DOI:
10.1039/D1NA00101A
(Communication)
Nanoscale Adv., 2021,
3, 2217-2221
Antiferromagnetic spin ordering in two-dimensional honeycomb lattice of SiP3†
Received
6th February 2021
, Accepted 20th March 2021
First published on 22nd March 2021
Abstract
Magnetism in low-dimensional materials has been of sustained interest due to its intriguing quantum mechanical origin and promising device applications. Here, we propose a buckled honeycomb lattice of stoichiometry SiP3, a two-dimensional binary group-IV and V material that exhibits an antiferromagnetic ground state with itinerant electrons. Here we perform elemental Si substitution in pristine blue phosphorene to downshift the Fermi energy and induce the Fermi instability that results in a spin polarized ground state. Within first-principles calculations, we observe antiferromagnetic spin alignment between adjacent ferromagnetic triangular domains where each Si atom is coupled with three neighboring P atoms with a ferromagnetic interaction. Such unique spin structure and resulting magnetic ground state are unprecedented in defect-free two-dimensional materials made of only p-block elements. This metal-free magnetism can be exploited for advanced spintronic and memory storage applications.
1 Introduction
Two-dimensional (2D) materials have been of great interest in recent times, owing to their interesting electronic properties arising from quantum confinement.1,2 Inspired by the isolation of graphene, a Dirac semimetal, extensive investigations have been continuing over the last decade to explore a plethora of other 2D materials with varying functionalities.3,4 One of the major quests has been the magnetism in such reduced dimension, mainly owing to their application possibilities in advanced spintronic and memory devices.5–7 This has so far been achieved by structural or chemical modifications, defect engineering, doping, external perturbations etc.8–12
In this regard, graphene nanoribbon has been one of the most extensively studied systems, where the zigzag edge tends to localize the spins.13–15 Actually, the partial flat bands at Fermi energy in non-interacting approximation, originating from zigzag edges result in diverging density of states (DOS) and consequent Fermi instability. Inclusion of electron–electron interactions splits those degenerate bands at Fermi energy to open up a gap and localizes the spins in ferrimagnetic alignment along the zigzag edge.2 These theoretical predictions have been confirmed within scanning tunneling spectroscopy experiments as well.16–18 However, experimental realization of long and smooth zigzag edge has still been challenging.19 The observation of such magnetic ordering has been elusive in defect-free 2D materials till the recent discovery of transition metal trichalcogenides, halides,20 borides21 and carbon organic frameworks.22–24 It is mainly due to the fact that, the long range magnetic ordering gets suppressed due to thermal agitation, as described by Mermin–Wagner theory.25
However, the recent observations of pristine 2D ferromagnetic van der Waals crystals containing transition metal atoms have shown the influence of uniaxial magnetic anisotropy to stabilize such magnetic ordering of d-electrons.20,26 This can be exploited for advanced device applications. However, the metal-based devices have several limitations, e.g., high cost, heavy, low durability and susceptible to corrosion. Therefore, here we asked a question: can we design a metal-free 2D magnetic material ? This lead us to search for 2D materials that are made of p-block elements. Here, we report silicon (Si) substituted 2D blue phosphorene, SiP3 which shows overall antiferromagnetic ground state with out-of-plane spin orientation. The magnetism has been achieved by inducing Fermi instability via down-shifting the Fermi energy of nonmagnetic blue phosphorene by hole doping through elemental Si substitution.
2 Computational details
We adopt the density functional theory (DFT) as implemented in SIESTA27 to explore the electronic and magnetic properties of pristine and doped 2D blue phosphorene. Generalized Gradient Approximation (GGA) is considered with Perdew–Burke–Ernzerhof (PBE) exchange and correlation functional.28 Troullier Martin scheme is adopted to generate the norm-conserving pseudopotentials.29 Double-ζ polarized basis set is used with 400 Ry energy cut-off for real space mesh size. Structural optimizations are performed using conjugate-gradient method along with the relaxation of lattice vectors until force on each atoms becomes less than 0.01 eV Å−1. We consider a vacuum of 15 Å in the non-periodic direction to avoid any interaction between adjacent unit cells. We consider Brillouin zone sampling over 16 × 16 × 1 and 48 × 48 × 1 Monkhorst–Pack grids for geometric relaxation and subsequent electronic property calculations, respectively. To check the dynamical stability, we further investigate the phonon dispersions using 2 × 3 × 1 supercell and k-mesh of 16 × 16 × 1 with mesh cut-off of 1600 Ry. Since, the consideration of localized orbital basis in SIESTA overestimates the band gap, we perform further calculations using plane-wave basis as implemented in VASP30–32 for comparison and present them in ESI.†
3 Results and discussion
The structure of 2D monolayer pristine blue phosphorene is shown in Fig. 1(a). It shows honeycomb lattice with out-of-plane buckling (Δz) and its ground state does not show any spin-polarization. This system has been obtained experimentally and is known to exhibit high charge carrier mobility.33,34 In Fig. 1(b), we present the electronic band dispersion of its 2 × 2 supercell which shows semiconducting band gap of ∼2 eV. Most interestingly, the band structure shows nearly flat top of the valence band and corresponding diverging DOS. We have been motivated by the fact that, if the Fermi energy can be down-shifted by hole doping to align with this diverging DOS, it might be possible to achieve magnetic ground state, as observed in case of zigzag edge graphene nanoribbons.13 However, since the Fermi energy can be located anywhere in the theoretically estimated large semiconducting gap of ∼2 eV, it is experimentally unlikely to achieve the required Fermi level shift by p-type doping through gate electrode. Therefore, we adopt the strategy of elemental substitution of phosphorus (P) atoms by silicon (Si) atoms that has one less electron, to dope the system with holes.
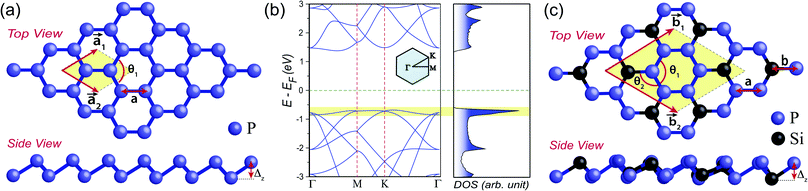 |
| Fig. 1 (a) The structure of pristine blue phosphorene, (b) band structure and density of states (DOS) of the same in 2 × 2 supercell and (c) the structure of SiP3. The shaded rhombuses in (a) and (c) show the corresponding unit cells with lattice vectors, and , respectively. The P–P (P–Si) bond length is denoted by a (b) and the ∠P–P–P (∠P–P–Si) is denoted by θ1 (θ2). Δz quantifies the out-of-plane buckling. The vertical and horizontal dashed lines in (b) show high-symmetric points in the irreducible Brillouin zone, shown in the inset and Fermi energy, respectively. | |
This kind of elemental substitution is known to produce shift in Fermi energy by a large extent, as has been observed in case of 2D binary compound BC3.35–37 This exhibits planar honeycomb lattice with homogeneously placed substitutional boron (B) atoms in graphene lattice, such that, every carbon hexagon is surrounded by six B atoms.38,39 Owing to one less electron in each B atom as compared to carbon (C) atom, the system gets hole doped with consequent down-shift of Fermi energy by ∼2 eV.36 Therefore, such elemental substitution appears to be a viable solution in present study as well and we consider similar stoichiometry of SiP3 to bring the Fermi energy down. Fig. 1(c) shows the structure of SiP3, where phosphorus hexagons are symmetrically surrounded by six Si atoms, resulting in a 8 atom unit cell. That is why, we choose to present the band structure of 2 × 2 supercell of pristine blue phosphorene as well in Fig. 1(b) for better comparison. Such group-IV–group-V binary compounds indeed exist in layered form with same stoichiometry and lattice geometry, as evident from previous studies.40–44
We investigate the substitution by other group-IV elements, e.g., C, Ge and Sn as well. However, we observe minimal structural distortion in case of Si substitution in pristine blue phosphorene (see Tables S1 and S2 in ESI†). This can be attributed to the minimal difference of atomic radius of P and Si for being in the same period and hence unaltered hybridization as compared to pristine blue phosphorene and silicene (see Table S3 in ESI†). In Table 1, we present the structural parameters of pristine and Si substituted blue phosphorene, which show close resemblance. However, due to the change in the buckling parameter for P–Si bond the associated ∠P–P–Si (θ2) show considerable increase (see Table 1 and Fig. 1(c)).
Table 1 The structural parameters of pristine blue phosphorene and SiP3: lattice constants (a1/b1), P–P bond lengths (a), P–Si bond lengths (b), ∠P–P–P (θ1), ∠P–P–Si (θ2) and out-of-plane buckling parameters (Δz(P–P) and Δz(P–Si)). All these are shown in Fig. 1(a) and (c). Note that, the lengths and angles are in Å and degree, respectively
System |
a
1/b1 |
a(b) |
θ
1
|
θ
2
|
Δ
z(P–P)
|
Δ
z(P–Si)
|
Phosphorene |
3.326 |
2.31 (−) |
92.22 |
— |
1.28 |
— |
SiP3 |
6.926 |
2.30 (2.31) |
91.78 |
97.92 |
1.287 |
0.983 |
For further stability analysis, we investigate the phonon dispersion of SiP3 and present in Fig. 2. The absence of any imaginary phonon mode and well separated acoustic and optical modes clearly indicate the dynamical stability of the system. The quadratic dispersion of the flexural out-of-plane (ZA) acoustic mode shows nice resemblance with that of pristine blue phosphorene,45 showing the robust and rigid 2D architecture. Moreover, the in-plane elastic response shows isotropic nature, as evident from the similar slopes of longitudinal acoustic branch (LA) near Γ points at both the ends of Fig. 2. This observation also resembles with that of pristine blue phosphorene45 and proves the existence of the inversion symmetry even with asymmetric P–P and P–Si buckling amplitudes in SiP3.
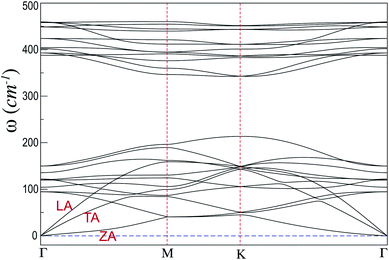 |
| Fig. 2 Phonon dispersion of SiP3 system with high-symmetric points (vertical dashed lines). | |
We then calculate the electronic band structure and density of states of SiP3 without and with spin-polarization. The band structure for nonmagnetic (NM) approximation shows partial dispersion-less band and corresponding diverging density of states near the Fermi energy (see Fig. 3(a)). These bands originate from considerable band mixing due to strong hybridization of P and Si. This is confirmed by further fat band calculation that shows complete hybrid nature of the bands near Fermi energy, with contributions from 3p orbitals of both P and Si. Nevertheless, the Fermi instability is still arising from the dispersion-less hybrid bands that can potentially lead to magnetic ground state, as has been observed in case of zigzag edge graphene nanoribbons.2,13–18
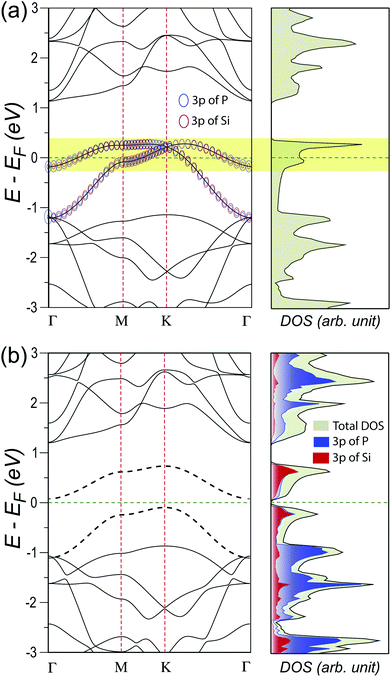 |
| Fig. 3 (a) The band structure and the density of states (DOS) of SiP3 system in nonmagnetic ground states. The contributions from 3p orbitals of Si and P atoms are shown in terms of fat bands for the two bands near Fermi energy. The size of the circles is proportional to the extent of contribution. (b) The band structure and DOS of the same system in antiferromagnetic ground state. The two central bands are shown as dotted lines. The projected DOS from 3p orbitals of Si and P are also shown. The vertical (horizontal) lines show the high-symmetric points (Fermi energy). | |
Further spin-polarized calculations with both ferromagnetic (FM) and antiferromagnetic (AFM) initial guesses with subsequent self-consistent electronic relaxations show 13 and 74 meV per unit cell more stability as compared to the NM state, respectively. This clearly indicates the preferential magnetic ground state with non-zero spin moments on atomic sites in 2D SiP3. This observation is in striking contrast to that of other known 2D materials made of p-block elements, which do not allow any bulk polarization of spins in absence of any defects, e.g., vacancies or edges.2,8 Such unique magnetic ground state of 2D SiP3 has not been observed in case of other group-IV elemental substitutions as well,42–44 due to the lack of Fermi instability in their NM energy dispersions (see Fig. S1 in ESI†). Owing to the highest stability, we further proceed with the AFM ground state of SiP3.
We first investigate the stabilization energy of such ground state as compared to that of pristine one. The stabilization energies per atom are calculated using the equation,
Here Etotal is the total energy of the system, Ei and Ni are the single atom energy and total number of atoms of i-th element and N is the total number of atoms in the unit cell. We find the SiP3 system is more stable than the pristine blue phosphorene by ∼0.11 eV per atom. Even the system with stoichiometry Si0.5P3.5, i.e., with only one substituted Si atom in the 2 × 2 supercell of blue phosphorene shows a ∼0.04 eV per atom higher stability as compared to its parent system. This indicates a stability gain by gradual Si replacement in pristine layer even at room temperature (∼25 meV). Such stability analysis along with the stable phonon dispersion shows that the synthesis of SiP3 system is experimentally conducive. It can be achieved by selective and systematic Si replacement in blue phosphorene, as has been observed in recent experiments.46–49
Now in the AFM ground state, the two NM hybrid bands at Fermi energy gets separated and opens up a tiny indirect band gap of 0.17 eV, as can be seen in Fig. 3(b). The hybrid nature of these two bands is evident from the comparable projected DOS from 3p orbitals of both P and Si. Such a small gap can easily be closed by thermal fluctuation of the Fermi surface at finite temperature or by small external perturbations. Our further investigation within plane-wave approximation results in comparatively more dispersive bands that cross the Fermi energy and results in metallic behavior (see Fig. S2 in ESI†). Therefore, we infer the ground state of SiP3 to be more likely an itinerant AFM state. In addition to that, it shows very peculiar spin ordering. The spins on each Si atom and its three neighboring P atoms are ferromagnetically coupled. However, the hexagons consisting of only P atoms show perfectly bipartite nature of opposite spin alignments between nearest neighbors. This leads to two triangular tiny ferromagnetic domains with opposite spin orientations in the rhombus unit cell, as can be seen in Fig. 4(a). The AFM ground state ensures localization of opposite spins on two Si atoms in the unit cell. Further charge transfer from neighboring P atoms to the Si atom creates imbalance between up and down spins on each P atom, resulting in a bipartite spin ordering in the P-hexagon (see Table S3 in ESI†). Such charge transfer happens due to the Lewis base character of P atoms. This sets up the ferromagnetic coupling between neighboring P and Si atoms, leading to the overall AFM spin ordering in SiP3, as shown schematically in Fig. 4(b). Note that, such Fermi instability induced long range magnetic ordering has been observed in case of zigzag edge graphene nanoribbons.2,13–18
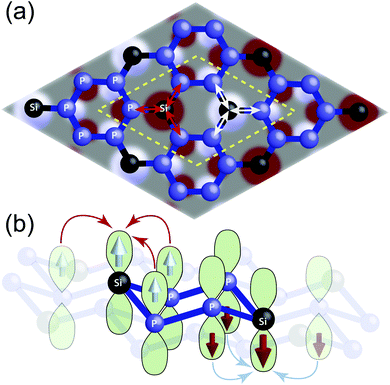 |
| Fig. 4 (a) Spin–density plot of SiP3 with two different colors denoting up and down spin orientations. The dashed rhombus shows the unit cell and the double-headed arrows show the ferromagnetic coupling. (b) Schematic representation of π-electron orbitals of SiP3 with spin transfer directions shown by the arrows. | |
Our detailed investigation of systems with other group IV elements, e.g., C, Ge and Sn always result in NM ground state within both localized and plane-wave basis (see Tables S4, S5 and Fig. S1, S3 in ESI†).
4 Conclusions
In conclusion, within first-principles calculations, we exploit the partially flat top of the valence band and consequent diverging density of states of pristine blue phosphorene to induce Fermi instability. This has been done by downshift of Fermi energy via elemental Si substitution that leads to SiP3 stoichiometry. This system exhibits itinerant AFM ground state with a unique spin ordering, where each Si atom is ferromagnetically coupled with three neighboring P atoms, however maintaining antiferromagnetic spin orientation between such neighboring triangular domains. This unique spin alignment primarily arises due to the charge transfer from neighboring P atoms to Si atoms. The ab initio energetics and phonon dispersion analysis show stability of this proposed system in ambient conditions. The observation of such magnetic ground state is unprecedented in 2D materials that are made of purely p-block elements in absence of any defect, twist or external perturbations. Further experimental exploration is necessary to confirm such long range ordering at finite temperature. This metal-free magnetism with unique spin texture can find huge spintronic, memory storage and qubit applications and will tempt further theoretical and experimental explorations.
Author contributions
SD and SM have defined the project. SA has performed the calculations. All the authors have analyzed the results and written the manuscript.
Conflicts of interest
The authors declare no competing financial interest.
Acknowledgements
SA and SD thank IISER Tirupati for Intramural Funding and Science and Engineering Research Board (SERB), Dept. of Science and Technology (DST), Govt. of India for Early Career Research (ECR) award grant (ECR/2016/000283). SM acknowledges the SERB, DST, Govt. of India for ECR award grant (ECR/2016/001431) and DST INSPIRE-Faculty award grant (IFA 14-PH-96). The authors thank Prof. K. Wakabayashi (Kwansei Gakuin University) for illuminating discussions. The support and the resources provided by ‘PARAM Brahma Facility’ under the National Supercomputing Mission, Government of India at the Indian Institute of Science Education and Research (IISER) Pune are gratefully acknowledged.
Notes and references
- A. H. Castro Neto, F. Guinea, N. M. R. Peres, K. S. Novoselov and A. K. Geim, Rev. Mod. Phys., 2009, 81, 109 CrossRef CAS.
- K. Wakabayashi and S. Dutta, Solid State Commun., 2012, 152, 1420 CrossRef CAS.
- K. S. Novoselov, A. Mishchenko, A. Carvalho and A. H. Castro Neto, Science, 2016, 353, 6298 CrossRef PubMed.
- A. K. Geim and K. S. Novoselov, Nat. Mater., 2007, 6, 183 CrossRef CAS PubMed.
- K. Wakabayashi, M. Fujita, H. Ajiki and M. Sigrist, Phys. Rev. B: Condens. Matter Mater. Phys., 1999, 59, 8271 CrossRef CAS.
- W. Han, R. K. Kawakami, M. Gmitra and J. Fabian, Nat. Nanotechnol., 2014, 9, 794 CrossRef CAS PubMed.
- A. Avsar, H. Ochoa, F. Guinea, B. Özyilmaz, B. J. van Wees and I. J. Vera-Marun, Rev. Mod. Phys., 2020, 92, 021003 CrossRef CAS.
- O. V. Yazyev and L. Helm, Phys. Rev. B: Condens. Matter Mater. Phys., 2007, 75, 125408 CrossRef.
- Y. W. Son, M. L. Cohen and S. G. Louie, Nature, 2006, 444, 347 CrossRef CAS PubMed.
- S. Dutta, A. K. Manna and S. K. Pati, Phys. Rev. Lett., 2009, 102, 096601 CrossRef PubMed.
- A. L. Sharpe, E. J. Fox, A. W. Barnard, J. Finney, K. Watanabe, T. Taniguchi, M. A. Kastner and D. Goldhaber-Gordon, Science, 2019, 365, 605 CrossRef CAS PubMed.
- T. M. R. Wolf, J. L. Lado, G. Blatter and O. Zilberberg, Phys. Rev. Lett., 2019, 123, 096802 CrossRef CAS PubMed.
- M. Fujita, K. Wakabayashi, K. Nakada and K. Kusakabe, J. Phys. Soc. Jpn., 1996, 65, 1920 CrossRef CAS.
- K. Wakabayashi, M. Sigrist and M. Fujita, J. Phys. Soc. Jpn., 1998, 67, 2089 CrossRef CAS.
- A. Yamashiro, Y. Shimoi, K. Harigaya and K. Wakabayashi, Phys. Rev. B: Condens. Matter Mater. Phys., 2003, 68, 193410 CrossRef.
- C. Tao,
et al.
, Nat. Phys., 2011, 7, 616 Search PubMed.
- G. Z. Magda, X. Jin, I. Hagymási, P. Vancsó, Z. Osváth, P. Nemes-Incze, C. Hwang, L. P. Biro and L. Tapaszto, Nature, 2014, 514, 608 CrossRef CAS PubMed.
- S. Wang, L. Talirz, C. A. Pignedoli, X. Feng, K. Müllen, R. Fasel and P. Ruffieux, Nat. Commun., 2016, 7, 11507 CrossRef CAS PubMed.
- P. Ruffieux,
et al.
, Nature, 2016, 531, 489 CrossRef CAS PubMed.
- C. Gong and X. Zhang, Science, 2019, 363, eaav4450 CrossRef CAS PubMed.
- C. Tang, K. Ostrikov, S. Sanvito and A. Du, Nanoscale Horiz., 2021, 6, 43 RSC.
- E. Jin,
et al.
, Science, 2017, 357, 673 CrossRef CAS PubMed.
- W. Jiang, H. Huang and F. Liu, Nat. Commun., 2019, 10, 2207 CrossRef PubMed.
- Y. Zhou and F. Liu, Nano Lett., 2021, 21, 230 CrossRef CAS PubMed.
- N. D. Mermin and H. Wagner, Phys. Rev. Lett., 1966, 17, 1133 CrossRef CAS.
- J. L Lado and J. Fernández-Rossier, 2D Mater., 2017, 4, 035002 CrossRef.
- J. M Soler, E. Artacho, J. D Gale, A. García, J. Junquera, P. Ordejón and D. Sánchez-Portal, J. Phys.: Condens. Matter, 2002, 14, 2745 CrossRef.
- J. P. Perdew, K. Burke and M. Ernzerhof, Phys. Rev. Lett., 1996, 77, 3865 CrossRef CAS PubMed.
- N. Troullier and J. L. Martins, Phys. Rev. B: Condens. Matter Mater. Phys., 1991, 43, 1993 CrossRef CAS PubMed.
- G. Kresse and J. Furthmüller, Phys. Rev. B: Condens. Matter Mater. Phys., 1996, 54, 11169 CrossRef CAS PubMed.
- G. Kresse and J. Furthmüller, Comput. Mater. Sci., 1996, 6, 15 CrossRef CAS.
- G. Kresse and D. Joubert, Phys. Rev. B: Condens. Matter Mater. Phys., 1999, 59, 1758 CrossRef CAS.
- J. L. Zhang, S. Zhao, C. Han, Z. Wang, S. Zhong, S. Sun, R. Guo, X. Zhou, C. D. Gu, K. D. Yuan, Z. Li and W. Chen, Nano Lett., 2016, 16, 4903 CrossRef CAS.
- J. Zeng, P. Cui and Z. Zhang, Phys. Rev. Lett., 2017, 118, 046101 CrossRef.
- D. Tománek, R. M. Wentzcovitch, S. G. Louie and M. L. Cohen, Phys. Rev. B: Condens. Matter Mater. Phys., 1998, 37, 3134(R) CrossRef PubMed.
- S. Dutta and K. Wakabayashi, J. Mater. Chem., 2012, 22, 20881 RSC.
- T. Kameda, F. Liu, S. Dutta and K. Wakabayashi, Phys. Rev. B: Condens. Matter Mater. Phys., 2019, 99, 075426 CrossRef.
- H. Tanaka, Y. Kawamata, H. Simizu, T. Fujita, H. Yanagisawa, S. Otani and C. Oshima, Solid State Commun., 2005, 136, 22 CrossRef CAS.
- H. Yanagisawa, T. Tanaka, Y. Ishida, E. Rokuta, S. Otani and C. Oshima, Phys. Rev. B: Condens. Matter Mater. Phys., 2006, 73, 045412 CrossRef.
- P. C. Donohue and H. S. Young, J. Solid State Chem., 1970, 1, 143 CrossRef CAS.
- J. Gullman and O. Olofsson, J. Solid State Chem., 1972, 5, 441 CrossRef CAS.
- B. Ghosh, S. Puri, A. Agarwal and S. Bhowmick, J. Phys. Chem. C, 2018, 122, 18185 CrossRef CAS.
- Y. Jing, Y. Ma, Y. Li and T. Heine, Nano Lett., 2017, 17, 1833 CrossRef CAS PubMed.
- M. Kar, R. Sarkar, S. Pal and P. Sarkar, Phys. Rev. B: Condens. Matter Mater. Phys., 2020, 101, 195305 CrossRef CAS.
- Z. Zhu and D. Tománek, Phys. Rev. Lett., 2014, 112, 176802 CrossRef PubMed.
- H. Wang,
et al.
, Nano Lett., 2012, 12, 141 CrossRef CAS PubMed.
- M. Tripathi, A. Mittelberger, N. A. Pike, C. Mangler, J. C. Meyer, M. J. Verstraete, J. Kotakoski and T. Susi, Nano Lett., 2018, 18, 5319 CrossRef CAS PubMed.
- S.-Y. Cho, H.-J. Koh, H.-W. Yoo and H.-T. Jung, Chem. Mater., 2017, 29, 7197 CrossRef CAS.
- K. Zhang,
et al.
, Nano Lett., 2015, 15, 6586 CrossRef CAS PubMed.
Footnote |
† Electronic supplementary information (ESI) available. See DOI: 10.1039/d1na00101a |
|
This journal is © The Royal Society of Chemistry 2021 |