective |
168
|
Ag/oxidized porous g-C3N4 |
Photo-assisted, reduction |
S. aureus
|
99% |
169
|
Ag/g-C3N4 |
Biogenic, methodology |
E. coli
|
Effective |
174
|
S. aureus
|
P. aeruginosa
|
Ag/g-C3N4 |
Calcination |
E. coli
|
Effective |
175
|
S. aureus
|
B. subtili
|
P. aeruginosa
|
Ag/g-C3N4 |
Photo-deposition method |
E. coli
|
Effective |
177
|
P. aeruginosa
|
Au/g-C3N4 |
Liquid-phase, exfoliation and photodeposition |
E. coli
|
Effective |
123
|
Au/g-C3N4 |
Deposition–precipitation |
E. coli
|
82% |
170
|
S. aureus
|
79% |
Au/g-C3N4 |
Calcination |
E. coli
|
99% |
176
|
Non-noble metal doping |
g-C3N4-Zn2+@graphene |
Chemical vapor deposition and ultrasonic dispersion |
S. aureus
|
99% |
158
|
CQDs/g-C3N4 |
Impregnation |
S. aureus
|
7 log |
165
|
Fullerene/g-C3N4 |
Hydrothermal method |
E. coli O157:H7
|
86% |
171
|
S-CQD/hollow tubular g-C3N4 |
Self-assembly and ultrasound |
E. coli
|
6.88 log |
172
|
Ag/P/Co/S/g-C3N4 |
Calcination |
E. coli
|
7 log |
173
|
2.3 Non-noble metal doped g-C3N4 nanocomposites
While g-C3N4 nanocomposites with noble metals have been shown to improve the photocatalytic antibacterial properties of the material, the high cost of noble metals prohibits widespread applications.125–129 Thus, g-C3N4 nanocomposites derived from inexpensive and abundant elements that are non-toxic would be advantageous.130–134 In such materials the g-C3N4 band gap may even be reduced to improve the separation efficiency of photogenerated carriers and the photoabsorption region may even be expanded to further improve the photocatalytic antibacterial performance.135–142 Surface engineering of carbon-based materials has been an effective tool for construction of materials with special functions.143–149 Advantageously, Lewis basic N-sites on the surface g-C3N4 allow strong interactions with Lewis acids, i.e. zinc ions, similar to that observed for other materials.150–157 For example, g-C3N4–Zn2+@graphene oxide (SCN–Zn2+@GO) were prepared using chemical vapor deposition (CVD).158 The bidentate ligand, SCN, may coordinate to the Zn2+ ions to form cross-links with GO, and additionally changing the crystal structure of g-C3N4 and introducing defect sites (Fig. 6a). The resulting SCN–Zn2+@GO nanocomposite possessed excellent antibacterial activity. Irradiation at 808 nm (NIR) led to heating and irradiation at 660 nm resulted in the generation of ROS and the combination of photothermal and photodynamic processes effectively killed bacteria within a short time (almost quantitatively under the conditions employed). In Fig. 6b and d, the E. coli and S. aureus blank groups possess unbroken topologies, with glossy bacteria membranes and intact intra-cell structures. The membrane structures of both E. coli and S. aureus are ruptured under 808 nm and 660 nm light illumination. The intra-cell density decreases and part of cytoplasm overflows (Fig. 6c and e, red arrows indicate protein or intra-cell material leakage and the black arrows indicate bacterial membrane distortion). In comparison, when SCN–Zn2+@GO was exposed to either 808 nm or 660 nm illumination, the inactivation rate obtained was only 20–66%. Based on the above results, the antibacterial mechanism is proposed in Fig. 6f. The ROS pass through the cell membrane of the bacteria to oxidize intracellular proteins and interfere homeostasis, while hyperthermia weakens the activities of the proteins and reduces adenosine triphosphate synthesis, inactiving E. coli and S. aureus within 10 min. Similarly, novel CoB/g-C3N4 nanosheets were successfully prepared by an electrostatic self-assembly process coupled with calcination.159 The interfacial Co–N bond could act as an e− transport channel by accelerating the e− transfer from g-C3N4 to CoB, as supported by density functional theory (DFT) calculations and indirectly evidenced from antibacterial experiments (Fig. 6g). Consequently, the e− induced O2 reduction process is promoted in CoB/g-C3N4, which boosts the generation of ROS (Fig. 6h). Notably, CoB/g-C3N4 exhibited superior disinfection efficacy of 100% against S. aureus with 125 min under visible light irradiation.
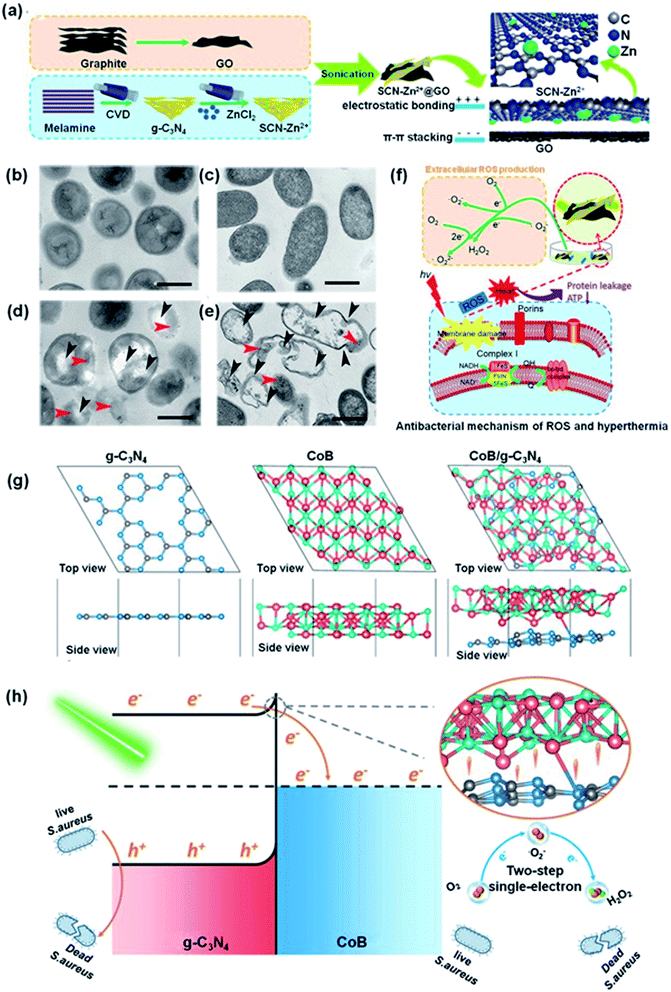 |
| Fig. 6 (a) Preparation of SCN–Zn2+@GO. TEM topology of S. aureus (b) and E. coli (c) as control, (d) and (e) following treatment with SCN–Zn2+@GO 20% after 10 min irradiation with visible light (the red arrows indicate protein leakage and the dark arrows indicate rupture or ruffling of the bacterial membrane). (f) Antibacterial mechanism of SCN–Zn2+@GO 20% under 808 or 660 nm irradiation of ROS and hyperthermia. Reproduced from ref. 158 with permission from WILEY-VCH, copyright 2018. (g) Structural models of the g-C3N4 (001) surface, CoB-(010) surface and CoB/g-C3N4 after geometry optimization. (h) The mechanism of S. aureus bacteria inactivation in the presence of CoB/g-C3N4 under visible light. Reproduced from ref. 159 with permission from the American Chemical Society, copyright 2019. | |
Quantum dots (QDs) are an important low-dimensional semiconductor materials. Because of their high reactivity and strong charge transfer abilities, QDs have been applied in photocatalytic sterilization.160 Carbon quantum dots (CQDs) were combined with g-C3N4 to enhance charge transfer and store e−.161–164 Tang et al. constructed a CQD/g-C3N4 photocatalyst by impregnation.165 The addition of CQDs dramatically increased the disinfection performance, which was attributed to the increased ROS levels. The CQD/g-C3N4 nanocomposites exhibit a greatly enhanced bactericidal efficiency under illumination with visible light. In contrast, the CQDs alone showed no catalytic activity against S. aureus under comparable conditions. Hence, the interaction between the CQDs and g-C3N4 plays a significant role in increasing the bacterial inactivation efficiency.
2.4 g-C3N4 heterojunction nanocomposites
Heterojunctions could enable the directional migration of photoinduced charges, allowing the charge to be enriched in specific direction, a process that should recue or even inhibit the recombination of photogenerated carriers.178,179 The antimicrobial properties of different g-C3N4 heterojunctions are discussed, including type I and type II heterojunctions, p–n heterojunctions, and Z-scheme heterojunctions.180–184 The photocatalytic antibacterial properties of g-C3N4 heterojunction nanocomposites are listed in Table 3.
Table 3 Antibacterial properties of g-C3N4 heterojunction nanocomposites
Material |
Preparation |
Bacteria |
Effect |
Ref. |
Bi2S3/g-C3N4 |
Ultrasound |
E. coli
|
99.6% |
185
|
S. aureus
|
99.2% |
Red P/g-C3N4 |
Sonochemical |
E. coli K-12
|
7 log |
186
|
Perylene diimide/oxygen-doped g-C3N4 |
In situ electrostatic assembling |
S. aureus
|
99.6% |
196
|
Bi2MoO6/g-C3N4 |
In situ solvothermal |
E. coli
|
100% |
199
|
MnO2/g-C3N4 |
Thermal vapor liquid-polymerization and redox |
E. coli
|
99.96% |
212
|
S. aureus
|
99.26% |
TiO2/kaolinite/g-C3N4 |
Sol–gel method |
S. aureus
|
2.9 log |
220
|
Ag/AgBr/g-C3N4/nitrogen-doped graphene aerogel |
Hydrothermal and freeze-drying |
E. coli
|
∼6 log |
252
|
S. aureus
|
∼1.2 log |
Ag/AgBr/g-C3N4 |
In situ deposition–precipitation |
E .coli
|
7.9 log |
253
|
Ag2WO4/g-C3N4 |
Ultrasound |
E. coli
|
90% |
222
|
Ag2PO4/g-C3N4 |
Co-precipitation and thermal pyrolysis |
E. coli
|
Effective |
254
|
S. aureus
|
Ni2P/g-C3N4 |
In situ anchoring and hydrothermal |
E. coli K-12
|
7 log |
255
|
m-Bi2O4/g-C3N |
Hydrothermal |
E. coli K-12
|
6 log |
223
|
Vanadium modified g-C3N4/TiO2 |
Calcination and ultrasonic |
E. coli
|
Effective |
256
|
S. aureus
|
BiVO4/Ag/g-C3N4 |
Photodeposition and hydrothermal |
E. coli
|
6.5 log |
225
|
Ag/ZnO/g-C3N4 |
Thermal polymerization and solvothermal |
E. coli
|
7.4 log |
226
|
Ag/ZnO/g-C3N4 |
Thermal polymerization and solvothermal |
E. coli
|
6.19 log |
257
|
Ag2WO4/Ag/g-C3N4 |
Hydrothermal and situ reductive |
E. coli
|
3.05 log |
258
|
Bi2MoO6–Ag/g-C3N4 |
Hydrothermal method |
E. coli
|
Effective |
259
|
S. aureus
|
α-Fe2O3/CeO2/g-C3N4 |
Hydrothermal method |
E. coli
|
Effective |
260
|
S. aureus
|
CuS/g-C3N4 |
Electrostatic adsorption |
E. coli
|
99% |
250
|
S. aureus
|
98% |
Vanadate QDs/g-C3N4 |
Sol–gel method |
Salmonella H9812
|
96% |
251
|
BiOI/g-C3N4 |
In situ generation |
E. coli
|
96% |
261
|
S. aureus
|
98% |
Cu2O/g-C3N4 |
Chemical precipitation |
E. coli
|
7 log |
262
|
Cu2O/g-C3N4 |
Hydrothermal method |
E. coli
|
Effective |
263
|
S. aureus
|
TiO2 nanofibers/Ag/g-C3N4 |
Ultrasound |
E. coli
|
99% |
264
|
S. aureus
|
83% |
Ag/AgCl/g-C3N4 |
In situ implanting and anchoring |
Tetracycline-resistant bacteria |
100% |
265
|
γ-Fe2O3/Ag/AgCl/g-C3N4 |
Solvothermal and photodeposition |
E. coli
|
5.59 log |
266
|
RGO/CA/g-C3N4 |
Ultrasonification |
E. coli
|
6.5 log |
267
|
TiO2/CuBA/g-C3N4 |
Ultrasound |
E. coli
|
Effective |
268
|
S. aureus
|
Ag2ZrO3/g-C3N4 |
Co-precipitation |
E. coli
|
97% |
189
|
B. subtilis
|
99% |
AgBr/g-C3N4 |
Adsorption deposition |
E. coli
|
6.5 log |
269
|
BiFeO3/Cu2O/g-C3N4 |
Hydrothermally and ultrasonic |
E. coli
|
Effective |
270
|
S. aureus
|
ZnO/g-C3N4/cellulose |
Ultrasonic irradiation |
E. coli
|
Effective |
271
|
S. aureus
|
CdS/g-C3N4 |
Sonochemical |
E. coli
|
Effective |
272
|
S. aureus
|
GO/g-C3N4 |
Sonication |
E. coli
|
97.9% |
70
|
AgCl/CNTs/g-C3N4 |
Deposition–precipitation |
E. coli
|
Effective |
273
|
MoO3−x/g-C3N4 |
Hydrothermal |
E. coli
|
100% |
274
|
AgO/g-C3N4 |
Chemical oxidation |
S. aureus
|
89% |
275
|
Fe-2,5-thiophenedicarboxylic acid/g-C3N4 |
Microwave-heating |
E. coli
|
100% |
276
|
Mg1.2Ti1.8O5/g-C3N4 |
Sol–gel method and calcination |
E. coli
|
100% |
277
|
CuWO4/g-C3N4 |
Sol–gel method |
E. coli
|
Effective |
278
|
S. aureus
|
ZnBi2O4/g-C3N4 |
Ultrasound-assisted chemical exfoliation |
E. coli
|
Effective |
279
|
S. aureus
|
Cr–ZnO/g-C3N4 |
Chemical coprecipitation method |
E. coli
|
Effective |
280
|
S. aureus
|
B. subtilis
|
ZnBi2O4/g-C3N4 |
Thermal polycondensation |
E. coli
|
97% |
281
|
TiO2/Ag/g-C3N4 |
Vacuum freeze-drying |
E. coli
|
84% |
282
|
AgBr/g-C3N4 |
Calcination |
P. putida
|
100% |
283
|
TiO2 nanotubes/Ti/g-C3N4/SnO2 |
Dipping and calcination |
E. coli
|
Effective |
284
|
BiOCl/g-C3N4 |
Hydrothermal method |
E. coli
|
96% |
285
|
NiFe2O4/g-C3N4 |
Hydrothermal method |
A. flavus
|
90% |
286
|
Perylene-3,4,9,10-tetracarboxylic diimide/g-C3N4 |
In situ
|
E. coli
|
Effective |
287
|
S. aureus
|
TiO2/g-C3N4 |
In situ
|
E. coli
|
65% |
288
|
ZnO/Mn/g-C3N4 |
Chemical co-precipitation |
E. coli
|
Effective |
289
|
S. aureus
|
ZnTiO2/S/g-C3N4 |
In situ
|
E. coli
|
Effective |
290
|
S. aureus
|
Ag–ZnO@g-C3N4 |
Physical mixing method |
E. coli
|
Effective |
291
|
S. aureus
|
B. subtilis
|
Poly(vinyl alcohol)/g-C3N4 |
Casting |
P. aeruginosa
|
Effective |
292
|
Polyaniline/g-C3N4 |
In situ oxidative polymerization methodology |
E. coli
|
Effective |
293
|
S. pneumoniae
|
PVA/Starch/Ag@TiO2/g-C3N4 |
Solution casting |
E. coli
|
Effective |
294
|
S. aureus
|
Fe@ZnO/g-C3N4 |
Chemical co-precipitation |
E. coli
|
Effective |
295
|
S. aureus
|
B. subtilis
|
S. salivarius
|
g-C3N4-based metal-free |
Calcination |
E. coli
|
Effective |
296
|
B. subtilis
|
Ag2S/g-C3N4 |
Sonochemical |
E. coli
|
Effective |
297
|
S. aureus
|
B. subtilis
|
S. salivarius
|
TiO2 nanofibers/g-C3N4 |
Electrospinning-calcination |
E. coli
|
100% |
298
|
S. aureus
|
Ag3PO4/g-C3N4 |
Hydrothermal method |
E. coli
|
Effective |
299
|
Ag2O/g-C3N4 |
Chemical deposition method |
M. aeruginosa
|
99% |
300
|
TiO2/g-C3N4 |
Hydrothermal and calcination |
E. coli
|
100% |
301
|
Ag2O/g-C3N4 |
Physical mixing method |
M. aeruginosa
|
100% |
302
|
AgO/g-C3N4 |
In situ
|
E. coli
|
Effective |
303
|
Ag3PO4/g-C3N4 |
Hydrothermal method |
Bacteriophage f2
|
100% |
304
|
AgBr/g-C3N4 |
In situ
|
E. coli
|
100% |
305
|
TiO2/g-C3N4 |
Hydrothermal |
E. coli
|
100% |
306
|
Porphyrin/g-C3N4 |
In situ
|
S. aureus
|
63% |
307
|
Ag/Ag/Ag/g-C3N4/BiVO4 |
In situ
|
E. coli
|
100% |
308
|
ZnO–Cd/g-C3N4 |
In situ
|
E. coli
|
Effective |
309
|
S. aureus
|
RGO/S8/g-C3N4 |
In situ
|
E. coli K-12
|
100% |
310
|
2.4.1 Type I heterojunction nanocomposites.
In general, type I heterojunctions are rarely considered as the optimal choice in photocatalysis because the photogenerated carriers can transfer to the interface with other semiconductors, reducing the redox capacity of the charge carriers. Nevertheless, under visible light irradiation, type I heterojunctions have the unique advantage, i.e. the e− and h+ can be transferred from one semiconductor to another. If another semiconductor has a wide photoabsorption window, a broad-spectrum-response photocatalyst with minimal charge recombination can be obtained by creating a type I heterojunction (Fig. 7a). Li et al. developed zinc-doped g-C3N4 (g-C3N4–Zn) with Bi2S3 nanorod heterojunctions (g-C3N4–Zn/BiS), using ultrasonication.185 In contrast to the precursors (g-C3N4–Zn and BiS), effective charge separation at the photocatalyst interface is achieved by adjusting the band gap, the density of the electronic distribution, and the oxygen adsorption capacity of the g-C3N4–Zn/BiS heterojunction. DFT calculations were employed to predict the stable crystal structure and the interface space between CN–Zn and BiS (Fig. 8a). The e− and h+ were separated effectively by the energy band offset and the interface electric field, hence the g-C3N4–Zn/BiS heterojunction produces abundant ROS and shows excellent photocatalytic efficiency. Near-quantitative bactericidal efficiency towards S. aureus was achieved after only 10 min of NIR irradiation (Fig. 8b). In addition, red P was a novel single-element photocatalyst, and its visible light response range is up to 700 nm. Efficient light harvesting is imperative for photocatalysts, and with this in mind Wang et al. developed a wide-spectral-response g-C3N4/red P photocatalyst using ultrasound.186–188 Ultrasonication was used to obtain nanosheets from bulk g-C3N4, and red P particles were anchored to the g-C3N4 nanosheets to construct close g-C3N4/red P heterojunctions. g-C3N4/red P may form a new wide-spectral-responsive photocatalytic system to fully utilize the solar energy. In addition, g-C3N4/red P was used as a dual activity center photocatalyst, exhibiting dramatically improved photocatalytic efficiency for sterilization under illumination with visible light. While the g-C3N4/red P nanocomposite showed 7
log cfu mL−1 bacterial inactivation after 1.3 h, the photocatalytic bacterial inactivation of pure g-C3N4 was limited, with < 1.5
log cfu mL−1E. coli inactivation after 2 h of illumination.
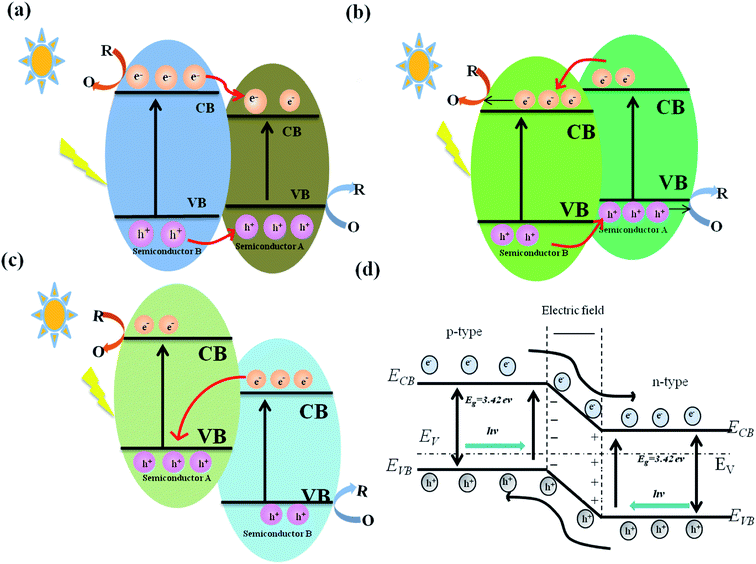 |
| Fig. 7 Various types of heterojunctions. (a) Type I heterojunction model. (b) Type II heterojunction model. (c) Z-type heterojunction model. (d) p–n heterojunction model. | |
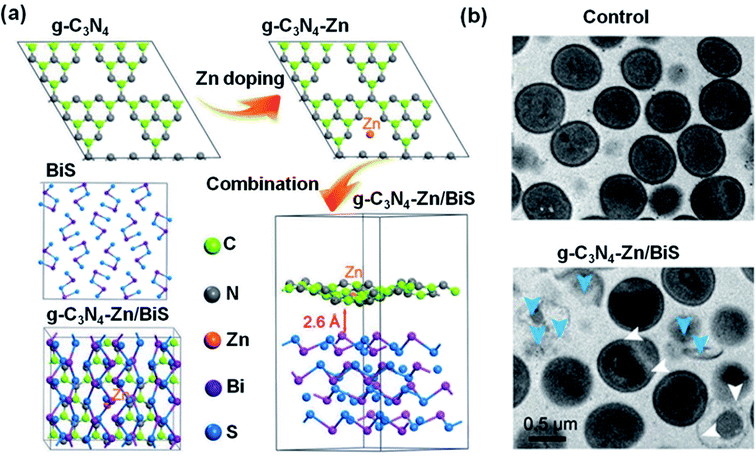 |
| Fig. 8 (a) Structural models of g-C3N4, g-C3N4–Zn, BiS, and g-C3N4–Zn/BiS after geometry optimization. (b) TEM topology of S. aureus after treatment with control or g-C3N4–Zn/BiS after 10 min irradiation. The white arrows indicate twisted and broken cell membranes and the blue arrows point to intracellular matrix outflow. Reproduced from ref. 185 with permission from WILEY-VCH, copyright 2019. | |
2.4.2 Type II heterojunction nanocomposites.
Type II heterojunctions g-C3N4-based nanocomposites have been widely reported as photocatalysts, e.g. Ag2ZrO3/g-C3N4,189 Nb2O5/g-C3N4 and Bi2MoO6/g-C3N4.190,191 These materials have interlaced band gaps and appropriate VB and CB energies. Staggered heterojunctions are the most efficient type of heterojunctions due to highly efficient charge transfer,192–194 and therefore, type II heterojunctions are widely used.195 Exposure of a type II heterojunction to visible light results in the transition of an e− from the VB to the CB, generating a corresponding h+ in the VB. When the CB of semiconductor A is higher in energy than the CB of semiconductor B, the e− in the CB of semiconductor A is transferred to the CB of semiconductor B. Simultaneously, the h+ in the VB of semiconductor B is transferred to the VB of semiconductor A. Finally, they react with O2 and H2O in the surrounding media to produce ROS, leading to good antibacterial effects (Fig. 7b). Gao et al. prepared a perylene diimide (PDI)/oxygen-doped g-C3N4 nanosheet (PDI/O-g-C3N4) nanocomposites using an in situ electrostatic assembly method.196 The PDI expanded the visible light range of the material, resulting in abundant photogenerated charge carriers and accumulation of ROS, boosting the oxidative capability. As a consequence, PDI/O-g-C3N4 demonstrated strong antibacterial oxidation activity under visible light with 96% of the S. aureus fully inactivated by PDI/O-g-C3N4 under 3 h of light irradiation, whereas only 62% of the S. aureus cells were inactivated by the control material. Coincidentally, Bi2MoO6 not only intersects the g-C3N4 band gap, but also has a very similar band gap energy (∼2.7 eV). Consequently, Bi2MoO6 combines with g-C3N4 to afford neoteric and efficient nanocomposites.197,198 As shown in Fig. 9a, Li et al. developed Bi2MoO6/g-C3N4 heterojunctions using an in situ solvothermal approach.199 The results showed that the photocatalyst completely inactivated 2.5 × 107 cfu mL−1E. coli after 3 h light irradiation (Fig. 9b).
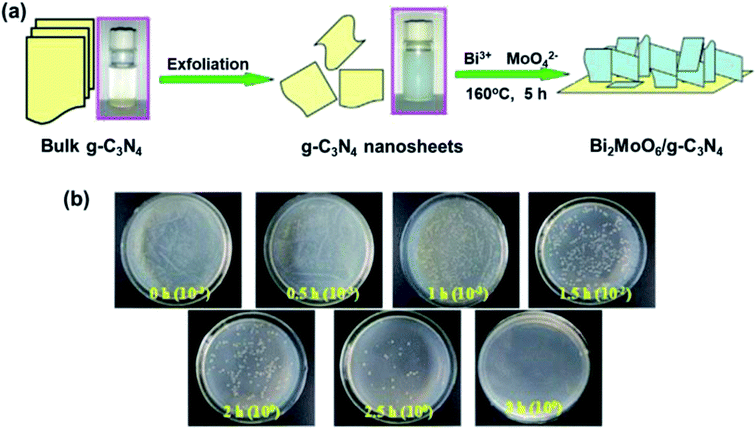 |
| Fig. 9 (a) Preparation of the Bi2MoO6/g-C3N4 heterojunction. (b) E. coli re-cultured after treatment with 20% Bi2MoO6/g-C3N4 as a function of time. Reproduced from ref. 199 with permission from Elsevier, copyright 2016. | |
2.4.3 Z-scheme heterojunction nanocomposites.
Recently, Z-scheme heterojunctions have been widely studied as the structure accelerates the separation of photogenerated carriers. The e− in the VB of semiconductor A transfers to the CB of semiconductor B, and the remaining h+ and e− undergo redox reactions with the oxygen and water in the surroundings to generate ROS (Fig. 7c). As expected, Z-scheme heterojunctions exhibit excellent photocatalytic disinfection performance.200–204 MnO2 is an inexpensive, abundant, biocompatible semiconductor that has a similar bandgap to g-C3N4.205–211 Wu et al. successfully constructed a MnO2/g-C3N4–Ti heterojunction using thermal vapor liquid-polymerization and redox methods212 (Fig. 10a). Contact between the g-C3N4 and MnO2 formed a Z-scheme heterojunction. The MnO2/g-C3N4–Ti composite inactivates S. aureus and E. coli in near-quantitative yields (Fig. 10b and c). In addition, TiO2 is an outstanding photocatalyst that binds with g-C3N4 for form a nanocomposite with high thermal stability.213–219 Li et al. constructed a g-C3N4/TiO2/kaolinite heterojunction using a sol–gel approach combined with self-assembly220 (Fig. 10d). Compared to bulk g-C3N4 or TiO2, the 3D structured g-C3N4/TiO2/kaolinite nanocomposite displayed increased adsorption-photocatalytic sterilization of S. aureus under light irradiation. The g-C3N4/TiO2/kaolinite composite inactivated 2.9
log S. aureus bacteria after 5 h of illumination, superior to g-C3N4 (1.6
log) and TiO2 (0.8
log) alone (Fig. 10e). In addition, under visible light, the g-C3N4/TiO2/kaolinite nanocomposite exhibits heightened adsorption-photocatalytic degradation of ciprofloxacin, a broad-spectrum antibiotic. The antibacterial efficiency of the g-C3N4/TiO2/kaolinite composite may be attributed to both the improved light utilization and an increase in e− transfer and separation efficiency (Fig. 10f). The visible light activated g-C3N4/TiO2/kaolinite composite is a useful material for pollutant decomposition and bacterial elimination.221
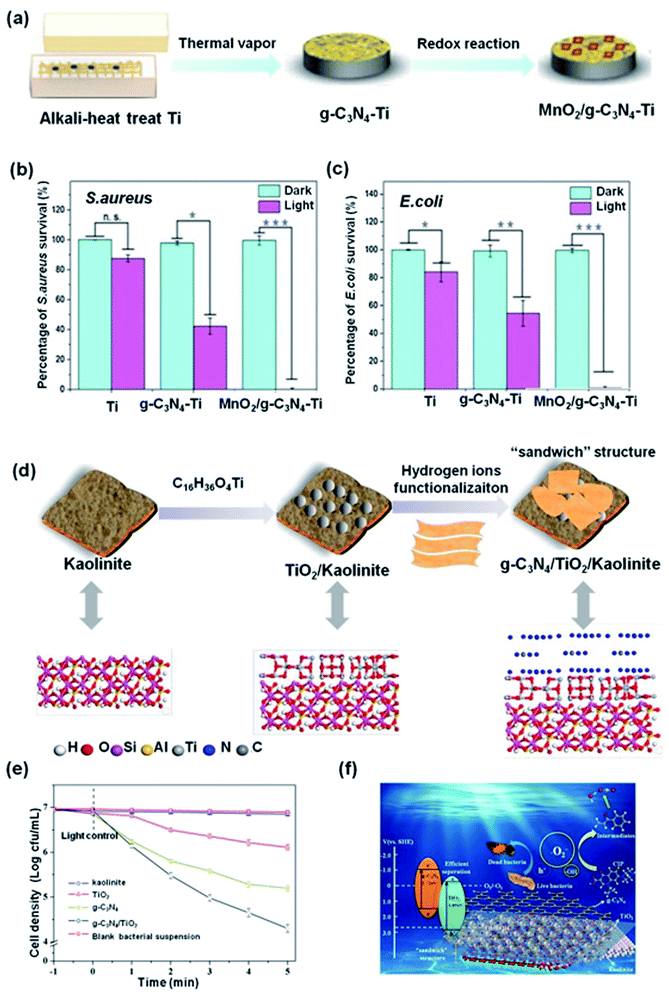 |
| Fig. 10 (a) Schematic showing the preparation of MnO2/g-C3N4–Ti. (b and c) The antibacterial effect of MnO2/g-C3N4–Ti irradiated for 20 minutes against S. aureus and E. coli, respectively. Reproduced from ref. 212 with permission from Elsevier, copyright 2019. (d) Schematic illustration of the preparation of g-C3N4/TiO2/kaolinite. (e) Photocatalytic disinfection efficiency of S. aureus for different samples. (f) Schematic diagram of photocatalytic mechanism of the g-C3N4/TiO2/kaolinite. Reproduced from ref. 220 with permission from Elsevier, copyright 2019. | |
2.4.4 Dual-path heterojunction nanocomposites.
Generally, the charge migration paths observed in g-C3N4 heterojunctions are mostly type II and Z-scheme heterojunctions, which expedite the fast separation of photogenerated charges and intensify the antibacterial activity of semiconductor materials. Many type II and Z-scheme heterojunction nanocomposites have been shown to inactivate bacteria under irradiation with visible light, including m-Bi2O4/g-C3N4, AgWO4/g-C3N4.222,223 Nevertheless, due to the relatively low redox potentials in type II and Z-scheme heterojunctions, these photocatalysts lack strong redox abilities.224 It is known that e− accumulate in the CB of semiconductor A, which has a high reduction potential, and h+ leave the VB of semiconductor B, which has a high oxidation potential. This not only effectively separates the e−/h+ pairs, but also produces the optimal redox properties. Therefore, the two models of ternary heterojunctions were also studied to further improve the antibacterial performance of photocatalytic heterojunctions. Zeng et al. constructed a ternary BiVO4/Ag/g-C3N4 heterojunction using photo-deposition and hydrothermal methods.225 Based on heterojunction band gap energy level and surface chemistry, a dual Z-scheme photogenerated carrier transfer model was applied to BiVO4/Ag/g-C3N4. Notably, the ternary BiVO4/Ag/g-C3N4 heterojunction markedly strengthened the photocatalytic antibacterial capability, completely inactivating 6.5
log E. coli cells after 1 h of light illumination, whereas the binary BiVO4/g-C3N4 heterojunction inactivated only 0.5
log E. coli under comparable conditions. The Ag and BiVO4 nanoparticles on the g-C3N4 nanosheets inhibit recombination of the photogenerated carriers, thus promoting ROS generation. Ma et al. developed ZnO/Ag/g-C3N4 heterojunction using a solvothermal reaction.226 This composite was used to kill E. coli under illumination with visible light. The ZnO/Ag/g-C3N4 composite demonstrated significant visible light sterilization efficiency compared to g-C3N4, Ag/g-C3N4 and ZnO/g-C3N4 materials. Specifically, ZnO/Ag/g-C3N4 inactivated 7.4
log E. coli after 2 h light illumination. However, only 0.49
log and 2.61
log E. coli were inactivated by g-C3N4 and ZnO/g-C3N4. The interface of ZnO improves the sterilization performance by increasing the separation rate of charges because of the SPR effect of Ag and the similar band gap energies of ZnO to g-C3N4.227–231
2.4.5 p–n heterojunction nanocomposites.
The construction of p–n type heterojunctions can increase the spectral response range of photocatalytic semiconductors.232–234 The p–n type heterojunction needs to form at the interface of the space charge region and these heterojunctions form an internal potential that guides the e− and h+ in opposite directions.235–239 The e− transfers to the CB of the n-type semiconductor, whereas the h+ transfers to the VB of the p-type semiconductor (Fig. 7d). The separation effect of charges in p–n heterojunctions is higher than other heterojunctions leading to superior photocatalytic antibacterial activity.240–245 CuS is a p-type semiconductor and is the material of choice for photocatalysis due to its narrow band gap and excellent physicochemical stability.246–249 Ding et al. synthesized CuS/g-C3N4 heterojunction using a hydrothermal approach harnessing electrostatic adhesion.250 In the CuS/g-C3N4 heterojunction (Fig. 11a), the e− and h+ transfer in reverse directions between g-C3N4 and CuS, accelerating the separation of charges, thus producing high levels of ROS and increasing the photocatalytic antibacterial activity. In addition, the CuS/g-C3N4 heterojunction can transform visible light to heat (Fig. 11b). Hence, due to the synergistic influence of the ROS and thermal effects, the CuS/g-C3N4 composite inactivated E. coli and S. aureus bacteria near-quantitatively after 20 min of light irradiation. In contrast, g-C3N4 only inactivated 30% of the E. coli cells and 25% of the S. aureus cells (Fig. 11c).
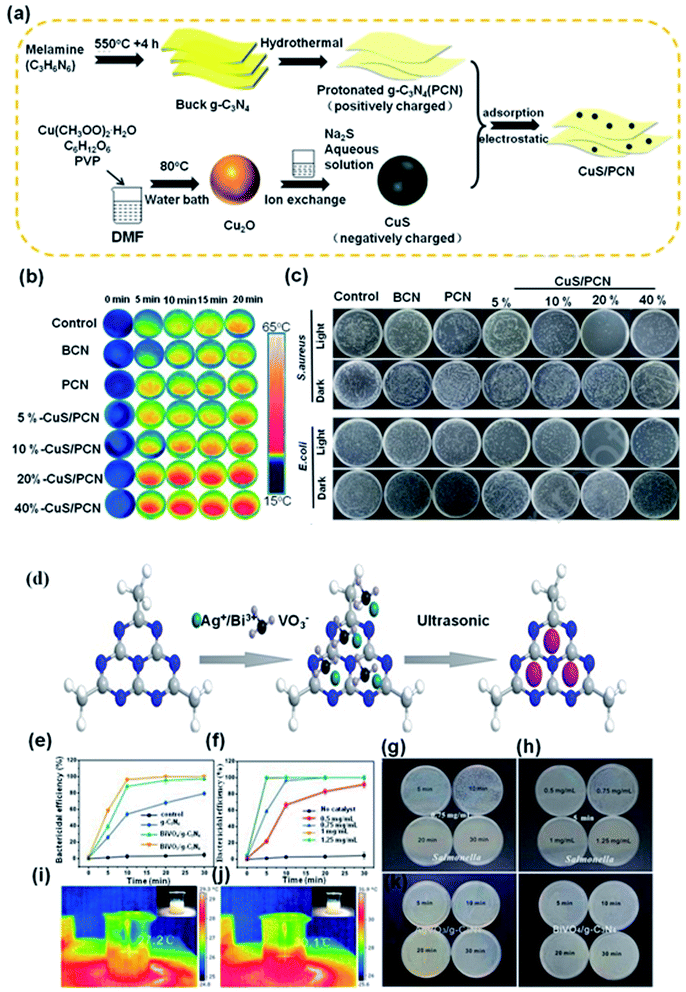 |
| Fig. 11 (a) Preparation of CuS/g-C3N4. (b) Photothermal images following irradiation as a function of concentration and time. (c) In vitro antibacterial activity for S. aureus and E. coli. Reproduced from ref. 250 with permission from Elsevier, copyright 2020. (d) Formation mechanism of vanadate QDs/g-C3N4. (e) Photocatalytic disinfection efficiency of Salmonella with different samples. (f) Photocatalytic disinfection efficiency of Salmonella with AgVO3/g-C3N4 at different concentrations. (g) Bacteria colony growth in the presence of AgVO3/g-C3N4 with Salmonella. (h) Bacteria colony growth in the presence of AgVO3/g-C3N4 with Salmonella at different concentrations. The corresponding thermal images of AgVO3/g-C3N4 following irradiation for (i) 5 min and (j) 10 min. Bacteria colony growth with (k) AgVO3/g-C3N4 and (l) BiVO4/g-C3N4 in the dark. Reproduced from ref. 251 with permission from Elsevier, copyright 2017. | |
Wang et al. fabricated vanadate (AgVO3 and BiVO4) QD/g-C3N4 nanocomposites using urea251 (Fig. 11d). Due to the abundant production of ROS by the vanadate QDs and g-C3N4, the vanadate QDs/g-C3N4 composites exhibited high bactericidal efficiency, with 96% inactivation (AgVO3 QDs/g-C3N4) and 87% inactivation (BiVO4 QDs/g-C3N4) of Salmonella after only 10 min of light illumination (Fig. 11e and g). As shown in Fig. 11f and h, the photocatalytic disinfection efficiency increases with increasing photocatalyst concentration. Only 22% of Salmonella were killed with a AgVO3/g-C3N4 composite concentration of 0.5 mg mL−1. However, at the same period, the photocatalytic inactivation of Salmonella increased to 58% when the photocatalyst concentration reach 0.75 mg mL−1. It is apparent from Fig. 11i and j that there is no significant change in temperature during the antibacterial tests. Furthermore, the bacteria grew well on the LB plate, meaning that photocatalyst does not kill the Salmonella (Fig. 11k and l). Considering the simplicity of the synthetic process, the chemical durability and the sterilization results, vanadate QDs/g-C3N4 are ideal photocatalysts for applications in environmental settings.
3. Conclusions and perspectives
Materials based on g-C3N4 are promising photocatalysts with excellent physico-chemical properties and have considerable promise in antibacterial applications. Nevertheless, the antibacterial applications of bulk g-C3N4 are limited by its narrow absorption of visible light and facile recombination of charges. Consequently, a variety of g-C3N4-based nanocomposites have been developed with high superficial areas, improved e−/h+ separation efficiencies and expanded visible light absorption ranges, combining to enhance their antibacterial activity. In this review, we highlighted the main strategies used to amplify the photocatalytic efficiency of g-C3N4-based nanocomposites and their antimicrobial properties, including different topologies, noble metal decoration, non-noble metal doping and heterojunction construction. The enhancement mechanisms and synergistic effects of g-C3N4-based nanocomposites was also discussed. Although g-C3N4 is an ideal photocatalyst for the construction of nanocomposites for antibacterial applications, there are still some issues to be solved and opportunities for further research:
(1) The antibacterial mechanism of g-C3N4-based nanocomposites include destroying cell membranes and cell walls, producing endotoxins, causing protein mutations, interfering with protein synthesis and oxidizing organics. However, the role of each process in the antibacterial activity has not yet been clearly defined, suggesting that future research on the antibacterial mechanisms of g-C3N4-based nanocomposites would be meaningful.
(2) Although g-C3N4-based nanocomposites have been extensively studied, the photocatalytic properties are not always predictable, and the performance between g-C3N4 and co-composites is often found to be additive and not synergistic. Therefore, molecular models that allow better composite design would be useful.
(3) When constructing g-C3N4 heterojunctions, a single heterojunction has many limitations in terms of light absorption and e− separation. Thus, the construction of dual heterojunctions, such as dual Z-type or combined Z-type and type II heterojunctions could enhance the photocatalytic effect of g-C3N4 nanocomposites and is a key topic for future research and development.
(4) The antibacterial efficiency of g-C3N4-based nanocomposite photocatalysts relies largely on ultraviolet and blue light. Extending the range to longer wavelengths would be advantageous.
(5) Most studies were carried at laboratory scales and synthetic strategies for large-scale production are challenging. The development of simple and large-scale green and sustainable synthetic methods that can be automated are required to facilitate commercial applications.
(6) ROS are also able to destroy viruses and therefore further research exploring the antiviral properties of g-C3N4 nanocomposites would be valuable.
Conflicts of interest
There are no conflicts to declare.
Acknowledgements
This work was supported by the National Natural Science Foundation of China (51803112, 216740859), China Postdoctoral Science Foundation Grant (2018M633503), Key Laboratory Construction Program of Xi'an Municipal Bureau of Science and Technology (201805056ZD7CG40), and Innovation Capability Support Program of Shaanxi (No. 2018PT-28, 2019PT-05).
References
- R. Edwards and K. G. Harding, Curr. Opin. Infect. Dis., 2004, 17, 91–96 CrossRef PubMed.
- J. Bures, J. Cyrany, D. Kohoutova, M. Förstl, S. Rejchrt, J. Kvetina, V. Vorisek and M. Kopacova, World J. Gastroenterol., 2010, 16, 2978 CrossRef CAS PubMed.
- T. Wei, Q. Yu and H. Chen, Adv. Healthcare Mater., 2019, 8, 1801381 CrossRef CAS PubMed.
- Y. Elad, H. Yunis and T. Katan, Plant Pathol., 1992, 41, 41–46 CrossRef CAS.
- K. Su, L. Tan, X. M. Liu, Z. D. Cui, Y. F. Zheng, B. Li, Y. Han, Z. Y. Li, S. L. Zhu, Y. Q. Liang, X. B. Feng, X. B. Wang and S. L. Wu, ACS Nano, 2020, 14, 2077–2089 CrossRef CAS PubMed.
- X. Z. Xie, C. Y. Mao, X. M. Liu, Y. Z. Zhang, Z. D. Cui, X. J. Yang, K. W. K. Yeung, H. B. Pan, P. K. Chu and S. L. Wu, ACS Appl. Mater. Interfaces, 2017, 9, 26417–26428 CrossRef CAS PubMed.
- U. Tsutomu, Y. Tetsuya, T. Sigeru and A. Keisuke, Chem. Lett., 2003, 32, 330–331 CrossRef.
- L. H. Li, J. C. Deng, H. R. Deng, Z. L. Liu and X. L. Li, Chem. Eng. J., 2010, 160, 378–382 CrossRef CAS.
- F. B. Liu, S. C. Lai, H. J. Tong, P. S. J. Lakey, M. Shiraiwa, M. G. Weller, U. Poschl and C. J. Kampf, Anal. Bioanal. Chem., 2017, 409, 2411–2420 CrossRef CAS PubMed.
- Q. Li, T. Li, C. Liu, G. Deloid, G. Pyrgiotakis, P. Demokritou, R. Zhang, H. Xiao and D. J. Mcclements, Nanotoxicology, 2017, 11, 1–15 CrossRef CAS PubMed.
- K. Yan, Y. B. Zhang, C. L. Mu, Q. N. Xu, X. N. Jing, D. Q. Wang, D. F. Dang, L. J. Meng and J. Z. Ma, Theranostics, 2020, 10, 7287–7318 CrossRef CAS PubMed.
- X. N. Jing, Z. Zhi, N. Zhang, H. H. Song, Y. Z. Xu, G. Q. Zhou, D. Q. Wang, Y. P. Shao and L. J. Meng, Chem. Eng. J., 2020, 385, 123893 CrossRef CAS.
- J. S. Ni, T. L. Min, Y. X. Li, M. L. Zha, P. F. Zhang, C. L. Ho and K. Li, Angew. Chem., Int. Ed., 2020, 59, 10179–10185 CrossRef CAS PubMed.
- L. S. R. Yadav, K. Manjunath, C. Kavitha and G. Nagaraju, J. Sci.: Adv. Mater. Devices, 2018, 3, 181–187 Search PubMed.
- K. Ghule, A. V. Ghule, B. J. Chen and Y. C. Ling, Green Chem., 2006, 8, 1034–1041 RSC.
- C. Y. Mao, Y. M. Xiang, X. M. Liu, Z. D. Cui, X. J. Yang, Z. Y. Li, K. W. K. Yeung, H. Pan, X. B. Wang, P. K. Chu and S. L. Wu, ACS Nano, 2017, 11, 9010–9021 CrossRef CAS PubMed.
- F. He, G. Chen, Y. G. Yu, S. Hao, Y. S. Zhou and Y. Zheng, ACS Appl. Mater. Interfaces, 2014, 6, 7171–7179 CrossRef CAS PubMed.
- J. W. Fu, J. G. Yu, C. J. Jiang and B. Cheng, Adv. Energy Mater., 2018, 8, 1701503 CrossRef.
- J. Q. Wen, J. Xie, X. B. Chen and X. Li, Appl. Surf. Sci., 2017, 391, 72–123 CrossRef CAS.
- T. Xiong, W. L. Cen, Y. X. Zhang and F. Dong, ACS Catal., 2016, 6, 2462–2472 CrossRef CAS.
- Z. S. Chen, S. Zhang, Y. Liu, N. S. Alharbi, S. O. Rabah, S. H. Wang and X. X. Wang, Sci. Total Environ., 2020, 731, 139054 CrossRef CAS PubMed.
- S. Kumar, S. Karthikeyan and A. F. Lee, Catalysts, 2018, 8, 74 CrossRef.
- W. K. Darkwah and Y. H. Ao, Nanoscale Res. Lett., 2018, 13, 388 CrossRef PubMed.
- Y. Xu and S. P. Gao, Int. J. Hydrogen Energy, 2012, 37, 11072–11080 CrossRef CAS.
- C. Prasad, H. Tang, Q. Q. Liu, I. Bahadur, S. Karlapudi and Y. J. Jiang, Int. J. Hydrogen Energy, 2020, 45, 337–379 CrossRef CAS.
- X. Y. Lu, J. Xie, X. B. Chen and X. Li, Appl. Catal., B, 2019, 252, 250–259 CrossRef CAS.
- L. T. Ma, H. Q. Fan, K. Fu, S. H. Lei, Q. Z. Hu, H. T. Huang and G. P. He, ACS Sustainable Chem. Eng., 2017, 5, 7093–7103 CrossRef CAS.
- Y. Zhao, J. Zhang and L. T. Qu, ChemNanoMat, 2015, 1, 298–318 CrossRef CAS.
- A. Zambon, J. M. Mouesca, C. Gheorghiu, P. A. Bayle, J. Pécaut, M. C. Bruno, S. Gambarell and L. Dubois, Chem. Sci., 2015, 7, 945–950 RSC.
- X. C. Wang, K. Maeda, A. Thomas, K. Takanabe, G. Xin, J. M. Carlsson, K. Domen and M. Antonietti, Nat. Mater., 2009, 8, 76–80 CrossRef CAS PubMed.
- A. Fujishima and K. Honda, Nature, 1972, 238, 37–38 CrossRef CAS PubMed.
- L. M. Hu, J. T. Yan, C. L. Wang, B. Chai and J. F. Li, Chin. J. Catal., 2019, 40, 458–469 CrossRef CAS.
- X. Li, C. Y. Liu, D. Y. Wu, J. Z. Li, P. W. Huo and H. Q. Wang, Chin. J. Catal., 2019, 40, 928–939 CrossRef CAS.
- B. Chai, J. T. Yan, G. Z. Fan, G. S. Song and C. L. Wang, Chin. J. Catal., 2020, 41, 170–179 CrossRef CAS.
- Y. Li, X. Li, H. W. Zhang, J. J. Fan and Q. J. Xiang, J. Mater. Sci. Nanotechnol., 2020, 56, 69–88 CrossRef.
- D. Q. Gao, Q. Xu, J. Zhang, Z. L. Yang, M. S. Si, Z. J. Yan and D. S. Xue, Nanoscale, 2014, 6, 2577–2581 RSC.
- M. Groenewolt and M. Antonietti, Adv. Mater., 2005, 17, 1789–1792 CrossRef CAS.
- B. C. Zhu, P. F. Xia, W. K. Ho and J. G. Yu, Appl. Surf. Sci., 2015, 344, 188–195 CrossRef CAS.
- H. N. Che, C. B. Liu, G. B. Che, G. F. Liao, H. J. Dong, C. X. Li, N. Song and C. M. Li, Nano Energy, 2020, 67, 104273 CrossRef CAS.
- Z. Y. Wang, Y. Huang, M. J. Chen, X. J. Shi, Y. F. Zhang, J. J. Cao, W. K. Ho and S. C. Lee, ACS Appl. Mater. Interfaces, 2019, 11, 10651–10662 CrossRef CAS PubMed.
- Y. D. Zou, B. B. Yang, Y. Liu, Y. Ren, J. H. Ma, X. R. Zhou, X. W. Cheng and Y. H. Deng, Adv. Funct. Mater., 2018, 28, 1806214 CrossRef.
- Y. H. Li, M. L. Gu, M. Zhang, X. M. Zhang, K. L. Lv, W. K. Ho and F. Dong, Chem. Eng. J., 2020, 389, 124421 CrossRef CAS.
- Z. X. Sun, H. Q. Wang, Z. B. Wu and L. Z. Wang, Catal. Today, 2017, 300, 160–172 CrossRef.
- A. Nikokavoura and C. Trapalis, Appl. Surf. Sci., 2018, 430, 18–52 CrossRef CAS.
- F. He, Z. X. Wang, Y. X. Li, S. Q. Peng and B. Liu, Appl. Catal., B, 2020, 269, 118828 CrossRef CAS.
- I. Papailias, N. Todorova, T. Giannakopoulou, N. Ioannidis, P. Dallas, D. Dimotikali and C. Trapalis, Appl. Catal., B, 2020, 268, 118733 CrossRef CAS.
- D. D. Ren, Z. Z. Liang, Y. H. Ng, P. Zhang, Q. J. Xiang and X. Li, Chem. Eng. J., 2020, 390, 124496 CrossRef CAS.
- X. Li, J. Xie, C. J. Jiang, J. G. Yu and P. Y. Zhang, Front. Environ. Sci. Eng., 2018, 12, 14 CrossRef.
- H. C. Lan, L. L. Li, X. Q. An, F. Liu, C. B. Chen, H. J. Liu and J. H. Qu, Appl. Catal., B, 2017, 204, 49–57 CrossRef CAS.
- J. Y. Shan, K. L. Yang, W. J. Xiu, Q. Qiu, S. L. Dai, L. H. Yuwen, L. X. Weng, Z. G. Teng and L. H. Wang, Small, 2020, 16, 2001099 CrossRef CAS PubMed.
- C. C. Han, P. F. Su, B. H. Tan, X. G. Ma, H. Lv, C. Y. Huang, P. Wang, Z. F. Tong, G. Li, Y. Z. Huang and Z. F. Liu, J. Colloid Interface Sci., 2021, 581, 159–166 CrossRef CAS PubMed.
- R. C. Shen, J. Xie, X. Y. Lu, X. B. Chen and X. Li, ACS Sustainable Chem. Eng., 2018, 6, 4026–4036 CrossRef CAS.
- R. C. Shen, J. Xie, P. Y. Guo, L. S. Chen, X. B. Chen and X. Li, ACS Appl. Energy Mater., 2018, 1, 2232–2241 CrossRef CAS.
- L. L. Sun, C. Y. Liu, J. Z. Li, Y. J. Zhou, H. Q. Wang, P. W. Huo, C. C. Ma and Y. S. Yan, Chin. J. Catal., 2019, 40, 80–94 CrossRef CAS.
- R. C. Shen, Y. N. Ding, S. B. Li, P. Zhang, Q. J. Xiang, Y. H. Ng and X. Li, Chin. J. Catal., 2021, 42, 25–36 CrossRef CAS.
- X. J. Bai, L. Wang, R. L. Zong and Y. F. Zhu, J. Phys. Chem. C, 2013, 117, 9952–9961 CrossRef CAS.
- Z. X. Jiang, X. Zhang, H. S. Chen, X. Hu and P. Yang, ChemCatChem, 2019, 11, 4558–4567 CrossRef CAS.
- B. Chong, L. Chen, D. Z. Han, L. Wang, L. J. Feng, Q. Li, C. H. Li and W. T. Wang, Chin. J. Catal., 2019, 40, 959–968 CrossRef CAS.
- R. C. Shen, K. L. He, A. P. Zhang, N. Li, Y. H. Ng, P. Zhang, J. Hu and X. Li, Appl. Catal., B, 2021, 291, 120104 CrossRef CAS.
- R. Geng, J. J. Yin, J. X. Zhou, T. F. Jiao, Y. Feng, L. X. Zhang, Y. Chen, Z. H. Bai and Q. M. Peng, Nanomaterials, 2020, 10, 1 CrossRef CAS PubMed.
- X. Y. Chu, Y. Qu, A. Zada, L. L. Bai, Z. J. Li, F. Yang, L. N. Zhao, G. L. Zhang, X. J. Sun, Z. D. Yang and L. Q. Jing, Adv. Sci., 2020, 7, 2001543 CrossRef CAS PubMed.
- X. G. Ma, Y. H. Lv, J. Xu, Y. F. Liu, R. Q. Zhang and Y. F. Zhu, J. Phys. Chem. C, 2012, 116, 23485–23493 CrossRef CAS.
- J. W. Fu, B. C. Zhu, C. J. Jiang, B. Cheng, W. You and J. G. Yu, Small, 2017, 13, 1603938 CrossRef PubMed.
- S. C. Yan, Z. S. Li and Z. G. Zou, Langmuir, 2010, 26, 3894–3901 CrossRef CAS PubMed.
- Y. F. Li, M. H. Zhou, B. Cheng and Y. Shao, J. Mater. Sci. Nanotechnol., 2020, 56, 1–17 CrossRef.
- J. Y. Li, Z. Y. Zhang, W. Cui, H. Wang, W. L. Cen, G. Johnson, G. M. Jiang, S. Zhang and F. Dong, ACS Catal., 2018, 8, 8376–8385 CrossRef CAS.
- R. C. Shen, D. D. Ren, Y. N. Ding, Y. T. Guan, Y. H. Ng, P. Zhang and X. Li, Sci. China Mater., 2020, 63, 2153–2188 CrossRef CAS.
- S. Z. Butler, S. M. Hollen, L. Y. Cao, Y. Cui, J. A. Gupta, H. R. Gutiérrez, T. F. Heinz, S. Sa. Hong, J. X. Huang, A. F. Ismach, E. J. Halperin, M. Kuno, V. V. Plashnitsa, R. D. Robinson, R. S. Ruoff, S. Salahuddin, J. Shan, L. Shi, M. G. Spencer, M. Terrones, W. Windl and J. E. G. Cao, ACS Nano, 2013, 7, 2898–2926 CrossRef CAS PubMed.
- F. Dong, Z. Zhao, T. Xiong, Z. Ni, W. D. Zhang, Y. J. Sun and W. K. Ho, ACS Appl. Mater. Interfaces, 2013, 5, 11392–11401 CrossRef CAS PubMed.
- L. Sun, T. Du, C. Hu, J. Chen, J. Lu, Z. Lu and H. Han, ACS Sustainable Chem. Eng., 2017, 5, 8693–8701 CrossRef CAS.
- Y. Wang, X. C. Wang and M. Antonietti, Angew. Chem., Int. Ed., 2012, 51, 68–89 CrossRef CAS PubMed.
- W. J. Ong, L. L. Tan, Y. H. Ng, S. T. Yong and S. P. Chai, Chem. Rev., 2016, 116, 7159–7329 CrossRef CAS PubMed.
- J. Wang, G. H. Wang, B. Cheng, J. G. Yu and J. J. Fan, Chin. J. Catal., 2021, 42, 56–68 CrossRef CAS.
- Y. L. Liu, S. S. Wu, J. Liu, S. B. Xie and Y. J. Liu, RSC Adv., 2021, 11, 4810–4817 RSC.
- D. D. Ren, W. N. Zhang, Y. N. Ding, R. C. Shen, Z. M. Jiang, X. Y. Lu and X. Li, Sol. RRL, 2020, 4, 1900423 CrossRef CAS.
- M. Tahir, C. B. Cao, F. K. Butt, F. Idrees, N. Mahmood, Z. Ali, I. Aslam, M. Tanveer, M. Rizwan and T. Mahmood, J. Mater. Chem. A, 2013, 1, 13949–13955 RSC.
- Z. W. Tong, D. Yang, Y. Y. Sun, Y. H. Nan and Z. Y. Jiang, Small, 2016, 12, 4093–4101 CrossRef CAS PubMed.
- J. Y. Li, Z. Y. Zhang, W. Cui, H. Wang, W. L. Cen, G. Johnson, G. M. Jiang, S. Zhang and F. Dong, ACS Catal., 2018, 8, 8376–8385 CrossRef CAS.
- J. D. Hong, S. M. Yin, Y. X. Pan, J. Y. Han, T. H. Zhou and R. Xu, Nanoscale, 2014, 6, 14984–14990 RSC.
- Q. H. Liang, Z. Li, Z. H. Huang, F. Kang and Q. H. Yang, Adv. Funct. Mater., 2015, 25, 6885–6892 CrossRef CAS.
- Y. Jian, D. M. Wang, M. Z. Huang, H. L. Jia, J. H. Sun, X. K. Song and M. G. Guan, ACS Sustainable Chem. Eng., 2017, 5, 6827–6834 CrossRef CAS.
- R. Li, Y. L. Ren, P. X. Zhao, J. Wang, J. D. Liu and Y. T. Zhang, J. Hazard. Mater., 2019, 365, 606–614 CrossRef CAS PubMed.
- C. L. Fu, L. J. Meng, Q. H. Lu, Z. F. Fei and P. J. Dason, Adv. Funct. Mater., 2008, 18, 857 CrossRef CAS.
- Q. Li, J. P. Yang, D. Feng, Z. X. Wu, Q. L. Wu, S. S. Park, C. S. Ha and D. Y. Zhao, Nano Res., 2010, 3, 632–642 CrossRef CAS.
- J. Xu, Z. P. Wang and Y. F. Zhu, J. Mater. Sci. Nanotechnol., 2020, 49, 133–143 CrossRef.
- Z. W. Tong, D. Yang, Z. Li, Y. H. Nan, F. Ding, Y. C. Shen and Z. Y. Jiang, ACS Nano, 2017, 11, 1103–1112 CrossRef CAS PubMed.
- F. Yang, G. Y. Ding, J. Wang, Z. H. Liang, B. Gao, M. M. Dou, C. Xu and S. M. Li, J. Membr. Sci., 2020, 606, 118146 CrossRef CAS.
- I. Kohsari, Z. Shariatinia and S. M. Pourmortazavi, Int. J. Biol. Macromol., 2016, 91, 778–788 CrossRef CAS PubMed.
- J. Wang, Y. M. Wang, Y. T. Zhang, A. Uliana, J. Y. Zhu, J. D. Liu and B. V. Bruggen, ACS Appl. Mater. Interfaces, 2016, 8, 25508–25519 CrossRef CAS PubMed.
- S. F. Kang, L. Zhang, M. F. He, Y. Y. Zheng, L. F. Cui, Di Sun and B. Hu, Carbon, 2018, 137, 19–30 CrossRef CAS.
- J. Xu, Z. P. Wang and Y. F. Zhu, ACS Appl. Mater. Interfaces, 2017, 9, 27727–27735 CrossRef CAS PubMed.
- S. F. Kang, W. Huang, L. Zhang, M. F. He, S. Y. Xu, D. Sun and X. Jiang, ACS Appl. Mater. Interfaces, 2018, 10, 13796–13804 CrossRef CAS PubMed.
- J. H. Thurston, N. M. Hunter, L. J. Wayment and K. A. Cornell, J. Colloid Interface Sci., 2017, 505, 910–918 CrossRef CAS PubMed.
- N. N. He, S. H. Cao, L. H. Zhang, Z. D. Tian, H. Chen and F. J. Jiang, Chemosphere, 2019, 235, 1116–1124 CrossRef CAS PubMed.
- Z. Y. Teng, N. L. Yang, H. Y. Lv, S. C. Wang, M. Z. Hu, C. Y. Wang, D. Wang and G. X. Wang, Chem, 2019, 5, 664–680 CAS.
- Y. Li, C. Zhang, D. M. Shuai, S. Naraginti, D. W. Wang and W. L. Zhang, Water Res., 2016, 106, 249–258 CrossRef CAS PubMed.
- J. Q. Chen, W. T. Lin, L. Y. Xie, J. H. Huang and W. J Wang, J. Nanomater., 2019, 2019, 1–9 Search PubMed.
- P. Yadav, S. T. Nishanthi, B. Purohit, A. Shanavas and K. Kailasam, Carbon, 2019, 152, 587–597 CrossRef CAS.
- Y. Li, Y. N. Li, S. L. Ma, P. F. Wang, Q. L. Hou, J. J. Han and S. H. Zhan, J. Hazard. Mater., 2017, 338, 33–46 CrossRef CAS PubMed.
- J. Xu, J. Huang, Z. P. Wang and Y. F. Zhu, Chin. J. Catal., 2020, 41, 474–484 CrossRef CAS.
- H. F. Li, M. N. Ding, L. L. Luo, G. X. Yang, F. Y. Shi and Y. N. Huo, ChemCatChem, 2020, 12, 1334–1340 CrossRef CAS.
- N. Jiang, H. C. Geng, Y. Qiao, X. Q. Zhu, C. Y. Li and Q. Y. Cai, New J. Chem., 2020, 44, 2303–2311 RSC.
- F. L. Wang, Y. P. Feng, P. Chen, Y. F. Wang, Y. H. Su, Q. X. Zhang, Y. Q. Zeng, Z. J. Xie, H. J. Liu, Y. Liu, W. Y. Lv and G. G. Liu, Appl. Catal., B, 2018, 227, 114–122 CrossRef CAS.
- G. X. Song, L. W. Wang, R. Q. Yang, Y. C. Ji, R. T. Zhang, L. Yang, L. H. Ding, A. Z. Wang, N. Ren and X. Yu, J. Nanosci. Nanotechnol., 2020, 20, 5944–5950 CrossRef CAS PubMed.
- N. S. Heo, S. Shukla, S. Y. Oh, V. K. Bajpai, S. U. Lee, H. J. Cho, S. Kim, Y. Kim, H. J. Kim, S. Y. Lee, Y. S. Jun, M. H. Oh, Y. K. Han, S. M. Yoo and Y. S. Huh, Mater. Sci. Eng., 2019, 104, 109846 CrossRef CAS PubMed.
- J. H. Thurston, N. M. Hunter and K. A. Cornell, RSC Adv., 2016, 6, 42240–42248 RSC.
- A. Mirzaei, Z. Chen, F. Haghighat and L. Yerushalmi, Appl. Catal., B, 2019, 242, 337–348 CrossRef CAS.
- H. C. Shen, E. A. López-Guerra, R. C. Zhu, T. Diba, Q. M. Zheng, S. D. Solares, J. M. Zara, D. M. Shuai and Y. Shen, ACS Appl. Mater. Interfaces, 2019, 11, 373–384 CrossRef CAS PubMed.
- S. F. Kang, Y. Fang, Y. K. Huang, L. F. Cui, Y. Z. Wang, H. F. Qin, Y. M. Zhang, X. Li and Y. G. Wang, Appl. Catal., B, 2015, 168, 472–482 CrossRef.
- S. Patnaik, D. P. Sahoo and K. Parida, Renewable Sustainable Energy Rev., 2018, 82, 1297–1312 CrossRef CAS.
- F. K. Meng, J. T. Li, S. K. Cushing, J. Bright, M. J. Zhi, J. D. Rowley, Z. L. Hong, A. Manivannan, A. D. Bristow and N. Q. Wu, ACS Catal., 2013, 3, 746–751 CrossRef CAS.
- L. J. Meng, W. J. Xia, L. Liu, L. Y. Niu and Q. H. Lu, ACS Appl. Mater. Interfaces, 2014, 6, 4989–4996 CrossRef CAS PubMed.
- J. G. Mcevoy and Z. S. Zhang, J. Photochem. Photobiol., C, 2014, 19, 62–75 CrossRef.
- D. B. Ingram, P. Christopher, J. L. Bauer and S. Linic, ACS Catal., 2011, 1, 1441–1447 CrossRef CAS.
- Q. Q. Ding, R. Li, M. D. Chen and M. T. Sun, Appl. Mater. Today, 2017, 9, 251–258 CrossRef.
- S. H. Wang, J. W. Zhan, K. Chen, A. Ali, L. H. Zeng, H. Zhao, W. L. Hu, L. X. Zhu and X. L. Xu, ACS Sustainable Chem. Eng., 2020, 8, 8214–8222 CrossRef CAS.
- K. H. Sun, J. Shen, Q. Q. Liu, H. Tang, M. Y. Zhang, S. Zulfiqar and C. S. Lei, Chin. J. Catal., 2020, 41, 72–81 CrossRef CAS.
- Z. Q. Ren, F. Y. Chen, K. X. Wen and J. F. Lu, J. Photochem. Photobiol., A, 2020, 389, 112217 CrossRef CAS.
- S. L. Ma, S. H. Zhan, Y. N. Jia, Q. Shi and Q. X. Zhou, Appl. Catal., B, 2016, 186, 77–87 CrossRef CAS.
- X. J. Bai, R. L. Zong, C. X. Li, D. Liu, Y. F. Liu and Y. F. Zhu, Appl. Catal., B, 2014, 147, 82–91 CrossRef CAS.
- L. J. Meng, L. Y. Niu, L. Li, Q. H. Lu, Z. F. Fei and P. J. Dyson, Chem.–Eur. J., 2012, 18, 13314–13319 CrossRef CAS PubMed.
- D. Q. Wang, L. J. Meng, Z. F. Fei, C. Hou, J. G. Long, L. L. Zeng, P. J. Dyson and P. Huang, Nanoscale, 2018, 10, 8536–8546 RSC.
- J. Y. Dai, J. B. Song, Y. Qiu, J. J. Wei, Z. Z. Hong, L. Li and H. H. Yang, ACS Appl. Mater. Interfaces, 2019, 11, 10589–10596 CrossRef CAS PubMed.
- V. Shanmugam, S. Selvakumar and C. S. Yeh, Chem. Soc. Rev., 2014, 43, 6254–6287 RSC.
- E. Atilla, S. K. Toprak and T. Demirer, Turk. J. Haematol., 2017, 34, 1–9 CrossRef PubMed.
- Z. L. Jin and L. J. Zhang, J. Mater. Sci. Nanotechnol., 2020, 49, 144–156 CrossRef.
- Z. Z. Liang, R. C. Shen, Y. H. Ng, P. Zhang, Q. J. Xiang and X. Li, J. Mater. Sci. Nanotechnol., 2020, 56, 89–121 CrossRef.
- Y. T. Gao, F. Chen, Z. Chen and H. F. Shi, J. Mater. Sci. Nanotechnol., 2020, 56, 227–235 CrossRef.
- K. Wang, Q. Li, B. S. Liu, B. Cheng, W. K. Ho and J. G. Yu, Appl. Catal., B, 2015, 176, 44–52 Search PubMed.
- X. X. Zhao, J. R. Guan, J. Z. Li, X. Li, H. Q. Wang, P. W. Huo and Y. S. Yan, Appl. Surf. Sci., 2021, 537, 147891 CrossRef CAS.
- H. N. Che, G. B. Che, P. J. Zhou, C. B. Liu, H. J. Dong, C. X. Li, N. Song and C. M. Li, Chem. Eng. J., 2020, 382, 122870 CrossRef CAS.
- M. D. Capobianco, B. Pattengale, J. Neu and C. A. Schmuttenmaer, J. Phys. Chem. Lett., 2020, 11, 8873–8879 CrossRef CAS PubMed.
- X. Y. Lu, J. Xie, S. Y. Liu, A. Adamski, X. B. Chen and X. Li, ACS Sustainable Chem. Eng., 2018, 6, 13140–13150 CrossRef CAS.
- R. C. Shen, J. Xie, Q. J. Xiang, X. B. Chen, J. Z. Jiang and X. Li, Chin. J. Catal., 2019, 40, 240–288 CrossRef CAS.
- Y. P. Zhu, T. Z. Ren and Z. Y. Yuan, ACS Appl. Mater. Interfaces, 2015, 7, 16850–16856 CrossRef CAS PubMed.
- G. Liu, P. Niu, C. H. Sun, S. C. Smith, Z. G. Chen, G. Q. Lu and H. M. Cheng, J. Am. Chem. Soc., 2010, 132, 11642–11648 CrossRef CAS PubMed.
- J. W. Fang, H. Q. Fan, M. M. Li and C. B. Long, J. Mater. Chem. A, 2015, 3, 13819–13826 RSC.
- N. Güy, Appl. Surf. Sci., 2020, 522, 146442 CrossRef.
- Y. Wang, Y. Di, M. Antonietti, H. R. Li, X. F. Chen and X. C. Wang, Chem. Mater., 2010, 22, 5119–5121 CrossRef CAS.
- C. Zhou, R. Shi, L. Shang, L. Z. Wu, C. H. Tung and T. R. Zhang, Nano Res., 2018, 11, 3462–3468 CrossRef CAS.
- R. C. Shen, C. J. Jiang, Q. J. Xiang, J. Xie and X. Li, Appl. Surf. Sci., 2019, 471, 43–87 CrossRef CAS.
- Y. J. Li, L. Ding, Y. C. Guo, Z. Q. Liang, H. Z. Cui and J. Tian, ACS Appl. Mater. Interfaces, 2019, 11, 41440–41447 CrossRef CAS PubMed.
- C. L. Fu, L. J. Meng, Q. H. Lu, X. K. Zhang and C. Gao, Macromol. Rapid Commun., 2007, 28, 2180–2184 CrossRef CAS.
- R. Xia, Z. F. Fei, N. Drigo, F. D. Bobbink, Z. J. Huang, R. Jasiunas, M. Franckevicius, V. Gulbinas, M. Mensi, X. D. Fang, C. R. Carmona, M. K. Nazeeruddin and P. J. Dyson, Adv. Funct. Mater., 2019, 29, 1902021 CrossRef.
- J. T. Gao, Y. Wang, S. J. Zhou, W. Lin and Y. Kong, ChemCatChem, 2017, 9, 1708–1715 CrossRef CAS.
- J. Q. Wen, J. Xie, H. D. Zhang, A. P. Zhang, Y. J. Liu, X. B. Chen and X. Li, ACS Appl. Mater. Interfaces, 2017, 9, 14031–14042 CrossRef CAS PubMed.
- H. Tang, R. Wang, C. X. Zhao, Z. P. Chen, X. F. Yang, D. Bukhvalov, Z. X. Lin and Q. Q. Liu, Chem. Eng. J., 2019, 374, 1064–1075 CrossRef CAS.
- J. J. Feng, D. K. Zhang, H. P. Zhou, M. Y. Pi, X. D. Wang and S. J. Chen, ACS Sustainable Chem. Eng., 2018, 6, 6342–6349 CrossRef CAS.
- P. Sun, H. Liu, M. B. Feng, Z. C. Zhai, Y. S. Fang, X. S. Zhang and V. K. Sharma, Appl. Catal., B, 2020, 272, 119005 CrossRef CAS.
- L. J. Meng, C. L. Fu, Z. F. Fei, Q. H. Lu and P. J. Dyson, Inorg. Chim. Acta, 2010, 363, 3926–3931 CrossRef CAS.
- J. Ran, T. Pan, Y. Y. Wu, C. Q. Chu, P. Cui, P. P. Zhang, X. Y. Ai, C. F. Fu, Z. J. Yang and T. W. Xu, Angew. Chem., Int. Ed., 2019, 58, 16463–16468 CrossRef CAS PubMed.
- X. D. Du, X. H. Yi, P. Wang, J. G. Deng and C. C. Wang, Chin. J. Catal., 2019, 40, 70–79 CrossRef.
- M. F. R. Samsudin, H. Ullah, A. A. Tahir, X. H. Li, Y. H. Ng and S. Sufian, J. Colloid Interface Sci., 2021, 586, 785–796 CrossRef CAS PubMed.
- Q. Yang, S. W. Yu, P. Fu, W. Yu, Y. Liu, X. Liu, Z. C. Feng, X. Guo and C. Li, Adv. Funct. Mater., 2020, 30, 1910205 CrossRef CAS.
- X. B. Li, J. Xiong, Y. Xu, Z. J. Feng and J. T. Huang, Chin. J. Catal., 2019, 40, 424–433 CrossRef CAS.
- C. Liu, Y. Feng, Z. T. Han, Y. Sun, X. Q. Wang, Q. F. Zhang and Z. G. Zou, Chin. J. Catal., 2021, 42, 164–174 CrossRef CAS.
- H. G. Yu, J. C. Xu, D. D. Gao, J. J. Fan and J. G. Yu, Sci. China Mater., 2020, 63, 2215–2227 CrossRef CAS.
- Y. Li, X. M. Liu, L. Tan, Z. D. Cui, X. J. Yang, Y. F. Zheng, K. W. K. Yeung, P. K. Chu and S. L. Wu, Adv. Funct. Mater., 2018, 28, 1800299 CrossRef.
- H. Guo, C. G. Niu, Y. Y. Yang, C. Liang, H. Y. Niu, H. Y. Liu, L. Li and N. Tang, Chem. Eng. J., 2021, 422, 130029 CrossRef CAS.
- P. G. Sionnest, Microchim. Acta, 2008, 160, 309–314 CrossRef.
- X. Q. Wu, J. Zhao, S. J. Guo, L. P. Wang, W. L. Shi, H. Huang, Y. Liu and Z. H. Kang, Nanoscale, 2016, 8, 17314–17321 RSC.
- X. J. Yu, J. J. Liu, Y. C. Yu, S. L. Zuo and B. S. Li, Carbon, 2014, 68, 718–724 CrossRef CAS.
- H. J. Feng, Q. Q. Guo, Y. F. Xu, T. Chen, Y. Y. Zhou, Y. G. Wang, M. Z. Wang and D. S. Shen, Chemsuschem, 2018, 11, 4256–4261 CrossRef CAS PubMed.
- Y. Y. Li, Y. Fang, Z. L. Cao, N. J. Li, D. Y. Chen, Q. F. Xu and J. M. Lu, Appl. Catal., B, 2019, 250, 150–162 CrossRef CAS.
- C. Y. Tang, C. Liu, Y. Han, Q. Q. Guo, W. Ouyang, H. J. Feng, M. Z. Wang and F. Xu, Adv. Healthcare Mater., 2019, 8, 1801534 CrossRef PubMed.
- M. J. M. Batista, O. F. Carceller, M. Ferrer, M. F. García and A. Kubacka, Appl. Catal., B, 2016, 183, 86–95 CrossRef.
- P. She, J. Li, H. G. Bao, X. Q. Xu and Z. Hong, J. Photochem. Photobiol., A, 2019, 384, 112028 CrossRef CAS.
- Y. Y. Wu, Y. Z. Zhou, H. Xu, Q. Q. Liu, Y. Li, L. L. Zhang, H. Q. Liu, Z. G. Tu, X. N. Cheng and J. Yang, ACS Sustainable Chem. Eng., 2018, 6, 14082–14094 CrossRef CAS.
- J. Xu, Q. Z. Gao, X. J. Bai, Z. P. Wang and Y. F. Zhu, Catal. Today, 2019, 332, 227–235 CrossRef CAS.
- Z. Z. Wang, K. Dong, Z. Liu, Y. Zhang, Z. W. Chen, H. J. Sun, J. S. Ren and X. G. Qu, Biomaterials, 2017, 113, 145–157 CrossRef CAS PubMed.
- K. Ouyang, K. Dai, H. Chen, Q. Y. Huang, C. H. Gao and P. Cai, Ecotoxicol. Environ. Saf., 2017, 136, 40–45 CrossRef CAS PubMed.
- W. J. Wang, Z. T. Zeng, G. M. Zeng, C. Zhang, R. Xiao, C. Y. Zhou, W. P. Xiong, Y. Yang, L. Lei, Y. Liu, D. L. Huang, M. Cheng, Y. Y. Yang, Y. K. Fu, H. Z. Luo and Y. Zhou, Chem. Eng. J., 2019, 378, 122132 CrossRef CAS.
- X. Q. Xu, S. M. Wang, X. F. Yu, J. L. Dawa, D. L. Gui and R. H. Tang, Appl. Surf. Sci., 2020, 501, 144245 CrossRef CAS.
- M. E. Khan, T. H. Han, M. M. Khan, M. R. Karim and M. H. Cho, ACS Appl. Nano Mater., 2018, 1, 2912–2922 CrossRef CAS.
- C. C. Liu, L. Wang, H. Xu, S. Wang, S. M. Gao, X. C. Ji, Q. Xu and W. Lan, Mater. Lett., 2016, 164, 567–570 CrossRef CAS.
- H. D. Zhang, X. Zhang, M. H. Zhu, H. Y. Li, Y. Zhao, X. R. Han, L. H. Jin and H. X. Shan, ChemPlusChem, 2020, 85, 2722–2730 CrossRef CAS PubMed.
- Z. Y. Liu, M. Y. Zhang and J. L. Wu, ChemistrySelect, 2018, 3, 10630–10636 CrossRef CAS.
- X. B. Chen, S. H. Shen, L. J. Guo and S. S. Mao, Chem. Rev., 2010, 110, 6503–6570 CrossRef CAS PubMed.
- S. I. Sadovnikov and A. I. Gusev, J. Mater. Chem. A, 2017, 5, 17676–17704 RSC.
- Y. X. Yan, H. Yang, Z. Yi, R. S. Li and T. Xian, Solid State Sci., 2020, 100, 106102 CrossRef CAS.
- N. N. Yuan, J. F. Zhang, S. J. Zhang, G. L. Chen, S. G. Meng, Y. Fan, X. Z. Zheng and S. F. Chen, J. Phys. Chem. C, 2020, 124, 8561–8575 CrossRef CAS.
- Y. Y. Wang, H. L. Huang, Z. Z. Zhang, C. Wang, Y. Y. Yang, Q. Li and D. S. Xu, Appl. Catal., B, 2021, 282, 119570 CrossRef CAS.
- C. J. Wang, Y. L. Zhao, H. Xu, Y. F. Li, Y. C. Wei, J. Liu and Z. Zhao, Appl. Catal., B, 2020, 263, 118314 CrossRef CAS.
- E. V. Kermani, A. H. Yangjeh, H. D. Khalilabad and S. Ghosh, J. Colloid Interface Sci., 2020, 563, 81–91 CrossRef PubMed.
- Y. Li, X. M. Liu, L. Tan, Z. D. Cui, D. D. Jing, X. J. Yang, Y. Q. Liang, Z. Y. Li, S. L. Zhu, Y. F. Zheng, K. W. K. Yeung, D. Zheng, X. B. Wang and S. L. Wu, Adv. Funct. Mater., 2019, 29, 1900946 CrossRef.
- W. J. Wang, G. Y. Li, T. C. An, D. K. L. Chan, J. C. Yu and P. K. Wong, Appl. Catal., B, 2018, 238, 126–135 CrossRef CAS.
- Y. P. Yuan, L. S. Yin, S. W. Cao, L. N. Gu, G. S. Xu, P. W. Du, H. Chai, Y. S. Liao and C. Xue, Green Chem., 2014, 16, 4663–4668 RSC.
- L. Jing, R. X. Zhu, D. L. Phillips and J. C. Yu, Adv. Funct. Mater., 2017, 46, 1703484 CrossRef.
- R. S. Das, S. K. Warkhade, A. Kumar, G. S. Gaikwad and A. V. Wankhade, J. Alloys Compd., 2020, 846, 155770 CrossRef CAS.
- I. Khan, N. Baig and A. Qurashi, ACS Appl. Energy Mater., 2018, 2, 607–615 CrossRef.
- Y. Tian, F. Zhou, S. Zhan, Z. Y. Zhu and Q. C. He, J. Photochem. Photobiol., A, 2018, 350, 10–16 CrossRef CAS.
- L. Y. Lu, G. H. Wang, M. Zou, J. Wang and J. Li, Appl. Surf. Sci., 2018, 441, 1012–1023 CrossRef CAS.
- Z. F. Huang, J. J. Song, X. Wang, L. Pan, K. Li, X. W. Zhang, L. Wang and J. J. Zou, Nano Energy, 2017, 40, 308–316 CrossRef CAS.
- J. Wang, G. H. Wang, X. H. Wei, G. Liu and J. Li, Appl. Surf. Sci., 2018, 456, 666–675 CrossRef CAS.
- R. R. Hao, G. H. Wang, C. J. Jiang, H. Tang and Q. C. Xu, Appl. Surf. Sci., 2017, 411, 400–410 CrossRef CAS.
- Q. Z. Gao, J. Xu, Z. P. Wang and Y. F. Zhu, Appl. Catal., B, 2020, 15, 118933 CrossRef.
- F. Chen, C. G. Niu, Q. Yang, X. M. Li and G. M. Zeng, Ceram. Int., 2016, 42, 2515–2525 CrossRef CAS.
- L. W. Zhang, T. G. Xu, X. Zhao and Y. F. Zhu, Appl. Catal., B, 2010, 98, 138–146 CrossRef CAS.
- J. Li, Y. C. Yin, E. Z. Liu, Y. N. Ma, J. Wan, J. Fan and X. Y. Hu, J. Hazard. Mater., 2017, 321, 183–192 CrossRef CAS.
- M. L. Tang, Y. H. Ao, C. Wang and P. F. Wang, Appl. Catal., B, 2020, 268, 118395 CrossRef CAS.
- P. F. Chen, L. Chen, S. F. Ge, W. Q. Zhang, M. F. Wu, P. X. Xing, T. B. Rotamond, H. J. Lin, Y. Wu and Y. M. He, Int. J. Hydrogen Energy, 2020, 45, 14354–14367 CrossRef CAS.
- S. Panimalar, R. Uthrakumar, E. T. Selvi, P. Gomathy, C. Inmozhi, K. Kaviyarasu and J. Kennedy, Surf. Interfaces, 2020, 20, 100512 CrossRef CAS.
- Q. L. Xu, B. C. Zhu, C. J. Jiang, B. Cheng and J. G. Yu, Sol. RRL, 2018, 2, 1800006 CrossRef.
- P. F. Xia, B. C. Zhu, B. Cheng, J. G. Yu and J. S. Xu, ACS Sustainable Chem. Eng., 2018, 6, 965–973 CrossRef CAS.
- R. Millaleo, M. R. Diaz, A. G. Ivanov, M. L. Mora and M. Alberdi, J. Soil Sci. Plant Nutr., 2010, 10, 470–481 CrossRef.
- B. Nanda, A. C. Pradhan and K. M. Parida, Microporous Mesoporous Mater., 2016, 226, 229–242 CrossRef CAS.
- Z. Y. Ma, Y. Sun, J. W. Xie, P. P. Li, Q. J. Lu, M. L. Liu, P. Yin, H. T. Li, Y. Y. Zhang and S. Z. Yao, ACS Appl. Mater. Interfaces, 2020, 12, 15919–15927 CrossRef CAS PubMed.
- H. H. Zeng, B. L. Xing, C. T. Zhang, L. J. Chen, H. H. Zhao, X. F. Han, G. Y. Yi, G. X. Huang, C. X. Zhang and Y. J. Cao, Energy Fuels, 2020, 34, 2480–2491 CrossRef CAS.
- X. Gao, H. W. Wu, W. J. Li, Y. Tian, Y. Zhang, H. Wu, L. Yang, G. Q. Zou, H. S. Hou and X. B. Ji, Small, 2020, 16, 1905842 CrossRef CAS PubMed.
- Z. H. Huang, Y. Song, D. Y. Feng, Z. Sun, X. Q. Sun and X. X. Liu, ACS Nano, 2018, 12, 3557–3567 CrossRef CAS.
- F. F. Wu, X. B. Gao, X. L. Xu, Y. N. Jiang, X. L. Gao, R. L. Yin, W. H. Shi, W. X. Liu, G. Lu and X. H. Cao, ChemSusChem, 2020, 13, 1537–1545 CrossRef CAS PubMed.
- B. B. Wu, Y. Li, K. Su, L. Tan, X. M. Liu, Z. D. Cui, X. J. Yang, Y. Q. Liang, Z. Y. Li, S. L. Zhu, K. W. K. Yeung and L. S. Wu, J. Hazard. Mater., 2019, 377, 227–236 CrossRef CAS PubMed.
- L. M. Sun, X. Zhao, C. J. Jia, Y. X. Zhou, X. F. Cheng, P. Li, L. Liu and W. L. Fan, J. Mater. Chem., 2012, 22, 23428–23438 RSC.
- C. Q. Li, Z. M. Sun, Y. L. Xue, G. Y. Yao and S. L. Zheng, Adv. Powder Technol., 2016, 27, 330–337 CrossRef CAS.
- T. W. Wu, H. T. Zhao, X. J. Zhu, Z. Xing, Q. Liu, T. Liu, S. Y. Gao, S. Y. Lu, G. Chen, A. M. Asiri, Y. N. Zhang and X. P. Sun, Adv. Mater., 2020, 32, 2000299 CrossRef CAS PubMed.
- C. J. Shuai, B. Wang, S. Z. Bin, S. P. Peng and C. D. Gao, ACS Appl. Mater. Interfaces, 2020, 12, 23464–23473 CrossRef CAS PubMed.
- S. Hejazi, S. Mohajernia, B. Osuagwu, G. Zoppellaro, P. Andryskova, O. Tomanec, S. Kment, R. Zbořil and P. Schmuki, Adv. Mater., 2020, 32, 1908505 CrossRef CAS PubMed.
- L. X. Zheng, F. Teng, X. Y. Ye, H. J. Zheng and X. S. Fang, Adv. Energy Mater., 2020, 10, 1902355 CrossRef CAS.
- M. Xiao, L. Zhang, B. Luo, M. Q. Lyu, Z. L. Wang, H. M. Huang, S. C. Wang, A. J. Du and L. Z. Wang, Angew. Chem., Int. Ed., 2020, 132, 7297–7301 CrossRef.
- C. Q. Li, Z. M. Sun, W. Z. Zhang, C. H. Yu and S. L. Zheng, Appl. Catal., B, 2017, 220, 272–282 CrossRef.
- L. T. Ma, H. Q. Fan, K. Fu and Y. W. Zhao, ChemistrySelect, 2016, 1, 3730–3738 CrossRef CAS.
- D. H. Xia, W. J. Wang, R. Yin, Z. F. Jiang, T. C. An, G. Y. Li, H. J. Zhao and P. K. Wong, Appl. Catal., B, 2017, 214, 23–33 CrossRef CAS.
- Z. Y. Zhu, F. Zhou and S. Zhan, Appl. Surf. Sci., 2019, 506, 144934 CrossRef.
- W. L. Shi, C. Liu, M. Y. Li, X. Lin, F. Guo and J. Y. Shi, J. Hazard. Mater., 2020, 389, 121907 CrossRef CAS PubMed.
- X. K. Zeng, S. Y. Lan and I. M. C. Lo, Environ. Sci.: Nano, 2019, 6, 610–623 RSC.
- S. L. Ma, S. H. Zhan, Y. G. Xia, P. F. Wang, Q. L. Hou and Q. X. Zhou, Catal. Today, 2019, 330, 179–188 CrossRef CAS.
- Y. Huo, Z. L. Wang, J. F. Zhang, C. H. Liang and K. Dai, Appl. Surf. Sci., 2018, 459, 271–280 CrossRef CAS.
- M. Mitsushio, K. Miyashita and M. Higo, Sens. Actuators, A, 2006, 125, 296–303 CrossRef CAS.
- Z. J. Zhang, W. Z. Wang, E. Gao, S. M. Sun and L. Zhang, J. Phys. Chem. C, 2012, 116, 25898–25903 CrossRef CAS.
- F. F. Mei, K. Dai, J. F. Zhang, W. Y. Li and C. H. Liang, Appl. Surf. Sci., 2019, 488, 151–160 CrossRef CAS.
- K. H. Leong, B. L. Gan, S. Ibrahim and P. Saravanan, Appl. Surf. Sci., 2014, 319, 128–135 CrossRef CAS.
- Z. Y. Zhang, C. L. Shao, X. H. Li, C. H. Wang, M. Y. Zhang and Y. C. Liu, ACS Appl. Mater. Interfaces, 2010, 2, 2915–2923 CrossRef CAS PubMed.
- J. Hu, Z. X. Zhong, F. Zhang, W. H. Xing, W. Q. Jin and N. P. Xu, Ind. Eng. Chem. Res., 2016, 55, 6661–6670 CrossRef CAS.
- Y. H. Ao, K. D. Wang, P. F. Wang, C. Wang and J. Hou, Appl. Catal., B, 2016, 194, 157–168 CrossRef CAS.
- T. S. Sreena, P. P. Rao, A. K. V. Raj and T. R. A. Thara, J. Alloys Compd., 2018, 751, 148–158 CrossRef CAS.
- F. Schuster, B. Laumer, R. R. Zamani, C. Magén, J. R. Morante, J. Arbiol and M. Stutzmann, ACS Nano, 2014, 8, 4376–4384 CrossRef CAS PubMed.
- W. L. Shi, M. Y. Li, X. L. Huang, H. J. Ren, F. Guo, Y. B. Tang and C. Y. Lu, Chem. Eng. J., 2020, 394, 125009 CrossRef CAS.
- H. Y. Xing, L. E, Z. G. Guo, D. Zhao and Z. F. Liu, Chem. Eng. J., 2020, 394, 124907 CrossRef CAS.
- Z. L. Jin, X. Yan and X. Q. Hao, J. Colloid Interface Sci., 2020, 569, 34–49 CrossRef CAS PubMed.
- Z. Z. Ai, K. Zhang, B. Chang, Y. L. Shao, L. Zhang, Y. Z. Wu and X. P. Hao, Chem. Eng. J., 2020, 383, 123130 CrossRef CAS.
- A. H. Yangjeh, M. Pirhashemi and S. Ghosh, J. Alloys Compd., 2020, 826, 154229 CrossRef.
- C. H. Shen, X. J. Wen, Z. H. Fei, Z. T. Liu and Q. M. Mu, Chem. Eng. J., 2020, 391, 123612 CrossRef CAS.
- H. X. Shi, J. Fan, Y. Y. Zhao, X. Y. Hu, X. Zhang and Z. S. Tang, J. Hazard. Mater., 2020, 381, 121006 CrossRef CAS PubMed.
- C. J. Zhang, W. H. Fei, H. Q. Wang, N. J. Li, D. Y. Chen, Q. F. Xu, H. Li, J. H. He and J. M. Lu, J. Hazard. Mater., 2020, 399, 123109 CrossRef CAS PubMed.
- J. L. Zhou, Z. Q. Zhang, X. L. Kong, F. He, R. X. Zhao, R. Z. Wu, T. Wei, L. Wang and J. Feng, Appl. Surf. Sci., 2020, 510, 145442 CrossRef CAS.
- D. L. Han, Y. J. Han, J. Li, X. M. Liu, K. W. K. Yeung, Y. F. Zheng, Z. D. Cui, X. J. Yang, Y. Q. Liang, Z. Y. Li, S. L. Zhu, X. B. Yuan, X. B. Feng, C. Yang and S. L. Wu, Appl. Catal., B, 2020, 261, 118248 CrossRef CAS.
- J. L. Huang, J. F. Zhou, J. Y. Zhuang, H. Z. Gao, D. H. Huang, L. X. Wang, W. L. Wu, Q. B. Li, D. P. Yang and M. Y. Han, ACS Appl. Mater. Interfaces, 2017, 9, 36606–36614 CrossRef CAS PubMed.
- P. L. Yu, Y. J. Han, D. L. Han, X. M. Liu, Y. Q. Liang, Z. Y. Li, S. L. Zhu and S. L. Wu, J. Hazard. Mater., 2020, 390, 122126 CrossRef CAS PubMed.
- Y. P. Wang, F. C. Jiang, J. F. Chen, X. F. Sun, T. Xiao and H. Yang, Nanomaterials, 2020, 10, 178 CrossRef CAS PubMed.
- H. Y. Ding, D. L. Han, Y. J. Han, Y. Q. Liang, X. M. Liu, Z. Y. Li, S. L. Zhu and S. L. Wu, J. Hazard. Mater., 2020, 393, 122423 CrossRef CAS PubMed.
- R. Wang, X. Y. Kong, W. T. Zhang, W. X. Zhu, L. J. Huang, J. Wang, X. Zhang, X. N. Liu, N. Hu, Y. R. Suo and J. L. Wang, Appl. Catal., B, 2018, 225, 228–237 CrossRef CAS.
- Y. X. Chen, P. L. Wang, Y. Liang, M. J. Zhao, Y. Y. Jiang, G. T. Guang, P. Zou, J. Zeng, Y. S. Zhang and Y. Wang, J. Colloid Interface Sci., 2018, 536, 389–398 CrossRef PubMed.
- Y. C. Yan, X. Q. Zhou, P. Yu, Z. F. Li and T. L. Zheng, Appl. Catal., A, 2020, 590, 117282 CrossRef CAS.
- L. Ghalamchi, S. Aber, V. Vatanpour and M. Kian, J. Ind. Eng. Chem., 2019, 70, 412–426 CrossRef CAS.
- W. J. Wang, T. C. An, G. Y. Li, D. H. Xia, H. J. Zhao, J. C. Yu and P. K. Wong, Appl. Catal., B, 2017, 217, 570–580 CrossRef CAS.
- S. Vignesha, S. Suganthib, J. K. Sundar and V. Raj, J. Ind. Eng. Chem., 2019, 76, 318–332 CrossRef.
- S. P. Adhikari, H. R. Pant, J. H. Kim, H. J. Kim, C. H. Park and C. S. Kim, Colloids Surf., A, 2015, 482, 477–484 CrossRef CAS.
- B. K. Liu, L. L. Mu, X. L. Han, J. T. Zhang and H. Z. Shi, J. Photochem. Photobiol., A, 2019, 380, 111866 CrossRef CAS.
- V. Shanmugam, A. L. Muppudathi, S. Jayavel and K. S. Jeyapeiumal, Arabian J. Chem., 2018, 3, 2439–2455 Search PubMed.
- S. Vignesh, S. Suganthi, J. K. Sundar and V. Raj, Appl. Surf. Sci., 2019, 488, 763–777 CrossRef CAS.
- Y. P. Li, Q. Wang, L. Y. Huang, X. Q. Xu, M. Xie, H. Wang, S. Q. Huang, F. Zhang, Z. Y. Zhao and J. Yang, J. Mater. Sci.: Mater. Electron., 2018, 30, 2783–2794 CrossRef.
- B. K. Liu, Y. J. Wu, J. T. Zhang, X. L. Han and H. Z. Shi, J. Photochem. Photobiol., A, 2019, 378, 1–8 CrossRef CAS.
- I. Meenakshisundarama, S. Kalimuthua, G. Priya and S. Karthikeyan, Mater. Res. Bull., 2019, 112, 331–335 CrossRef.
- S. Ghafoor, A. Inayat, F. Aftab, H. Duran, K. Kirchhoff, S. Waseem and S. N. Arshad, J. Environ. Chem. Eng., 2019, 7, 103452 CrossRef CAS.
- D. Sun, Y. Zhang, Y. F. Liu, Z. G. Wang, X. C. Chen, Z. Y. Meng, S. F. Kang, Y. Y. Zheng, L. F. Cui, M. L. Chen, M. D. Dong and B. Hu, Chem. Eng. J., 2020, 384, 123259 CrossRef CAS.
- C. L. Ai, S. C. Wu, L. Y. Li, Y. J. Lei and X. W. Shao, Colloids Surf., A, 2019, 583, 123981 CrossRef CAS.
- H. X. Zhao, S. Chen, X. Quan, H. T. Yu and H. M. Zhao, Appl. Catal., B, 2016, 194, 134–140 CrossRef CAS.
- M. A. Khan, S. Mutahir, F. Y. Wang, J. W. Zhou, W. Lei and M. Z. Xia, Sol. Energy, 2019, 186, 204–214 CrossRef CAS.
- J. Deng, J. L. Liang, M. Li and M. P. Tong, Colloids Surf., B, 2017, 152, 49–57 CrossRef CAS PubMed.
- S. Vignesh, M. A. Lakshmi and J. K. Sundar, J. Mater. Sci., 2018, 29, 10784–10801 CAS.
- S. W. Zhao, M. Zheng, H. L. Sun, S. J. Li, Q. J. Pan and Y. R. Guo, Dalton Trans., 2020, 49, 3723–3734 RSC.
- D. Ayodhya and G. Veerabhadram, Mater. Res. Innovations, 2020, 24, 210–228 CrossRef CAS.
- C. Liu, Y. B. Tang, P. W. Huo and F. Y. Chen, Mater. Lett., 2019, 257, 126708 CrossRef CAS.
- H. Yan, Z. W. Zhu, Y. M. Long and W. F. Li, J. Photochem. Photobiol., A, 2020, 390, 112297 CrossRef CAS.
- W. N. Shen, X. Wang, Y. F. Ge, H. Feng and L. J. Feng, Colloids Surf., A, 2019, 575, 102–110 CrossRef CAS.
- D. Vidyasagar, A. Kulkarni, S. G. Ghugal and S. S. Umare, J. Chem. Technol. Biotechnol., 2019, 94, 761–767 CrossRef CAS.
- S. J. Gu, D. Zhang, S. R. Luo and H. Yang, Int. J. Photoenergy, 2019, 2019, 1–9 CrossRef.
- R. Gupta, B. Boruah, J. M. Modak and G. Madras, J. Photochem. Photobiol., A, 2019, 372, 108–121 CrossRef CAS.
- R. Nithya and S. Ayyappan, J. Photochem. Photobiol., A, 2020, 398, 112591 CrossRef CAS.
- M. A. Qamar, S. Shahid, M. Javed, S. Iqbal, M. Sher and M. B. Akbar, J. Photochem. Photobiol., A, 2020, 401, 112776 CrossRef CAS.
- X. L. Geng, L. Wang, L. Zhang, H. Wang, Y. Y. Peng and Z. Y. Bian, Chem. Eng. J., 2021, 420, 129722 CrossRef CAS.
- D. Yan, X. Wu, J. Y. Pei, C. C. Wu, X. M. Wang and H. Y. Zhao, Ceram. Int., 2020, 46, 696–702 CrossRef CAS.
- A. Balapure, Y. Nikhariya, N. S. S. Boppudi, R. Ganesan and J. R. Dutta, ACS Appl. Mater. Interfaces, 2020, 12, 21481–21493 CrossRef CAS PubMed.
- M. Faraji, N. Mohaghegh and A. Abedin, J. Photochem. Photobiol. B, 2018, 178, 124–132 CrossRef CAS PubMed.
- Y. W. Sun, X. Qi, R. Q. Li, Y. T. Xie, Q. Tang and B. X. Ren, Opt. Mater., 2020, 108, 110170 CrossRef CAS.
- D. Sun, J. Mao, L. Cheng, X. L. Yang, H. Li, L. X. Zhang, W. Zhang, Q. Zhang and P. W. Li, Chem. Eng. J., 2021, 418, 129417 CrossRef CAS.
- L. W. Wang, X. Zhang, X. Yu, F. Gao, Z. Y. Shen, X. L. Zhang, S. G. Ge, J. Liu, Z. J. Gu and C. Y. Chen, Adv. Mater., 2019, 31, 1901965 CrossRef PubMed.
- L. Du, C. Jin, Y. F. Cheng, L. Q. Xu, X. L. An, W. Shang, Y. P. Zhang and X. Rao, J. Alloys Compd., 2020, 842, 155612 CrossRef CAS.
- M. A. Qamar, S. Shahid, M. Javed, M. Sher, S. Iqbal, A. Bahadur and D. X. Li, Colloids Surf., A, 2021, 611, 125863 CrossRef CAS.
- S. A. Younis, P. Serp and H. N. Nassar, J. Hazard. Mater., 2021, 410, 124562 CrossRef CAS PubMed.
- M. Sher, S. A. Khan, S. Shahid, M. Javed, M. A. Qamar, A. Chinnathambi and H. S. Almoallim, J. Environ. Chem. Eng., 2021, 9, 105366 CrossRef CAS.
- J. H. Thurston, A. J. Clifford, B. S. Henderson, T. R. Smith, D. Quintana, K. F. Cudworth, T. J. Lujan and K. A. Cornell, ACS Appl. Bio Mater., 2020, 3, 1681–1689 CrossRef CAS.
- M. Oves, M. O. Ansari, R. Darwesh, A. Hussian, M. F. Alajmi and H. A. Qari, Coatings, 2020, 10, 950 CrossRef CAS.
- A. Ahmed, M. B. K. Niazi, Z. Jahan, T. Ahmad, A. Hussain, E. Pervaiz, H. A. Janjua and Z. Hussain, Eur. Polym. J., 2020, 130, 109650 CrossRef CAS.
- M. A. Qamar, S. Shahid and M. Javed, Ceram. Int., 2020, 46, 22171–22180 CrossRef CAS.
- C. Zhang, Y. Li, C. Wang and X. Y. Zheng, Sci. Total Environ., 2021, 755, 142588 CrossRef CAS PubMed.
- D. Ayodhya and G. Veerabhadram, J. Mol. Struct., 2019, 1186, 423–433 CrossRef CAS.
- S. P. Adhikari, G. P. Awasthi, J. Lee, C. H. Park and C. S. Kim, RSC Adv., 2016, 6, 55079–55091 RSC.
- J. G. Du, Z. Xu, H. Li, H. J. Yang, S. J. Xu, J. Wang, Y. A. Jia, S. L. Ma and S. H. Zhan, Appl. Surf. Sci., 2021, 541, 148487 CrossRef CAS.
- G. D. Fan, B. H. Du, J. J. Zhou, W. W. Yu, Z. Y. Chen and S. W. Yang, Appl. Catal., B, 2020, 265, 118610 CrossRef.
- G. Y. Li, X. Nie, J. Y. Chen, Q. Jiang, T. C. An, P. K. Wong, H. M. Zhang, H. J. Zhao and H. Yamashita, Water Res., 2015, 86, 17–24 CrossRef CAS PubMed.
- G. D. Fan, B. H. Du, J. J. Zhou, Z. S. Yan, Y. F. You and J. Luo, Chem. Eng. J., 2021, 404, 126509 CrossRef CAS.
- W. N. Shen, X. Wang, Y. F. Ge, H. Feng and L. J. Feng, Colloids Surf., A, 2019, 575, 102–110 CrossRef CAS.
- R. Cheng, L. J. Shen, J. H. Yu, S. Y. Xiang and X. Zheng, Catalysts, 2018, 8, 406 CrossRef.
- S. H. Zhan, Q. L. Hou, Y. Li, S. L. Ma, P. F. Wang, Y. A. Li and H. T. Wang, RSC Adv., 2018, 8, 34428–34436 RSC.
- Y. Liu, X. K. Zeng, X. Y. Hu, J. Hu, Z. Y. Wang, Y. C. Yin, C. H. Sun and X. W. Zhang, Catal. Today, 2019, 335, 243–251 CrossRef CAS.
- J. Xu, Q. Z. Gao, Z. P. Wang and Y. F. Zhu, Appl. Catal., B, 2021, 291, 120059 CrossRef CAS.
- R. A. Rather and I. M. C. Lo, Water Res., 2020, 168, 115166 CrossRef CAS PubMed.
- M. Sher, M. Javed, S. Shahid, S. Iqbal, M. A. Qamar, A. Bahadur and M. A. Qayyum, RSC Adv., 2021, 11, 2025–2039 RSC.
- W. J. Wang, J. C. Yu, D. H. Xia, P. K. Wong and Y. C. Li, Environ. Sci. Technol., 2013, 47, 8724–8732 CrossRef CAS PubMed.
|
This journal is © The Royal Society of Chemistry 2021 |
Click here to see how this site uses Cookies. View our privacy policy here.