DOI:
10.1039/D1NA00050K
(Review Article)
Nanoscale Adv., 2021,
3, 2741-2776
Mechanical properties of aerospace epoxy composites reinforced with 2D nano-fillers: current status and road to industrialization
Received
20th January 2021
, Accepted 24th March 2021
First published on 29th March 2021
Abstract
High-performance epoxy composites find application in the aerospace industry. Although epoxy is a high-performance polymer, its fracture toughness is compromised due to its highly cross-linked nature. Nanomaterials such as carbon nanotubes (CNTs), graphene derivatives, and inorganic 2-dimensional (2D) nanomaterials are being explored to improve epoxy composites' mechanical properties. Graphene is one of the most popular 2D nano-reinforcing agents for epoxy composites. Following graphene discovery, the research community's attention was brought to various other few-atom thick 2D nanomaterials. Hence, apart from graphene, inorganic nanosheets such as transition metal dichalcogenides (TMDs), hexagonal boron nitride (hBN), etc., are also being studied as modifiers for enhancing the mechanical performance of epoxy composites. Graphene, TMDs and hBN are known to possess a high aspect ratio, high specific surface area and inherently high mechanical strength and stiffness, contributing to a stronger and tougher composite. Despite that, the challenges associated with these nanomaterials, such as dispersion issues, lack of standardization, underlying health hazards, etc., have hampered their commercialization. It has been long past a decade since the discovery of graphene, yet there are concerns regarding the lab to industry scale-up, and health and environmental hazards associated with nanomaterials for the fabrication of aerospace composites. This review offers a comprehensive literature survey and a perspective into the possible ways of bridging the gaps between the laboratory research and industrialization of 2D nanosheet-filled epoxy composites.
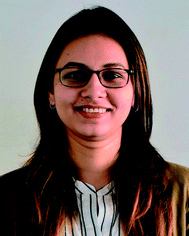 Radhika Wazalwar | Radhika Wazalwar is currently a Ph.D. student at the Department of Materials Engineering, Indian Institute of Science, Bengaluru, India. Radhika graduated with a Bachelors in Technology from the Department of Metallurgical and Materials Engineering, Visvesvaraya National Institute of Technology, Nagpur, India in 2016. Presently she is working on chemical modification of 2D nano-fillers for enhancing the mechanical behaviour of epoxy composites under the supervision of Prof. Ashok M. Raichur. |
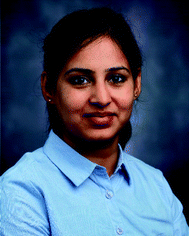 Megha Sahu | Dr Megha Sahu is currently working as a Materials and Process Senior Engineer at Boeing India Private Limited, Bengaluru, India. She obtained her Ph.D. from the Department of Materials Engineering, Indian Institute of Science, Bengaluru, India in 2017 and holds a Masters degree in Chemistry from Lucknow University, India. Dr Sahu has filed more than 15 patents in the field of polymers, additive manufacturing and composites. Her research work includes graphene based advanced epoxy composites, nanomaterial synthesis and chemical alteration of nanomaterials for application related to improved mechanical behaviour of polymer composites. |
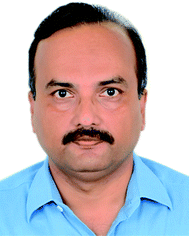 Ashok M. Raichur | Ashok M. Raichur is currently a professor at the Department of Materials Engineering, Indian Institute of Science (IISc), Bengaluru, India. He received his Ph.D. from the Department of Metallurgical Engineering, University of Nevada, Reno, USA in 1996 and joined IISc as a faculty in 1997. His group works on diverse research areas including 2D and core–shell nanomaterials for epoxy toughening, novel nanomaterials for biomedical applications and developing new generation of drug delivery systems for gene and anticancer drug delivery. |
1. Introduction
Metal alloys, especially aluminum alloys, have been an indispensable part of the aerospace industry for a long time. A large portion of the product range offered by aircraft manufacturers still comprises of metal aircraft. However, since the last decade, there has been a shift towards polymer composites. Modern planes such as Boeing 787 and Airbus A380, which came into service in the last decade, utilize more than 50% by weight carbon fiber reinforced epoxy composites in the aircraft fuselage, wings and empennage assemblies. In comparison to metals, polymer composites, especially epoxy composites, offer several advantages. They possess higher corrosion resistance, better strength to weight ratio, require fewer sub-assemblies, consume less fuel due to reduced weight, offer easier repair and maintenance, etc. However, there are some limitations associated with epoxy composites. Like most other polymers, epoxy is also thermally and electrically non-conducting, which leads to its inability to deal with electromagnetic interference and lightning strikes. Another limitation of epoxy is that it is highly brittle due to its heavily cross-linked network. Aircraft structures undergo extreme thermal cycles, mechanical load and fatigue and need to have good fracture toughness so that catastrophic failure does not occur. Researchers are continuously trying to improve the mechanical properties of epoxy composites, focusing on fracture toughness. Many toughening agents such as rubber,1,2 thermoplastic polymer particles, silica nanoparticles,3 and nanoclay4 have been explored to enhance epoxy's mechanical properties. Several researchers have been exploring CNTs,5,6 graphene, and more recently, inorganic 2D nanomaterials7,8 for the improved mechanical performance of epoxy composites. Amongst these, 2D nanomaterials are popular due to their high aspect ratio and inherently high mechanical strength.9
Many review articles have been published on the subject of nano-fillers for epoxy. Domun et al.10 comprehensively reviewed the status of epoxy composites reinforced with single and multiwalled CNTs, graphene, nano-silica and nano-clay. The authors summarized the effect of these nano-fillers on the tensile and fracture properties of epoxy composites. They pointed out the lack of uniformity in the composite fabrication methods, filler functionalization, and dispersion techniques used, restricting the understanding of the composites' mechanical properties. The lack of suitable quantitative methods to assess filler dispersion and filler-matrix interaction was also mentioned. A review article by Marouf et al.11 discussed and compared the effect of one, two and three-dimensional nano-fillers on the fracture properties of epoxy composites. The underlying toughening mechanisms in binary and ternary epoxy composites reinforced with nanofillers such as nano-silica, nano-rubber, CNTs, nanoclay and graphene were discussed in detail in this article. Szeluga et al.12 reviewed graphene fillers' effect on the mechanical, thermal, electrical and flame retardant properties of epoxy composites. The effect of filler size, degree of exfoliation and functionality on the properties of epoxy composites was summarized. The authors pointed out that there is insufficient literature to assess the filler-matrix interaction accurately. Atif et al.13 reviewed epoxy composites reinforced with graphene. They established a correlation of the filler size, morphology, and functionalization extent with the final epoxy composites' mechanical, thermal, and electrical properties. The authors pointed out the lack of consensus regarding graphene's effect on the properties of epoxy nanocomposites.
In a review by Kulkarni et al.,14 the authors described the covalent and non-covalent functionalization approaches for surface modification of graphene. The authors discussed the effects of processing protocols, filler dispersion, and filler surface modification on graphene–epoxy composites' electrical, mechanical, and thermal properties. Singh et al.15 reviewed graphene, CNTs, and graphene/CNT hybrid reinforced epoxy composites. The influence of the filler's type and functionality on the mechanical, thermal, electrical and flame-retardant properties of epoxy composites was discussed. The various applications of such composites, especially in aircraft body, electro-magnetic shielding, corrosion-resistant coatings, etc., were discussed.
Research groups worldwide have extensively worked on epoxy reinforced with graphene and inorganic 2D nanofillers and have produced promising results in terms of the mechanical and thermomechanical performance of these composites. Despite the abundant literature on 2D nanomaterial-filled epoxy resins, it has still not graduated from the laboratory to the aircraft industry. Hence, a perspective is needed in terms of the research conducted in this field, its challenges, and the possible solutions to these challenges.
The review articles on 2D nanofiller reinforced epoxy composites available so far have majorly taken into consideration graphene and its derivatives. To the best of our knowledge, the status of inorganic 2D nano-fillers, especially TMDs and hBN and their various organic and inorganic multiscale hybrids, which are being extensively explored to reinforce epoxy composites, has not been reviewed yet. Many authors have discussed the problems associated with the processing and dispersion of nano-fillers. However, successful commercialization of such epoxy nanocomposites is being hampered by challenges such as health hazards, lack of standardization of nanomaterials, and effective scale-up of technology, which have not been discussed in the review articles so far.
The current review comprises an exhaustive literature survey on epoxy composites loaded with 2D nanomaterials, including graphene and its derivatives, TMDs, hBN and other 2D inorganic nanomaterials and their hybrids. Fig. 1 shows a schematic of the structure of graphene and TMDs and their multiscale hybrids. However, since this review focuses on 2D fillers, only 2D–2D hybrids have been covered in detail. The effect of filler size, filler chemistry, loading level and dispersion state on the mechanical and thermomechanical properties of epoxy resin has been discussed. Furthermore, a holistic view of the major challenges of using 2D nanosheets as potential fillers for epoxy resin has been brought to light. This review tries to rationalize the projected potential of graphene and other 2D materials used in the fabrication of epoxy composites and highlights the gap between the laboratory and industry. The difficulties associated with the standardization and scale-up, and health hazards related to the use of 2D nanomaterials have also been discussed. Fig. 2 schematically summarizes the idea behind the review article, covering the types of 2D fillers used to make epoxy composites, their applications in the aerospace industry and various challenges around such nanocomposites.
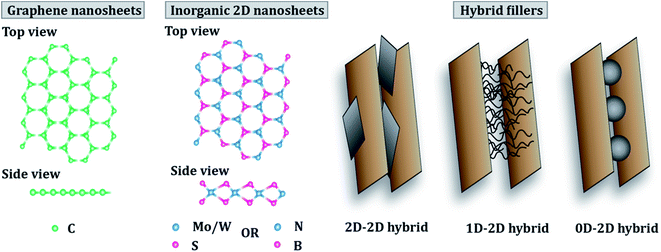 |
| Fig. 1 2D fillers and their multiscale hybrids. | |
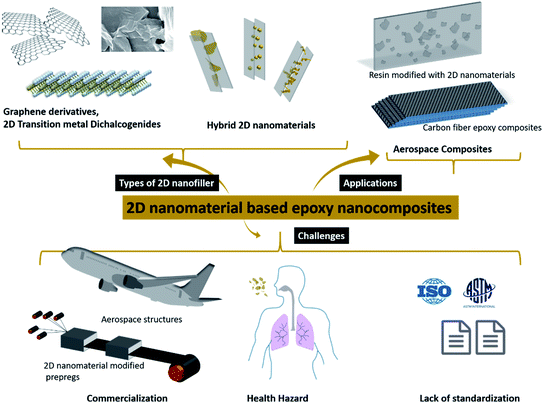 |
| Fig. 2 Types of 2D nanomaterials and applications and challenges of their epoxy-based nanocomposites. | |
2. 2D nano-materials
This section briefly touches upon the various synthesis methods of 2D nanosheets such as graphene, TMDs, hBN, etc., along with their basic properties and applications. Such 2D nanomaterials are often subjected to surface modification to improve their performance as fillers in composite materials. These functionalization strategies have also been discussed.
2.1 Graphene
Graphene is a single layer of carbon atoms arranged in a 2D honeycomb lattice. Graphene is the building block of 0D (fullerenes), 1D (CNTs) and 3D graphitic materials.16 Monolayer graphene displays a Young's modulus of 1 TPa and intrinsic tensile strength of 130 GPa.9 Graphene exhibits a large theoretical surface area of 2630 m2 g−1, unique optical properties, ultrahigh electronic mobility (>200
000 cm2 V−1 s−1)17,18 and high thermal conductivity (>5000 W m−1 K−1).19 These excellent properties make graphene a go-to material for myriad applications, including sensors, conductive films, polymer composites, photonics, etc.20 The robust and flexible nature of graphene opens possibilities for its surface modification.21Table 1 summarizes the inherent mechanical properties of some nano-fillers. The popularity of graphene extends to several research areas. Due to its versatile nature, it is used in the biomedical industry,22 sensors and electronic devices,23,24 wastewater treatment,25 optical applications,26,27 thermal conductivity applications,28 polymer composites etc.
Table 1 Mechanical properties of nanomaterials
Nanomaterial type |
Young's modulus (TPa) |
Tensile strength (GPa) |
Fracture toughness (MPa m0.5) |
Ref. |
Monolayer graphene |
1 |
130 |
4–5 |
9 and 42 |
Monolayer GO |
0.2 |
28.5 and 35.3 for hydroxyl rich and epoxide rich GO respectively |
1.0 and 1.16 for hydroxyl rich and epoxide rich GO respectively |
43 and 44 |
Monolayer rGO |
0.25 |
0.9 |
2.8–3 |
45
|
SWCNTs |
1 |
175 |
2.7 |
46 and 47 |
MWCNTs |
0.95 |
63 |
|
48
|
MoS2 |
0.33 (5–25 layers) |
23 (monolayer breaking strength) |
|
49 and 50 |
WS2 |
0.27 |
|
|
51
|
BN |
1.16 (t = 15 nm) |
|
|
52
|
2.2 Inorganic 2D materials
As compared to their bulk equivalent, 2D materials often possess unique properties due to their high aspect ratio and tunable surface properties. They are known to be mechanically robust (Table 1). Researchers are extensively studying other layered 2D materials having structural similarity to graphene to explore their unusual properties and applications. Falling in this category of 2D materials are transition metal dichalcogenides (TMDs),29 hexagonal boron nitride (hBN),30 graphitic carbon nitride,31 2D black phosphorus,32 transition metal carbides, nitrides and carbonitrides (MXenes),33 layered double hydroxides (LDHs),34 2D metal oxides/sulphides,35 2D metals,36 2D polymers,37 2D metal–organic frameworks,38 2D covalent–organic frameworks39 and 2D perovskites.40 Among these 2D nanomaterials, TMDs, hBN and MXenes have been used to synthesize polymer composites,8,41 particularly epoxy composites.
2.3 Functionalization of the filler: purpose and role
Nanomaterials can be functionalized using two ways: covalent and non-covalent routes. Covalent functionalization routes of graphene and its derivatives involve covalent bond formation between organic compounds and either the C
C bond of pristine graphene or oxygen groups of GO. Noncovalent functionalization aims to control the restacking and agglomeration tendency of graphene and improve its dispersion without interfering with graphene's inherent electronic structure. It involves attaching functional groups to graphene surfaces by interactions like van der Waals, ionic, π–π interactions, etc.53
Graphene is hydrophobic and hence disperses in very few solvents. Functionalization improves its compatibility with organic solvents and polymer matrices,54 which is crucial for the fabrication of nanocomposites. Additionally, these functional groups/compounds can impart new properties to graphene or enhance its inherent properties.
Regarding the epoxy matrix, in particular, functionalized fillers have shown a catalytic effect on the epoxy curing reaction. Functional groups such as amine, carboxyl, hydroxyl, etc., are known to lower the curing activation energy and enhance the cross-linking of the epoxy resin.55,56 The amine groups, in particular, tend to act as secondary hardening agents, thereby strengthening the epoxy–filler interface.57–59 The strong filler–matrix interface contributes to improved mechanical properties, as seen in the coming sections.
The uniform dispersion of graphene in the epoxy matrix is challenging due to its large specific surface area and the tendency to restack and agglomerate. The most popular solution for this problem is the surface modification of graphene. Researchers have widely experimented around modifying the surface of graphene with surfactants,60–62 amines,63–65 silanes,66,67 oxygen groups68,69 and polymers.70–72 Surface modification of graphene allows the filler to have covalent and non-covalent interactions with the matrix, which helps achieve uniform dispersion. When large polymer groups are attached to the filler's surface, the restacking of graphene sheets is reduced due to the steric hindrance produced by the polymer chains. Graphene has also been used in combination with other nano-fillers such as CNTs,73–75 rubber,76,77 inorganic78,79 and organic nano-particles,80,81 inorganic 2D materials,82etc. to make hybrid fillers for epoxy composites. The introduction of organic and inorganic nanoparticles between graphene sheets is known to reduce their restacking tendency, thereby synergistically improving the filler matrix interaction and contributing towards improved mechanical and thermal properties.
Due to its ease of synthesis and high yield, graphene oxide (rather than graphene) is often used as the base filler material for polymer nanocomposites. The presence of oxygen groups on the surface of GO provides ample opportunity for the surface to be effectively functionalized further using amines or polymers. However, that is not the case with inorganic 2D nano-sheets. In the case of epoxy composites, TMDs functionalized with amines,83 surfactants,84 and silanes85 have been reported. However, compared to functionalized graphene, fewer reports are available on surface functionalized inorganic 2D nanosheets to reinforce epoxy composites.
Yet, surface functionalization of TMDs, inspired by the functionalization routes used for graphene oxide, has been studied by some researchers. Depending on the desired end application, the functionalizing species can be customized, and the surface of the TMDs can be fine-tuned to achieve specific surface interactions. Presolski et al.86 have classified TMD functionalization methods into two categories: thiol/sulphur functionalization87,88 and nucleophilic reactivity.89 Covalent functionalization of TMDs is commonly done at defect sites using electron transfer reactions. Surface modified TMDs display increased compatibility with organic solvents. Functionalization of TMDs improves their dispersion and exfoliation and brings about dramatic changes in their inherent mechanical, catalytic, optical and electrical properties.
3. 2D nanosheet filled epoxy composites: fabrication and properties
This section will focus on the effect of 2D nano-sheets on the mechanical properties of epoxy composites. Before the discovery of graphene, several other filler materials were popularly used to reinforce epoxy. These include rubber, core–shell rubber, rigid inorganic nanoparticles, CNTs, etc.Table 2 summarizes the advantages and limitations of the various fillers used to synthesize epoxy composites. The several advantages that graphene offered over the other fillers attracted the attention of the research community.
Table 2 Merits and demerits of various types of fillers used to reinforce epoxy
Filler type |
Size range |
Merits |
Demerits |
Ref. |
Rubber/CSR |
Rubber: μm |
Large improvement in fracture toughness of epoxy composites |
Lower tensile and thermal properties and increased viscosity of composites. No control over the size of rubber because it phase separates during curing. Very high loading (approx. 10–30 vol%) |
90–92
|
CSR: sub-μm |
Rigid inorganic nanoparticles |
Diameter: nano |
High inherent thermal stability and modulus. Improved fracture toughness (less than rubber) and tensile properties in epoxy composites |
Agglomeration issues. High nanoparticle loading (1–20 vol%) is required to improve the mechanical properties. Processing is difficult due to the increased viscosity of epoxy composites |
11, 93 and 94 |
CNTs |
Diameter: nano |
High aspect ratio, inherently high tensile properties of CNTs. Low loading (<5 wt%) results in improved mechanical properties in epoxy composites |
Unbundling/separating CNTs is challenging. Maintaining the aspect ratio is tough |
10, 95 and 96 |
L: μm |
Graphene and 2D nanofillers |
t: nano |
Graphene has the best inherent strength and stiffness among nanomaterials. At low loading (0.04 wt%), thermal and mechanical properties both improve in epoxy composites |
Dispersion in epoxy is difficult due to restacking, folding, agglomeration issues. Surface modification improves dispersion but creates defects, lowering the inherent strength |
9, 97 and 98 |
L: μm |
3.1 Graphene epoxy composites
3.1.1 Graphene/rGO epoxy composites.
A vast amount of literature is available on epoxy reinforced with graphene and its derivatives. Due to epoxy resin's brittle nature, major emphasis has been made on improving the fracture toughness of epoxy composites. Graphene platelets have been a popular reinforcing agent for epoxy resin for more than a decade. Moreno et al.99 customized thermal reduction cycles of GO to study the effect of thermally reduced GO (TRGO) on the mechanical and electrical properties of epoxy composites. GO was thermally reduced at three different temperatures 700 °C, 1000 °C and 2000 °C. The best mechanical properties were reported for 2 wt% TRGO-700/epoxy composites with 16% higher E than the neat resin. More oxygen groups were retained on the surface of TRGO when reduced at 700 °C as compared to 2000 °C, thereby improving filler-matrix interaction. TRGO-2000 contains fewer defects because of the rehybridization of sp3 C to sp2 C, to a large extent restoring the graphitic structure. As a result, it is more suitable for electrical applications. Wu et al.100 reinforced a blend of bi-functional epoxy resin using multilayer graphene nano-platelets (GnPs). An external electric field was employed to align the graphene flakes (Fig. 3). At 1.5 wt% loading, the GIC improved by 891% when graphene flakes were aligned perpendicular to the crack propagation direction. An improvement of 681% in GIC was observed for 2 wt% loading, in the case of randomly oriented graphene flakes. The thermal and electrical conductivities also increased substantially. Rafiee et al.101 compared the effect of graphene platelets (GPLs) and CNTs on the mechanical properties of epoxy composites and concluded that GPLs performed better. 0.1 wt% GPL/epoxy composites showed 53%, 31% and 40% improvement in mode I fracture toughness (KIC), Young's modulus (E) and ultimate tensile strength (UTS) as compared to neat epoxy, respectively. The superiority of GPLs over CNTs was attributed to the large specific surface area, planar geometry and better interlocking with the epoxy matrix due to the wrinkled morphology of GPLs. Wei et al.102 reported an improvement of 13% in UTS and 30% in storage modulus for 0.3 wt% graphene/epoxy composite.
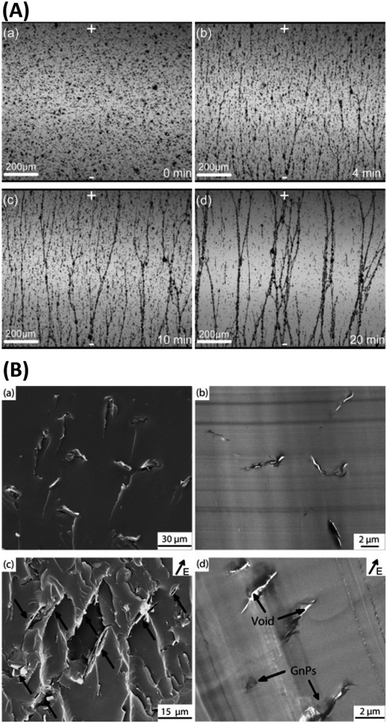 |
| Fig. 3 (A) Optical microscopy images of aligned GnPs in GnP/epoxy composite before (a) and after (b, c and d) applying an electric field; (B) SEM (a and c) and TEM (b and d) images of the epoxy nanocomposites showing randomly oriented and aligned GnPs in epoxy composites.100 Reprinted with permission from ref. 100 (Copyright © 2015, Elsevier). | |
Chandrasekaran et al.103 compared the toughening effects of three nanofillers on DGEBA. It was observed that at 0.5 wt% MWCNT, GNP and TRGO loading, epoxy composites displayed 8, 24 and 40% improvement in fracture toughness, respectively. The performance of TRGO was the best due to the increased filler-matrix interaction among the three fillers. Tang et al.104 prepared rGO/epoxy composites using two dispersion techniques, one by solution mixing using acetone and second by employing planetary ball milling. The latter gave rise to a well-dispersed filler, confirmed using TEM and TOM images. For 0.2 wt% well-dispersed rGO loaded epoxy, KIC improved by 52% and glass transition temperature (Tg) increased by 11 degrees compared to neat epoxy.
3.1.2 GO/epoxy composites.
As mentioned previously, oxidation of graphite attaches hydroxyl, carboxyl and epoxide groups to the surface of the sheets, facilitates exfoliation and makes them compatible with a range of polar solvents and polymers. As a result, plenty of research has been conducted related to the mechanical and thermal properties of GO/epoxy composites. Yang et al.105 reported remarkable improvement in compressive failure strength and toughness by 48.3 and 1185.2%, respectively, at a mere loading level of 0.0375 wt% GO to the epoxy matrix. The reason behind the improved properties was uniformly dispersed GO because the GO/epoxy composites were prepared by a two-phase extraction process using an aqueous dispersion of GO. Rafiee et al.106 reinforced epoxy with GO prepared from oxidation of graphite flakes. Remarkable improvement of 65%, 115%, 50% and 45% was observed in KIC, GIC, E and UTS at a mere 0.125 wt% GO loading as compared to the base resin, respectively. The reported fatigue properties showed a 25 times lower da/dN value for the nanocomposite than the base epoxy. These improvements were attributed to the doubling of surface roughness when GO loading was increased from 0 to 0.125 wt%. On increasing the loading further, the roughness effect appeared to saturate. Bortz et al.107 synthesized graphene by opening helical carbon nanofibers to obtain graphene nanoribbons and further oxidized it to obtain GO. Remarkable tensile strength and modulus of 73.57 MPa and 3.32 GPa at 0.5 and 0.1 wt% GO were reported, respectively. KIC and GIC improved by 62% and 110%, respectively, for 1 wt% GO/epoxy composites as compared to neat epoxy. Flexural modulus and strength improved by 12% and 23%, respectively, at 1 wt% GO loading. Muñoz et al.108 reported 39% and 13% improvement in compressive elastic modulus and flexural modulus, respectively, for 0.3 wt% commercially available GO-loaded epoxy. Xue et al.98 reported an improvement of 10%, 9% and 56% in E, UTS and lap shear strength at 1 wt% GO loading in epoxy, respectively. The Tg also improved by over 4 degrees at 1 wt% GO loading. Kang et al.109 reported 80% and 98% higher impact toughness and KIC at 1 wt% GO loading in epoxy, respectively. A green solvent-free filler dispersion method was adopted by Tang et al.,110 wherein GO was phase transferred from its aqueous dispersion into epoxy resin (E51) using triglycidyl para-aminophenol (TGPAP) as the functionalization and phase transfer agent (Fig. 4). This dispersion method is easy to scale up and ensures uniform filler dispersion. A blend of E51 and 20 wt% TGPAP containing 1 wt% GO showed an improvement in the storage modulus, tensile strength and toughness by 43%, 92% and 126% compared to the neat resin, respectively. The flexural strength, modulus and micro-hardness of the above composite were 37%, 38% and 137% higher than the neat resin, respectively. Wei et al.69 systematically studied the effect of the extent of oxidation of GO sheets on the mechanical properties of GO/epoxy composites. Various batches of GO were synthesized using the Hummers method by varying the concentration of KMnO4 and reaction time. TEM results confirmed that the best dispersion was observed for the GO 4 batch consisting of the following reactant concentrations: 1 g graphite, 1 g NaNO3, 6 g KMnO4 and 48 ml H2SO4 reacted for 6 hours. The 0.2 wt% GO4/epoxy composite showed 56% and 128% improvement in KIC and GIC compared to the neat resin, respectively. It was concluded that the extent of oxidation of graphene affects the covalent bonding between the GO and epoxy matrix. Hence, an optimal level of oxidation is required to achieve the best filler-matrix interaction and, in turn, the best mechanical properties.
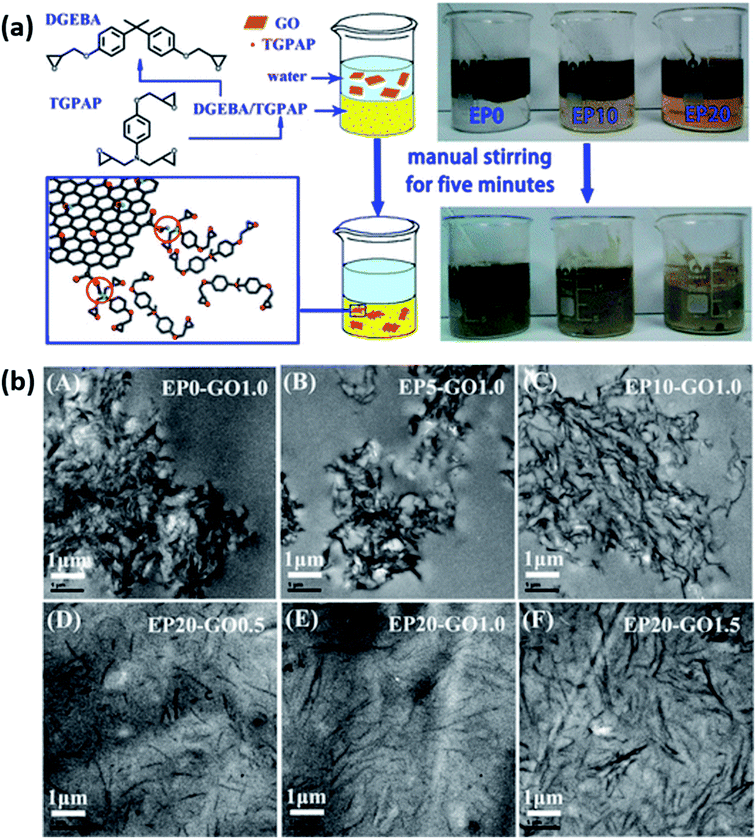 |
| Fig. 4 Schematic and digital images of composites fabricated by the phase transfer method (a); TEM images of nanocomposites at various concentrations of the filler (b).110 Reprinted with permission from ref. 110 (Copyright © 2016, American Chemical Society). | |
3.1.3 Amine-GO/epoxy composites.
Amine functionalized graphene derivatives are a common filler material for epoxy composites. The availability of primary amine groups on the surface of the filler allows it to undergo favorable covalent interactions with the epoxy matrix, thereby strengthening the filler–matrix interface. Ferreira et al.111 used hexamethylene diamine functionalized GO (AGO) to reinforce epoxy. In the case of 1 wt% AGO/epoxy composite 33% and 25% higher hardness and storage modulus were reported as compared to neat epoxy, respectively. Chatterjee et al.65 used dodecyl amine (DDA) functionalized expanded graphene nano-platelets (EGNPs) to reinforce epoxy and reported a 66% higher KIC for 0.1 wt% EGNP/epoxy composites as compared to neat epoxy. Flexural modulus increased by 15% at 2 wt% loading due to improved load transfer (Fig. 5). Ashori et al.112 used GO functionalized with three different amines: ethylenediamine (EDA), 4,4′-diamino diphenyl sulfone (DDS) and p-phenylenediamine (PPD) as a filler for carbon fiber reinforced epoxy. The evaluation of fracture surface morphology indicated that the interfacial interaction was strengthened due to the introduction of functional groups on the surface of GO. Fang et al.113 reported 60% and 53% improvement in E and UTS of 0.4 wt% methylene dianiline (MDA) modified rGO/epoxy composites, respectively. The fracture toughness and flexural strength improved by 94% and 92% for 0.6 wt% filler loading, respectively. Seong et al.114 reported 120% and 63% improvement in impact toughness and storage modulus in 1.5 phr MDA modified GNP/epoxy composites compared to 86% and 39% improvement in 1.5 phr GNP/epoxy composites, respectively. Naebe et al.115 compared the reinforcing effects of thermally reduced GO (TRG), and TRG modified with the Bingel reaction (FG) on epoxy composites. It was reported that 0.1 wt% TRG and FG/epoxy composites show 15% and 22% higher flexural strength and 6% and 16% higher storage modulus than neat epoxy, respectively. Guo et al.116 reported that GO modified with triazine derivatives (GO–TCT–DETA) dispersed more uniformly in epoxy matrix whereas blank GO agglomerated. A 49% and 15% higher flexural strength and modulus was reported for 0.1 wt% GO–TCT–DETA/epoxy composite as compared to neat epoxy, respectively.
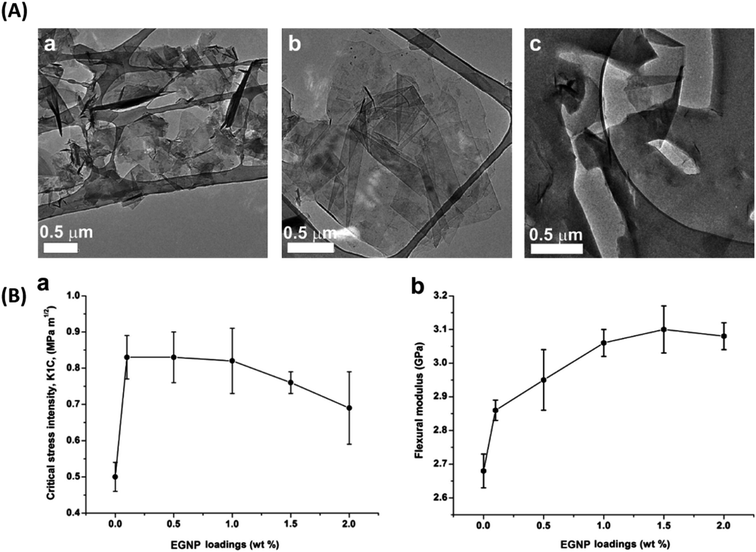 |
| Fig. 5 (A) TEM images of amine-EGNPs (a and b) and 0.5 wt% amine-EGNP/epoxy composite (c). (B) Mechanical properties of epoxy composites with different EGNP loadings.65 Reprinted with permission from ref. 65 (Copyright © 2012 Elsevier B.V.). | |
3.1.4 Silane-GO/epoxy composites.
Many authors have reported promising results for epoxy reinforced with silane-modified graphene derivatives117 to enhance the corrosion resistance,118,119 mechanical and thermal properties of epoxy composites. Wan et al.66 reported that superior dispersion of silane-f-GO in the epoxy matrix was achieved compared to GO. Fig. 6A shows a schematic of GO functionalization using glycidoxypropyltrimethoxy silane (GPTMS). The TEM image of silane-f-GO appeared darker than that of GO indicating attachment of functional groups to the surface of GO. The storage modulus increased by 52% for 0.5 wt% silane-f-GO/epoxy composite indicating that the silane groups improved the filler matrix interaction and increased the stiffness of epoxy (Fig. 6B). The tensile and fracture properties also improved. Li et al.120 compared the tensile and fracture properties of epoxy reinforced with amino-silane functionalized GO (APTS-GO), and epoxy-silane functionalized GO (GPTS-GO). The authors concluded that GPTS-GO was more uniformly dispersed than APTS-GO, but the latter offered better interfacial stress transfer. Hence, depending on the silane groups attached to GO, the properties of the final epoxy composites can be customized. Moghadam et al.121 reported that 0.5 wt% G–Si/epoxy composites showed 38% and 14% improvement in UTS and E, respectively. KIC increased by 86% for 0.5 wt% G–Si/epoxy composites as compared to neat epoxy.
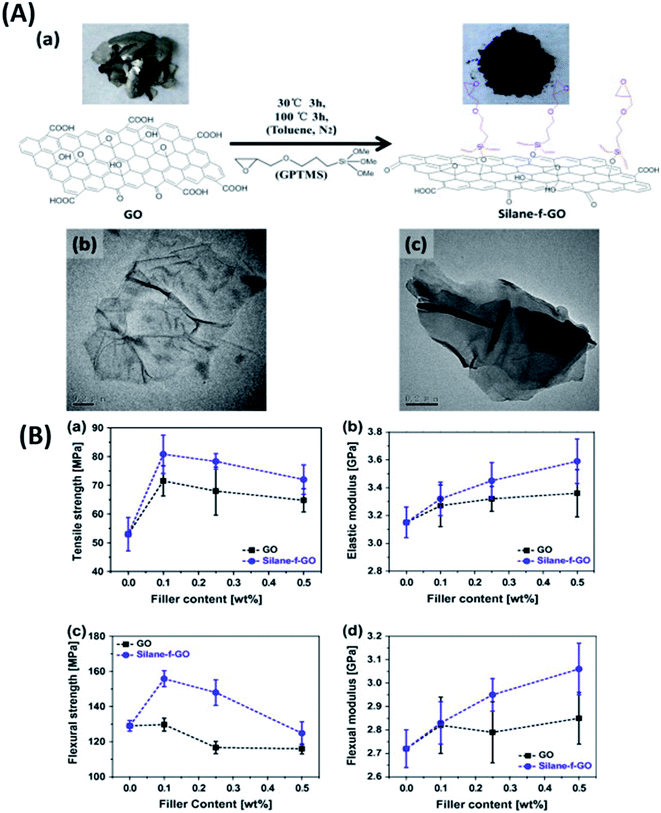 |
| Fig. 6 (A) Synthesis route schematic of silane functionalized GO (a) and TEM images of GO (b) and silane-f-GO (c) and (B) mechanical properties of epoxy nanocomposites (a–d).66 Reprinted with permission from ref. 66 (Copyright © 2014 Elsevier Ltd). | |
3.1.5 Polymer-g-GO/epoxy composites.
In comparison to the other covalent functionalization methods of graphene, surface modification using polymer chains is promising because it is cost-effective and efficient.122,123 Wan et al.70 successfully reinforced diglycidyl ether of bisphenol-A (DGEBA) resin with DGEBA grafted GO and reported enhanced tensile and fracture properties. The use of the matrix as a functionalizing species enhanced the filler dispersion and filler matrix interaction. Similar studies were reported by Zhao et al.124 Recently, researchers71,125 studied the effect of hyperbranched polyamide (HBPA) functionalized GO on epoxy composites. Li et al.71 used amine-terminated HBPA instead of blank HBPA to functionalize GO. Due to the amine groups on HBPA, Li et al. reported improvement in tensile properties at a mere 0.15 wt% loading compared to 0.5 wt% HBPA loading in the case of Qi et al.125 Apart from the tensile and thermomechanical properties, flexural strength and modulus increased by 43% and 97% for 0.15 wt% GO–HBPA–NH2 loading in epoxy respectively.71
In another recent study, Mi et al.126 modified GO with hyperbranched polyamide (HPA). Impact strength, tensile strength, flexural strength and flexural modulus for 0.10 wt% HPA–GO/epoxy composite increased by 310%, 37%, 8% and 10% compared to neat epoxy, respectively. The interfacial properties of the filler were quantified using contact angle measurements. Interestingly, it was reported that after HPA grafting, the water contact angle of GO increased, making it hydrophobic, but its DGEBA contact angle reduced, lowering the interfacial energy. Guan et al.127 used two types of polyetheramine with different chain lengths (D230 and D2000) to modify the surface of GO. The UTS improved by 63% and 51%, E by 12% and 10% and tensile toughness by 90% and 119% for 0.5 wt% D230-g-GO and D2000-g-GO/epoxy composites, respectively. The authors proved that the interphase between the filler and the matrix could be tuned by grafting polymer chains of different lengths on the surface of GO. Zhao et al.128 reported a method of in situ polymerization to graft polyamide 6 (PA6) chains on GO surface. The thickness of GO was observed to increase substantially after functionalization. As a result of functionalization, the surface of PA6–GO appeared rougher than that of GO due to the introduction of defects (Fig. 7A). The author reported 53% higher KIC for 1.5 wt% GO–PA6/epoxy composite as compared to the neat resin (Fig. 7B). The PA6–GO filler contained 1 wt% GO, hence it's noteworthy that the 1.5 wt% GO–PA6/epoxy composite contained only 0.015 wt% GO. Pan et al.129 used perylene bisimide (PBI) as a foundation for oligomerization of glycidol to prepare hyperbranched glycidol (HPG). This polymer, then mounted on rGO, was further used to synthesize epoxy composites. UTS, impact strength and impact toughness increased by 62%, 51% and 148% for 0.7 wt% PBI–HPG–rGO/epoxy composite as compared to the neat resin, respectively. Ma et al.130 modified GO using hydroxyl-terminated triazine derivatives (GO–TCT–Tris). Flexural strength and modulus increased by 49% and 16% for 0.1 wt% GO–TCT–Tris/epoxy composite than the neat resin, respectively. Table 3 summarizes the mechanical properties of epoxy composites reinforced with graphene and its derivatives.
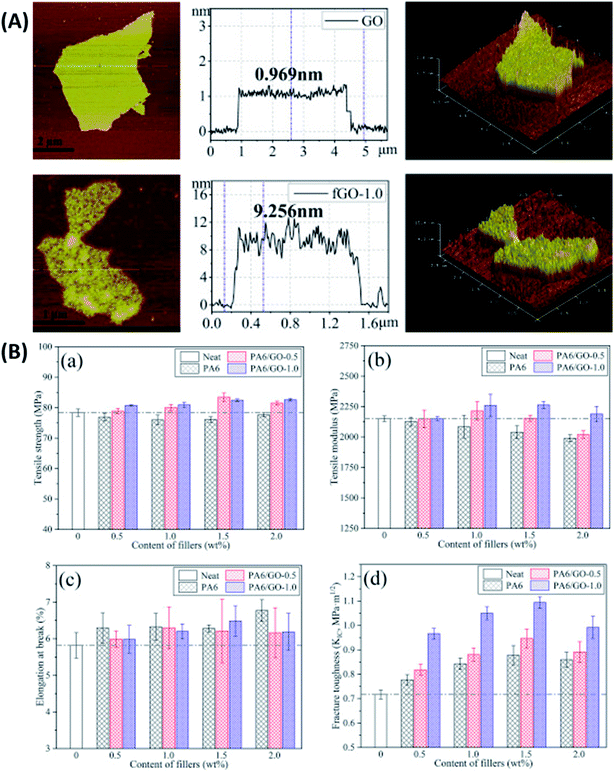 |
| Fig. 7 (A) AFM images and sheet thickness analysis of GO (a) and PA6–GO (b) and (B) tensile (a–c) and fracture (d) properties of epoxy composite.128 Reprinted with permission from ref. 128 (Copyright © 2018 Elsevier Ltd). | |
Table 3 Percentage improvement in UTS, E and KIC of graphene epoxy composites
Resin and hardener |
Filler |
Dispersion method |
UTS (%) |
E (%) |
K
IC (%) |
Ref./year |
Name |
Size |
Loading |
DGEBA and DETDA 100 : 24.4 |
GO-g-PEG |
|
0.1 wt% |
Acetone, sonication |
|
|
334 |
2020 (ref. 131) |
E44 and DDS (26 : 7.8) |
Polycaprolactone–graphene |
|
1 wt% |
Acetone, sonication |
87 |
|
|
2020 (ref. 132) |
DGEBA and DETDA 100 : 24.4 |
GO |
|
0.5 wt% |
Acetone, sonication |
25 |
|
90 |
2020 (ref. 133) |
GO–TBCP |
Miscelle dia = 6–11 nm |
0.5 wt% |
32 |
|
397 |
DGEBA and aliphatic amine |
PA6/GO |
t = 9.25 nm |
1.5 wt% |
Filler added to the hardener, sonication |
|
5 |
53 |
2019 (ref. 128) |
E51 DGEBA and hardener |
GO-2021P |
t = 8.33 nm |
1 wt% |
TRM |
34 |
26 |
|
2019 (ref. 134) |
DGEBA and DDM |
Acid treated graphene |
t = 1 nm |
0.2 wt% |
Ethanol, sonication |
14 |
|
|
2019 (ref. 135) |
Araldite MY 721 and Aradur 9664 |
GO-g-PAA |
|
0.7 wt% |
Ethanol, sonication |
|
|
87 |
2019 (ref. 72) |
Epoxy E 51 and DDM H-256 |
GO |
|
0.1 wt% |
Acetone, sonication |
27 |
14 |
|
2019 (ref. 130) |
GO–TCT–Tris |
|
0.1 wt% |
41 |
28 |
|
E51 and D230 in the ratio 100/30 |
HBPA–GO |
3–5 layers |
0.15 wt% |
Filler added to the hardener, sonication |
42 |
|
|
2019 (ref. 71) |
DGEBA E44 and DDS |
GOFO |
|
0.5 wt% |
THF by sonication |
20 |
12 |
|
2019 (ref. 136) |
Epoxy 5417A 2 : 1 |
GO |
|
0.2 wt% |
Filler added to the hardener, sonication |
16 |
6 |
56 |
2018 (ref. 69) |
DGEBA WSR618 and QS-1622 (2 : 1) |
HBPA–GO |
|
0.5 wt% |
THF by sonication |
23 |
18 |
44 |
2018 (ref. 125) |
YD-128 and hardener |
GNP |
L = 10 μm |
2 wt% |
Acetone, sonication |
6 |
66 |
|
2018 (ref. 137) |
Melamine fGNP |
L = 10 μm |
2 wt% |
34 |
95 |
|
Araldite LY 1564 and Aradur 3487 1 : 0.34 |
GO |
|
0.25 wt% |
Filler added to the hardener, sonication |
15 |
13 |
|
2018 (ref. 68) |
DGEBA E51 and H-256 hardener |
GO |
L = few μm |
0.1 wt% |
Acetone, sonication |
26 |
15 |
|
2018 (ref. 116) |
GO–TCT–DETA |
|
0.1 wt% |
41 |
28 |
|
Lapox-B-11 and Lapox AH-713 2 : 1 |
TZG |
|
0.1 wt% |
DMF, sonication |
32 |
35 |
133 |
2018 (ref. 138) |
DER 354 and DETDA |
DG |
t = 1.2 nm L = 1 μm |
0.8 wt% |
Acetone, sonication |
37 |
|
|
2017 (ref. 139) |
RL 440 and t HY 441 in 100 : 20 |
Ppy–GO |
|
1.5 wt% |
Acetone, sonication |
51 |
84 |
|
2017 (ref. 140) |
EPOLAM 2063 and hardener 100/107 |
GO |
|
0.5 wt% |
Ethanol, sonication |
3 |
|
19 |
2017 (ref. 141) |
HPEEK–GO |
|
0.5 wt% |
7 |
|
31 |
YD128 and DDM |
GO |
L = 0.25–5 μm, t = 0.927 nm |
1 wt% |
Acetone, sonication |
|
|
98 |
2017 (ref. 109) |
NPEL-128S and F205 in 100 : 58 |
GO–ODA |
|
0.5 wt% |
Acetone, sonication |
104 |
97 |
|
2017 (ref. 142) |
Araldite LY 564 and Aradur 2954 100/35 |
Plasma-fGNP |
L = 1–3 μm |
0.25 wt% |
Methanol, sonication |
2 |
5 |
22 |
2017 (ref. 143) |
YD128 |
GO |
|
0.4 wt% |
Acetone, sonication |
168 |
104 |
|
2017 (ref. 144) |
Epofix resin and hardener 25 : 3 |
G-EP |
t = 2.2 nm |
1 wt% |
DMF, sonication |
116 |
96 |
|
2016 (ref. 124) |
DGEBA YD-128 and DDS 100 : 21 |
DDS–GO |
t = 1 nm, L = 100–500 nm |
0.1 wt% |
Filler acted as a co-curing agent |
26 |
|
|
2016 (ref. 63) |
LY5052 and HY5052 (100/38) |
DC-GR |
t = 2.13 nm |
0.2 wt% |
Acetone, sonication |
56 |
26 |
|
2016 (ref. 145) |
DGEBA/MHHPA (180/175) |
TRGO |
|
0.2 wt% |
Ethanol, planetary milling |
|
|
52 |
2015 (ref. 61) |
Triton-TRGO |
|
0.2 wt% |
52 |
|
65 |
Epon 828 and hardener (100 : 14.5) |
ATBN-GNP |
L = 5 μm, t < 10 nm |
5 wt% |
Acetone, sonication |
|
|
93 |
2015 (ref. 146) |
E51 and TGPAP blend |
GO |
|
1.5 wt% |
Phase transfer method |
92 |
|
|
2015 (ref. 110) |
DER-331 and DEH 24 (100/15) |
GO–TEPA |
t = 1.5 nm |
0.5 wt% |
TRM |
|
72 |
|
2015 (ref. 64) |
Araldite F and DDM |
M25 graphene |
L = 25 μm, t = 6 nm |
5 wt% |
Filler added to resin, sonicated |
|
28 |
|
2015 (ref. 147) |
E-44 and DDS (3.33) |
PBI–HPG–RGO |
t = 16 nm |
0.7 wt% |
Acetone, sonication |
62 |
|
|
2015 (ref. 129) |
DGEBA/MHHPA in 180/175 |
Silane fGO |
L = 1–3 μm |
0.1 wt% |
Acetone, planetary milling |
|
|
39 |
2014 (ref. 66) |
|
|
0.25 wt% |
48 |
10 |
|
Epon 828 and D230 |
ATBN–GO |
|
0.04 wt% |
Filler added to the hardener, sonication, |
|
|
50 |
2014 (ref. 97) |
DGEBA/MHHPA in 180/175 |
GO |
L = few μm |
0.5 wt% |
Acetone, sonication, planetary milling |
22 |
|
|
2014 (ref. 127) |
D 230-f-GO |
|
0.5 wt% |
63 |
12 |
|
D 2000-f-GO |
|
0.5 wt% |
51 |
10 |
|
Araldite GY 191 and HY 956 |
GO with CF |
L = 2 μm |
0.1 wt% |
Acetone, sonication |
|
27 |
54 |
2014 (ref. 148) |
Araldite LY556 and Aradur 917 100/90.1 |
GNP |
t = 12–15 nm L = 20–50 μm |
1.0 wt% |
TRM |
|
|
43 |
2014 (ref. 103) |
DGEBA/MHHPA 180/175 |
GO |
t = 0.486 nm |
0.25 wt% |
Acetone, sonication, ball milling |
28 |
5 |
26 |
2014 (ref. 70) |
DGEBA-g-GO |
t = 0.865 nm |
0.25 wt% |
75 |
13 |
41 |
KEM 101 and KH 700 in 5 : 1 |
In situ rGO |
Area: 176.4 μm2 |
1.5 wt% |
Aqueous phase transfer |
500 |
70 |
|
2013 (ref. 149) |
DGEBA and MHHPA |
rGO |
t = 1.23 nm |
0.2 wt% |
Ethanol, sonication, ball milling |
|
|
52 |
2013 (ref. 104) |
LY5052 and HY5052 (100 : 38) |
APTS-GO |
|
0.2 wt% |
Acetone, sonication |
16 |
32 |
|
2013 (ref. 120) |
GPTS-GO |
|
0.2 wt% |
|
|
72 |
DGEBA and hardener |
APTS-rGO |
t = 2.696 nm |
1 wt% |
Acetone, sonication |
45 |
|
|
2012 (ref. 67) |
Epikote Epikure 100/24 |
DDA–graphene |
|
0.1 wt% |
Acetone, TRM |
|
|
66 |
2012 (ref. 65) |
DGEBA and MDA (100/27) |
AB-graphite |
2–15 layers |
4 wt% |
Ethanol, sonication |
30 |
40 |
|
2011 (ref. 150) |
Resoltech 180/1805 |
GO |
L = 2 μm |
0.5 wt% |
Acetone/TRM |
13 |
5 |
63 |
2011 (ref. 107) |
Epoxy 2000 and 2120 epoxy |
FGS |
t = 2–3 layers |
0.125 wt% |
Acetone, sonication |
45 |
50 |
65 |
2010 (ref. 106) |
Epon 828 |
MDA–GO |
t = 4.83 nm |
0.6 wt% |
Acetone, sonication |
53 |
60 |
94 |
2010 (ref. 113) |
3.2 Inorganic 2D nanosheets/epoxy composites
Graphene is known to impart electrical conductivity to insulating polymers and hence is useful to fabricate electrically conductive polymers. In contrast, TMDs are high bandgap semiconductors and hence incapable of imparting electrical conductivity to polymers. As a result, they are useful in synthesizing epoxy composites with enhanced mechanical properties while maintaining the polymer's electrically insulating nature.
Eksik et al.151 used unmodified MoS2 nanoplatelets (MNPs) to reinforce epoxy and reported improvement in tensile and fracture properties. An improvement of 32%, 60% and 160% was reported in UTS, KIC and GIC of 0.2 wt% MNP/epoxy composite over neat epoxy, respectively. A significant improvement of 13 degrees was observed in the Tg of the same composite compared to the neat resin. Since the author used unmodified MNPs, agglomeration was observed at very low wt% (0.3 wt%) in comparison to the surface-modified fillers. Zhao et al.85 reported a 66% improvement in impact intensity at 0.7 wt% loading of silane-modified boron nitride in the epoxy matrix. An 80% improvement was reported in the apparent shear strength at 120 °C for the same composite. In another study152 the author has established a relationship between the degree of exfoliation of MoS2 and its influence on the mechanical properties of epoxy composites. The degree of exfoliation was controlled using the intercalation time. It was reported that the well-exfoliated sheets led to an enormous 500% and 6800% increase in the UTS and E of 1 wt% MoS2/epoxy composite, respectively. Sahu et al.8 reported a 43% and 65% improvement in compressive and flexural strength for epoxy reinforced with 0.25 wt% PEI modified WS2. Fig. 8A brings out the difference between the bulk and exfoliated WS2 sheets. The functionalization of WS2 nanosheets improved the filler's load transfer ability and led to an 83% improvement in fracture toughness of epoxy-containing 0.25 wt% filler (Fig. 8B).
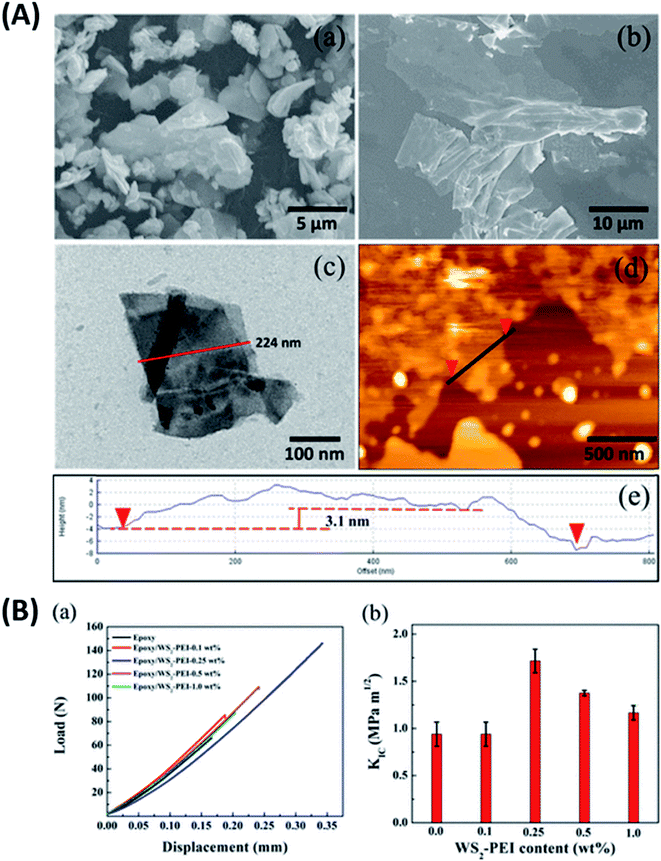 |
| Fig. 8 (A) SEM images of bulk WS2 (a). SEM (b), TEM (c) and AFM (d and e) images of PEI functionalized WS2 and (B) fracture toughness of the PEI–WS2/epoxy composites (a and b).8 Reprinted with permission from ref. 8 (Copyright © 2017, American Chemical Society). | |
Boron nitride displays excellent thermal conductivity and has been widely used to fabricate thermally conducting epoxy composites.153–155 Some researchers have also used hBN to enhance the mechanical properties of epoxy resin. Liu et al.7 reinforced epoxy with (3-aminopropyl)triethoxysilane (APTES) modified boron nitride and reported a 100% increase in tensile strength at 10 wt% loading. Tg increased by 14 degrees for 40 wt% loading. Lee et al.156 compared the reinforcing effects of rGO, and 1-pyrenebutyric acid (PBA) functionalized BNNF (boron nitride nano-flakes). Lee et al.156 showed the filler's TEM image and the statistical analysis of the thickness of BNNFs. The author reported that PBA–BNNF performed much better and reported an improvement of 21%, 54% and 107% in E, UTS and tensile toughness of 0.3 wt% PBA–BNNF/epoxy composites, respectively.
Another class of 2D materials called the MXenes has recently gained popularity as a reinforcement material in epoxy composites.157 Research related to MXene reinforced epoxy is still at a nascent stage. Zhang et al.158 reported a 76% and 66% increase in impact strength and flexural strength for 1 wt% Ti2CTx/epoxy composites, but the hardness dropped. The Tg increased by 20 degrees at 2 wt% loading, with a reduction in creep strain. Table 4 gives a summary of epoxy composites containing inorganic 2D nano-sheets as fillers.
Table 4 Percentage improvement in UTS, E and KIC for epoxy reinforced with inorganic 2D nanofillers
Resin and hardener |
Filler |
Dispersion method |
UTS (%) |
E (%) |
K
IC (%) |
Ref./year |
Name |
Size |
Loading |
W52 and JH93 100 : 25 |
APTES–BNNS |
t = 2–3 nm |
10 wt% |
Hot pressing |
2 |
|
|
2020 (ref. 7) |
EP (m(EPON828)/m(PPGDGE) = 60/40) and D230 |
G–MoS2 |
|
1 wt% |
Acetone, sonication |
500 |
|
|
2019 (ref. 152) |
DGEBF NPEF-170 and DMDC |
KH580-f-MoS2 |
t = 1–6 nm |
0.7 wt% |
THF sonication, TRM |
8 |
22 |
|
2018 (ref. 85) |
DGEBA Lapox-B-11 and TETA Lapox AH-713 (2 : 1) |
CTAB–MoS2 |
|
0.2 wt% |
THF, sonication |
23 |
27 |
|
2018 (ref. 84) |
EP(m(EPON828)/m(PPGDGE) = 55/45) resin and D230 |
Melamine–MoS2 |
|
0.8 wt% |
Acetone, sonication |
400 |
450 |
|
2018 (ref. 159) |
TGDDMM and DDS |
WS2–PEI |
L = 260 ± 45 nm, t = 3 nm |
0.25 wt% |
Ethanol, sonication |
|
|
83 |
2017 (ref. 8) |
E51 and Jeffamine D230 |
AT–hBN |
t = 3–4 nm |
1 wt% |
THF, sonication |
6 |
5 |
|
2016 (ref. 160) |
Epon 862 and Epikure hardener |
MNP |
t = 5–10 nm, L = 400–500 nm |
0.2 wt% |
Sonication in 1-vinyl-2 pyrrolidone |
32 |
|
60 |
2014 (ref. 151) |
Epon 862 and hardener |
PBA–BNNF |
L = 200–500 nm, t < 7 nm |
0.3 wt% |
Acetone, sonication |
54 |
21 |
|
2013 (ref. 156) |
3.3 Hybrid filler/epoxy composites
Hybrid fillers are multiscale fillers used to reinforce polymer matrix materials. The major advantage of hybrid fillers is that each individual filler's inherent properties are exploited synergistically to achieve the desired mechanical properties in the final composite. Apart from enhancing electrical,161,162 thermal163 and corrosion resistance164,165 properties, hybrid fillers also provide unique architecture that is capable of strengthening the filler matrix interface and contribute significantly to the mechanical properties of epoxy composites.
3.3.1 2D–2D hybrids.
Domun et al.74 reported a 41% and 92% improvement in KIC and GIC on the addition of f-GNP (0.25 wt%)/BNNS (boron nitride nanosheets 0.1 wt%) hybrid to the epoxy matrix, respectively. A hybrid MoS2/hBN (1
:
1) filler (Fig. 9A) was used in ref. 166 to reinforce epoxy. A 95% higher UTS and 58% higher E were reported for 1 wt% and 0.25 wt% MoS2/hBN epoxy composite, respectively. The cross-linking density was shown to improve by 45% over the neat resin after the hybrid filler was added to epoxy. This was clear evidence of an improved filler matrix interaction.
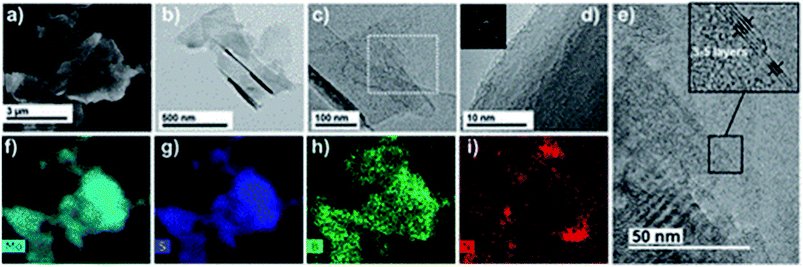 |
| Fig. 9 SEM image (a) and EDS elemental maps of Mo, S, B and N (f–i), TEM and HRTEM images of the MoS2/h-BN hybrid (b–e).166 Reprinted with permission from ref. 166 (Copyright © 2019, American Chemical Society). | |
3.4 Emerging material derived from graphene: aerographene
Aerogels are 3-dimensional porous and interconnected architecture having an ultralow density, superelasticity,167 high specific surface area, high adsorption capacity168 and tunable porosity.169 Graphene and its derivatives can be used as building blocks to make such 3D structures. Graphene aerogels, also commonly known as aerographene, are widely used in polymer composites to enhance the thermal,170,171 electrical,172,173 tribological174,175 and mechanical properties of the polymer. For example, Kim et al.176 reported a maximum improvement of 76% in the fracture toughness of non-oxidized graphite aerogel (NOGA)/epoxy composite at a loading level of 0.45 vol% as compared to neat epoxy. NOGA was synthesized using a bi-directional freezing process to achieve a robust wall of aligned graphene sheets (Fig. 10). Maximum improvement was achieved when the crack propagation direction was perpendicular to the graphene flake wall alignment. A summary of the mechanical properties of aerographene reinforced composites has been provided in the table below (Table 5).
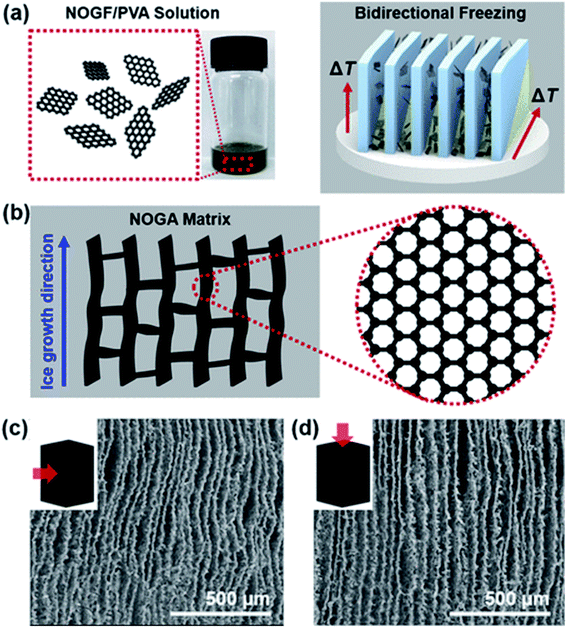 |
| Fig. 10 Schematic of the synthesis of aerographene by bidirectional freezing (a), aerographene structure (b) and aerographene SEM images (c and d).176 Reprinted with permission from ref. 176 (Copyright © 2018, American Chemical Society). | |
Table 5 Percentage improvement in UTS, KIC and flexural modulus for epoxy reinforced with aerographene
Resin |
Filler |
Composite fabrication |
UTS (%) |
K
IC (%) |
Flex mod. (%) |
Ref./year |
Type |
Synthesis |
Loading |
ML-523 and HA-11 |
3D nitrogen doped graphene |
GO + dicyanamide hydrothermal reduction |
0.1 wt% |
Ultrasonic mixing |
19 |
|
|
2020 (ref. 177) |
LY 1556 and XB 3471 100 : 12 |
Ultra large-GA |
Directional freeze drying of GO |
0.11 vol% |
Vacuum infiltration |
|
69 |
|
2018 (ref. 178) |
Small-GA |
0.16 vol% |
|
33 |
|
Epoxy and hardener |
Multilayer graphene web |
CVD on Ni template |
8.3 wt% |
Vacuum infiltration |
|
100 |
|
2018 (ref. 179) |
LY1556 and TETA in 100 : 12 |
Non-oxidized graphene aerogel |
Bi-directional freeze casting in PVA |
0.45 vol% |
Vacuum infiltration |
|
76 |
|
2018 (ref. 176) |
0.34 vol% |
|
|
25 |
Aeromarine 300/21 |
Commercial GF |
|
0.13 wt% |
Mold casting |
12 |
|
|
2017 (ref. 180) |
Rim 135 and Rim 137 100 : 30 |
Aero-graphite |
CVD on ZnO template |
0.45 wt% |
Vacuum infiltration |
|
19 |
|
2016 (ref. 181) |
Epoxy and hardener |
GA |
Reduction using HI, freeze drying |
1.4 wt% |
Vacuum infiltration |
|
64 |
|
2015 (ref. 182) |
0.5 wt% |
|
|
12 |
LY1556 and TETA 100 : 12 |
GF |
CVD on Ni template |
0.1 wt% |
Vacuum infiltration |
|
70 |
|
2014 (ref. 183) |
0.2 wt% |
|
|
53 |
4. Toughening mechanisms
The toughening mechanisms occurring in the composites can be divided into intrinsic and extrinsic.184 The toughening mechanisms induced due to the addition of the nano-filler, such as crack deflection, pull out, delamination etc., are extrinsic toughening mechanisms. In contrast, the matrix itself is capable of causing toughening to some extent by undergoing localized plastic deformation, micro-cracking etc. These mechanisms fall into the intrinsic toughening category. Fig. 11 represents toughening mechanisms in epoxy reinforced with 2D fillers. Depending on the filler added, the predominant toughening mechanisms vary. To date, a number of fillers have been explored for reinforcing epoxy with a focus on enhancing its fracture properties.
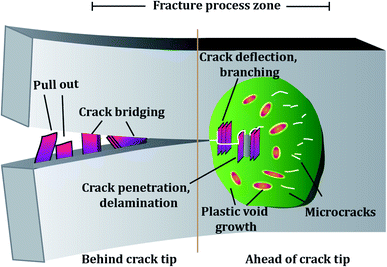 |
| Fig. 11 Toughening mechanisms in epoxy composites reinforced with 2D nanomaterials. | |
The filler's inherent properties, such as high modulus, breaking strength, fracture toughness, etc., also play a major role in contributing towards a toughened matrix. Graphene and TMDs both display exceptional mechanical strength, which reflects in their epoxy composites. The filler size can affect the extent of toughening. Studies showed that the larger the GO sheet, the more the wrinkles and the more defects associated with the GO sheets. These defects act as stress concentrators and hence lower the fracture toughness. In contrast, smaller sheets have fewer defects and can provide better load transfer and reinforcing capability.185 Apart from the filler size, filler alignment also plays a role. As compared to random and parallel arrangement, graphene nanoplatelets transversely aligned to the crack growth contribute towards toughening due to increased interaction of the crack with the filler, eventually causing graphene pull-out.100
The major toughening mechanisms that underplay in graphene–epoxy composites are crack deflection,71,186 crack pinning,71,72 micro-crack formation,187 and delamination103 (Fig. 12). Crack pinning is possible only if the crack tip size is smaller than the filler size. Hence, it is rarely observed in the case of nano-fillers but has been reported by some authors.138,143 The high aspect ratio of graphene sheets coupled with strong filler-matrix interaction causes the crack to change its path leading to crack deflection. The crack continues to propagate after undergoing deflection, but now the crack propagation occurs at different heights. This leads to off-plane loading conditions, generating a coarse, multiplane fracture surface area, thereby contributing to enhanced fracture properties.103,107 When crack deflection occurs, the crack tends to tilt and twist and crack growth occurs in mixed mode. As a result, more fracture energy is spent than only mode I manifesting as a higher fracture toughness in the graphene-based epoxy composite.106,188
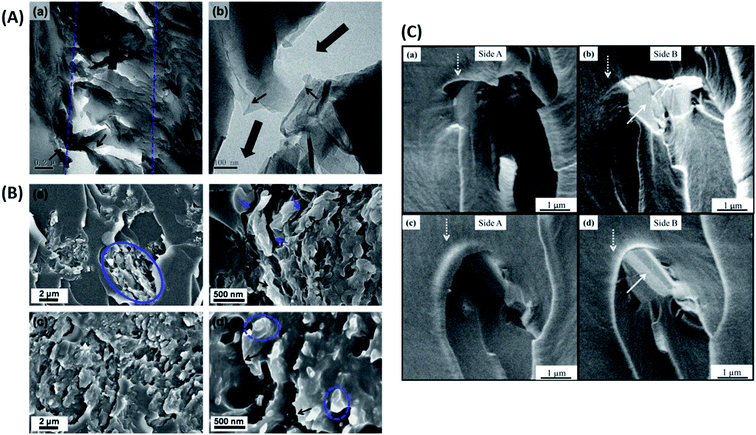 |
| Fig. 12 (A) TEM images of nanocomposites displaying crack bridging (a and b), crack deflection (b) and delamination (b) of GO.66 Reprinted with permission from ref. 66 (Copyright © 2014 Elsevier Ltd). (B) SEM images of fractured epoxy composite samples showing poor adhesion in the case of graphene/epoxy (a and b) and strong adhesion in Triton–graphene/epoxy (c and d).60 Reprinted with permission from ref. 60 (Copyright © 2013 Elsevier Ltd). (C) Toughening mechanisms in epoxy composites containing GNPs (a and b) and TRGO (c and d), the white arrow indicates the delaminated surface of the nano-filler.103 Reprinted with permission from ref. 103 (Copyright © 2014 Elsevier Ltd). | |
As was highlighted above in Section 2.3, the filler dispersion, interaction of the filler with the matrix and the nature of the interface between the two play a crucial role in enhancing the fracture properties of the composite.70,116 To improve the filler matrix interaction, functionalization of the filler is the most preferred route.189 Wan et al.60 have shown that the functionalization of graphene improved filler-matrix interaction, hence the fracture properties (Fig. 12B).
The graphene sheets are usually bound to each other due to van der Waals attraction. In the case of strong filler-matrix interaction, the filler is tightly bound to the matrix and does not tear apart from the matrix. In such a case, the graphene sheets can undergo delamination, i.e., the graphitic layers can separate when subjected to a mechanical load (Fig. 12C). This separation of graphitic layers allows the crack to eventually penetrate the layers to give rise to a dimpled fracture surface. All these factors contribute towards increased fracture surface area and hence towards improved fracture properties.103 Stress whitened zones are a way to assess the extent of toughening because they indicate the filler matrix interaction. The larger the stress whitened zone, the more the energy dissipated and the better the fracture properties.185,190
5. Comparison of epoxy composites containing different 2D nanofillers based on mechanical and thermal properties
A wide range of 2D materials, including graphene, inorganic 2D nano-sheets and their various hybrids, are being widely studied for reinforcing epoxy composites. It is evident from the literature survey that functionalized graphene has outperformed the other types of fillers in terms of enhancements observed in tensile, fracture and thermomechanical properties. Graphene oxide can be easily functionalized due to carboxyl and hydroxyl groups attached to its surface. The ease of synthesizing such fillers and their compatibility with various organic solvents have rendered functionalized GO a preferred option for reinforcing epoxy. However, TMDs have also shown promising improvements in the mechanical properties of epoxy composites. TMDs are the go-to option, especially when mechanically strong and electrically insulating polymer composites are required. Hybrid fillers have started to gain popularity in the last few years, especially multiscale hybrids. Hybrid fillers can prevent restacking and agglomeration of 2D nano-sheets by introducing and retaining gaps between the sheets.
Functionalized graphene performs better than blank graphene because it can be uniformly dispersed in the matrix due to the favorable interactions between the functionalizing species and the matrix. As a result, a stronger filler-matrix interaction is established while preventing agglomeration. Sometimes, the functionalized filler can contain primary and secondary amine groups, which act as secondary hardening agents and trigger epoxy curing reactions. As a result, the interface between such a filler and the matrix is further strengthened. When polymer grafted GO is used as a filler, the polymer chains attached to the sheet inhibit agglomeration and ensure uniform dispersion of the sheets in the matrix. However, an exception was observed where an unmodified filler also showed improvements in mechanical properties (Fig. 13). Yousefi et al.149 used reduced GO to produce a remarkable improvement in tensile properties. Since the reduction was carried out in situ using hydrazine, it was concluded that the oxygen functional groups left behind on the surface of rGO after reduction were responsible for bringing about effective load transfer.
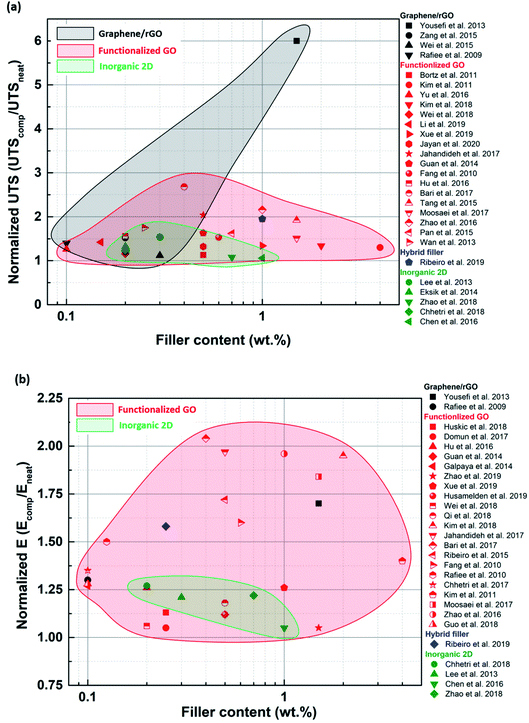 |
| Fig. 13 Normalized UTS (a) and E (b) plotted against filler loading for various types of fillers to compare the effects of each type of filler. | |
As compared to the other 2D materials, graphene has the highest inherent modulus, breaking strength and fracture toughness. Although functionalization of graphene improves dispersion, it can introduce defects and lower the inherent mechanical properties. Despite this, it is still evident that graphene performs better than inorganic 2D materials when it comes to enhancement in tensile properties. Functionalized graphene was capable of improving tensile properties in a broader loading range. Non-functionalized inorganic 2D nanosheets are more susceptible to agglomeration, and hence their loading needs to be restricted below the agglomeration threshold. Inorganic 2D nano-sheets are very often much smaller in lateral size than graphene sheets. As a result, the aspect ratios of graphene and its derivatives are higher than the inorganic 2D nano-sheets. Hence, better load transfer can be achieved in the case of graphene (Fig. 13).
Fracture property improvement ranging from 20% to >100% can be achieved using fGO as a filler, even at low loadings (Fig. 14). Improvements reported for blank graphene or rGO were much less than those reported for fGO, implying that better dispersion and load transfer occurs when the filler surface is modified. In exceptional cases such as Wu et al.,100 nearly 900% improvement in GIC was achieved for non-functionalized GNPs. The electrically conducting nature of graphene flakes was utilized to align them in a particular direction. As a result, the maximum toughening effect was observed when the direction of crack propagation was perpendicular to the graphene wall.
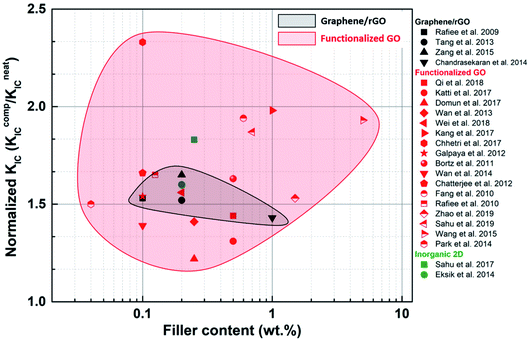 |
| Fig. 14 Comparison of the effect of various 2D fillers on the fracture toughness KIC of epoxy composites. | |
Irrespective of the combination of hybrid filler used, it has consistently been reported that the agglomeration and stacking of 2D nano-sheets can be successfully prevented by introducing another filler that acts synergistically with the 2D nano-sheet. The hybrid filler is capable of better filler matrix interaction due to reduced restacking and agglomeration issues. As a result, low loadings of hybrid fillers are also capable of significantly enhancing the fracture properties.
Mechanical properties like tensile and fracture strength are often studied at room temperature. To get some insight into the behavior of the epoxy composites at high temperatures, dynamic mechanical studies are a good way of qualitatively establishing the thermomechanical properties of epoxy composites and accurately determining their glass transition temperature. Especially in the aerospace industry, the material undergoes multiple thermal cycles, and hence thermomechanical studies can offer useful conclusions. Dynamic mechanical analysis (DMA) studies have not been extensively conducted for epoxy composites. The data available are scattered, and no consistency was observed (Fig. 15). The available data showed that functionalized fillers perform better, implying that stiffness is dependent on the filler matrix interaction. In the case of inorganic and hybrid fillers, more data are required to draw solid conclusions regarding the thermomechanical behavior. Thermal studies are often neglected or compromised during the synthesis of composites for structural applications. But, it is important to focus on improving thermomechanical properties simultaneously with the other mechanical properties.
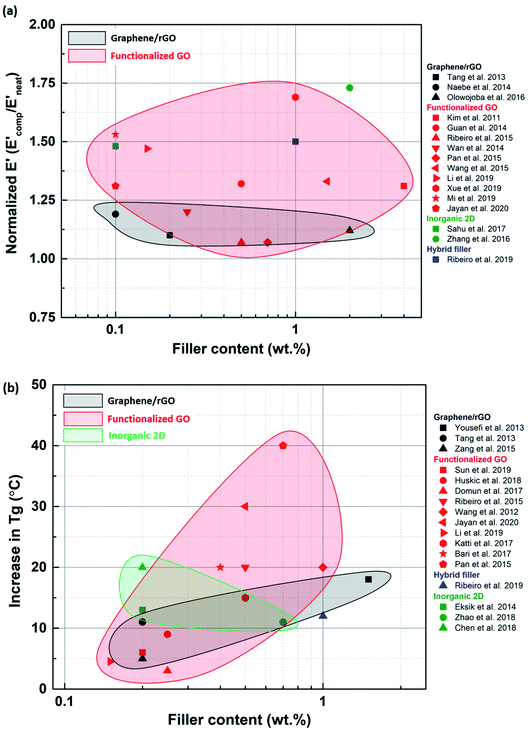 |
| Fig. 15 Effect of filler type and loading on E′ at 100 °C (a) and Tg (b) of epoxy composites. | |
T
g is often considered to be an indicator of the network density of the composite. The addition of a filler affects the cross-linking of the resin, in turn influencing the Tg. Especially in the case of amine-modified fillers, the filler performs multiple functions. Hence, when amine groups with a chemical structure similar to the hardener are attached to the filler, they tend to modulate the stoichiometric ratio around the filler to form a hierarchical structure. This structure can then influence the mobility of the epoxy chains around the filler, thereby affecting the Tg.
6. Challenges
6.1 Lack of unanimity related to the influence of filler size on composite properties
In nanoparticle reinforced composites, the filler's aspect ratio is a crucial parameter that affects the filler-matrix interaction and drives the matrix toughening mechanisms. The effect of filler size on the mechanical properties of epoxy composites was studied in depth by some research groups.191 Kim et al.189 studied the effect of non-oxidized graphite flakes (f-NOGFs) functionalized using potassium sodium tartrate tetrahydrate on epoxy resin. f-NOGFs were sorted into three different sizes (L = 0.25, 0.75 and 1 μm, t = 4 nm). It was reported that the epoxy composites containing f-NOGFs of size 1 μm showed the best tensile properties among the three. The E, UTS, and tensile toughness improved by 31%, 99%, and 230% than the neat epoxy at 0.6 wt% loading. This behavior was attributed to the large interfacial area of interaction between the matrix and the filler. Since the oxidation route was not followed for surface modification, the graphene flakes were relatively defect-free compared to GO, and their inherent strength was preserved. Similar results were reported by other authors.68,190 Huskić et al. reported that GO synthesized from expanded graphite of size 1200 μm displayed better tensile strength and Tg than GO from expanded graphite of size 130 μm. Alexopoulos et al.190 reported that among the two GNP types (grade C t = 2 nm L = 2 μm and grade M t = 6 nm and L = 15 μm), the larger GNP sheets showed an 18% higher UTS at 0.25 wt% as compared to 11% improvement for the composite containing smaller GNPs. Larger GO sheets connected better with the epoxy matrix and brought about efficient load transfer. In another study,192 the size effect of commercially available graphene nanoplatelets (GNPs) of two different sizes (L = 5 μm and 25 μm) on the fracture properties of epoxy was evaluated. An improvement of 82% and 60% in the fracture toughness was observed for large and small GNPs at a loading of 2 wt%, respectively. It was concluded that large-sized graphene sheets are capable of deflecting and bridging the advancing crack. However, the reverse was reported in ref. 185. It was observed that out of three different sizes (L = 10.79, 1.72, 0.7 μm, t = 15–30 nm), the smallest GO flakes gave the best fracture toughness, 75% higher than the neat resin, at 0.1 wt% loading. The larger sheets tend to have more wrinkles and folds and are likely to affect the filler's load-transfer ability and act as stress concentrators during fracture.193 Fractography analysis showed that the smallest-sized GO showed the maximum resistance to crack propagation compared to the other two sizes. It was therefore concluded that the fracture properties are inversely proportional to the size of the flakes. These results were in sync with the simulation results reported by Zhao et al.194 They reported an increase in stress concentration in the matrix with a decrease in the filler size at a constant loading. Shokrieh et al.195 compared the reinforcing effects of graphene nano-platelets (GPLs t = 3.5 nm and L = 40–120 nm) and graphene nanosheets (GNS t = 6–8 nm and L = 5–8 μm) to understand the effect of filler geometry on the fracture toughness of the composite. The fracture toughness improved by 39% and 16% for 0.5 wt% GPL/epoxy and GNS/epoxy composites, respectively compared to the neat resin. Tensile modulus improved by 10% for 0.5 wt% GPL/epoxy composite. GPLs performed better than GNS due to higher surface area of interaction with the matrix and lower thickness.
Graphene nano-sheets falling in a wide size range (40 nm to 25 μm) have been used by various research groups. The terms ‘large’ and ‘small’ have been used for a very wide size range on a relative scale. There are no well-defined size brackets to classify graphene sheets as ‘small,’ ‘medium’ or ‘large’ sheets. As a result, it is difficult to draw clear conclusions related to the effect of the size of 2D fillers on the mechanical properties of epoxy composites. There is a need for standardization of nanomaterials based on the size and thickness and other crucial properties such as purity, surface roughness etc.,196 which is discussed in detail in Section 6.5 below.
Moreover, many factors such as the resin's type and properties, the functionalization level of the filler, loading conditions, composite fabrication method, curing schedule, etc. affect the final properties of the epoxy composite. Hence, no solid conclusion can be drawn from the literature mentioned above about the size effect of the filler on epoxy composites because it is subject to a number of other factors.
6.2 Filler dispersion: methods, characterization and challenges
Solution mixing is a very widely used dispersion technique due to the filler's affinity to organic solvents.143,197 However, solution mixing involves using organic solvents, which can cause degradation of the composite properties if not completely removed before curing. Atif et al.187 studied the effect of retained acetone in multilayer graphene (MLG) reinforced epoxy composites. Epoxy composites were synthesized using three dispersing media: MLG in the hardener (MH), MLG in epoxy (ME) and MLG in acetone (MA). The best mechanical properties were observed for the MH-type composites. At 0.3 wt% of MH, the impact toughness, young's modulus and flexural modulus increased by 89%, 24% and 46%, respectively, compared to neat epoxy. The KIC and GIC improved by 29% and 7%, respectively, at 0.1 wt% MH loading. It was reported that trace solvent (acetone) could weaken the epoxy chains, give rise to porosity and act as stress concentration sites, degrading the Tg and mechanical properties. On similar lines Chong et al.191 studied the effects of residual solvents on the properties of epoxy composites. The authors reported that the residual solvent THF and NMP in epoxy composites lower the Tg significantly (>50 degrees), the effects being more profound for NMP. The residual solvent also lowered the tensile properties. However, the fracture toughness and fracture energy both increased for composites containing residual solvents. This might result from lower cross-linking because the solvent inhibited the curing reaction of epoxy to some extent. The lower cross-linking results in lower brittleness and thereby improved fracture properties.
It is noteworthy that the industries do not approve of the solvent-based approach because solvent removal adds an extra step, consuming extra time and increasing the cost. Also, improper disposal of solvents can cause damage to human health and the environment. Hence solvent-free methods for composite fabrication like direct addition of the filler to the hardener or resin using high-speed shear mixing or ultrasonication,71,147 three roll milling,198,199 phase transfer methods,110 resin impregnation176,178etc. are being studied. Three roll milling performs a dual function when a resin–filler mixture is subjected to shear forces acting between three cylindrical rolls. It exfoliates multiple-layer sheets into few layers in situ and ensures uniform dispersion in the epoxy matrix without additives or solvents.
Nonetheless, if the shear forces in the solvent-free dispersion methods exceed the optimum limit, it can introduce defects in the graphene sheets and weaken the composite. Industries encourage solvent-free methods for dispersion because it reduces industrial waste and the risk of hazards. However, solvent-free methods are difficult to scale up while maintaining the quality of dispersion. Hence, new techniques which can achieve homogeneous dispersion without the risk of hazards need to be explored.
A wide range of characterization methods have been used to confirm the state of dispersion of the graphene filler in the epoxy matrix. Optical,70 electron68,104 and atomic force microscopy71 techniques are commonly used to visually comprehend the dispersion state of the filler. However, at low loadings (≤0.1 wt%), it can be difficult to study dispersion using microscopy techniques since a layer of epoxy resin might shield the filler.106 As a result, other methods were considered. It was reported by Hu et al.145 that a high-intensity D band in the Raman spectra was an indication of graphene agglomeration. Similar results were reported in ref. 200 and 201. Eksik et al.151 reported a novel confocal Raman imaging method for evaluating the dispersion state of MoS2 nanoplatelets in epoxy resin. Since uniform dispersion of pristine TMDs is challenging at high loading levels, significant agglomeration was observed beyond 0.3 wt% loading.
XRD is an effective tool to analyze the degree of exfoliation of graphene in the epoxy matrix at low loadings.70,121,140,202,203 Qi et al. used the XRD tool to study GO exfoliation. The XRD curve of unmodified epoxy displayed a broad diffraction peak in the range of 2θ = 8–30, corresponding to cured epoxy. The diffraction curve of GO/epoxy and HBPA–GO/epoxy composites also contained the same broad peak of epoxy, but the characteristic peak of GO and HBPA–GO were absent, thereby indicating efficient exfoliation. It is noteworthy that good exfoliation does not indicate good dispersion, and hence other techniques must be used to confirm the dispersion state.125 Chen et al. performed UV-vis spectroscopy on 2 mm thick samples of MoS2/epoxy composites. It was reported that amine-f-MoS2/epoxy composites displayed a 10% transmittance against 56% and 65% transmittance of 0.5 wt% MoS2/epoxy composites and neat epoxy, respectively, thereby indicating that the dispersion state of amine-f-MoS2 in epoxy was more uniform than pristine MoS2.83
SEM and TEM remain the most popular methods for visually evaluating the filler's dispersion state in the matrix. However, the area under consideration during microscopy analysis is of the scale of microns and is extremely small as compared to the end product. Even if the dispersion appears uniform under the microscope, one cannot be certain that it is uniform throughout the sample. Therefore, it is still challenging to evaluate filler dispersion inside the matrix with total certainty and reproducibility.
6.3 Industry scale-up and commercialization
After the discovery of graphene in 2004, researchers have been making continuous efforts to utilize the full potential of this revolutionary nanomaterial. Graphene has turned out to be the torchbearer for other nanomaterials such as TMDs (e.g., WS2, MoS2, MoSe2, and WSe2), which have also garnered attention. However, after years of effort the most important question remains unanswered. When will the world witness the game-changing 2D nanomaterials being used in real applications?
The use of 2D nanomaterials in advanced composites for industrial applications is still at a very early stage. However, as the understanding of material properties, processing requirements, and raw material availability has improved over the years, some industries have started to adopt these new materials. Despite few industrial implementations that the 2D nanomaterials have found, especially in the sports industry (Table 6), these new promising nanomaterials have not delivered to their hype yet. Moreover, there is no clarity on what type of graphene derivative is used in commercial sports goods. Whether it is monolayered or multilayered, non-functionalized or functionalized or small or large graphene sheets is ambiguous. Also, there have been very few developments where graphene has been used in commercial aerospace or automotive products. Notably, in 2019 Aernnova (Álava, Spain), Grupo Antolin-Ingenieria (Burgos, Spain) and Airbus (Toulouse, France), working as partners for a Graphene Flagship (Gothenburg, Sweden) consortium funded by the European Union, manufactured Airbus A350 using graphene-enhanced resin in the form of CFRP. It was reported that this effort led to the development of lightweight composites resulting in fuel saving. UK-based prepreg manufacturer, Haydale launched a graphene-modified prepreg material for lightning strike protection of aircraft. In the case of the automotive industry, there is one industrial implementation by Ford worth noticing. Ford revealed in 2019 the use of graphene-enhanced foam in their Ford F-150 and Mustang vehicles. It was reported that the use of graphene led to a 20% increase in mechanical properties along with 17% and 30% improvement in noise reduction and heat endurance, respectively. However, the 20% improvement in mechanical properties is far from the 50% to more than 100% improvement in mechanical properties reported in most graphene-based polymer composite studies reported in the literature. The important question that needs to be answered is what is stopping the manufacturers from utilizing these nanomaterials to their full potential and what is the bottleneck for the aerospace and automotive industry-wide adoption of these materials?
Table 6 Commercial graphene composite products
Product |
Model name |
Sector |
Company |
Application |
Year |
Attributes |
Nano-material used |
Tennis racket |
Youtek Graphene Speed S Series |
Sports |
Head |
Structural |
2013 |
Improved strength to weight ratio |
Graphene |
Skis |
Joy |
Sports |
Head |
Structural |
2014 |
Improved strength to weight ratio |
Graphene |
Tennis racket |
Graphene XT |
Sports |
Head |
Structural |
2015 |
Improved strength to weight ratio |
Graphene |
Bicycle wheel |
Quarno |
Sports |
Vittoria |
Thermal |
2015 |
Improved heat dissipation |
Graphene |
Bicycle |
Interceptor graphene |
Sports |
Dassi Bikes |
Structural |
2016 |
Improved strength to weight ratio |
Graphene |
Sports shoes |
G-series shoes |
Sports |
Inov-8 & Manchester University |
Structural |
2018 |
Improved strength and flexibility |
Graphene |
Epoxy paste adhesive |
AGM TP300 AGM TP400 |
Manufacturing |
Applied Graphene Materials |
Thermal |
2019 |
Thermally conductive polymer material |
Graphene |
3D printing filament |
Koltron G1 |
Manufacturing |
Graphmatech & Add North |
Thermal |
2019 |
Thermally conductive polymer material |
Graphene |
Nylon Aros graphene pellets |
Aros Create |
Manufacturing |
Graphmatech |
Structural |
2019 |
Electrical and tribological properties |
Graphene |
Bullet proof vest |
2AM line |
Defence |
Planar Tech |
Structural |
2020 |
Improved strength to weight ratio |
Graphene + UHMWPE |
Bicycle wheel |
Eagle F1 |
Sports |
Goodyear |
Structural |
2020 |
Improved strength to weight ratio |
Graphene + amorphous spherical silica |
6.4 2D materials – the hype cycle
Gartner Inc. introduced the hype cycle model, which explains the journey of a technological innovation going through the peak of hype and reaching realistic scenarios where the technology finds a way for final implementation with respect to time. The hype curve is applicable to 2D nanomaterials including graphene. Fig. 16 shows the Gartner hype cycle for graphene and graphene-based materials.
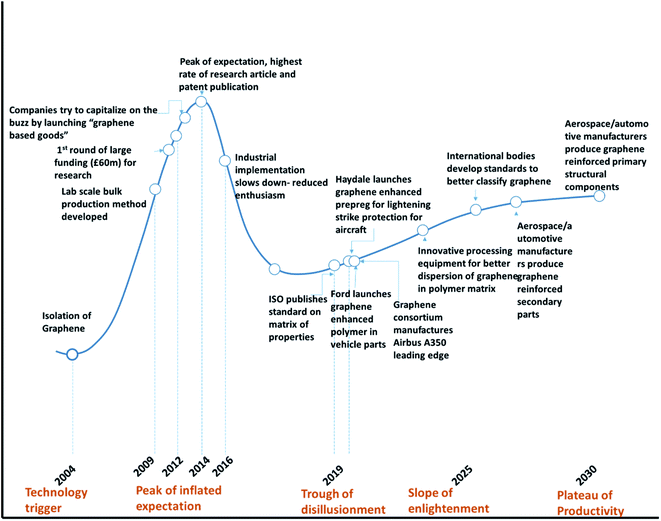 |
| Fig. 16 Gartner hype cycle for graphene based polymer composite product commercialization.205,206 | |
Since the isolation of graphene by Novoselov of Manchester University in 2004,204 researchers were curious, and various groups were trying to find the potential for numerous applications. Fig. 17 shows the initial trend in several publications between 2004 and 2009. There was a steady increase in the number of studies and publications. However, when Novoselov received the Nobel Prize in 2010, there was a sudden jump in the number of publications. The number of publications increased fourfold between the years 2009 and 2014. This was the peak of the hype cycle for graphene. This was also the time where many sports goods manufacturers developed “graphene-enhanced” products. Few commercial goods manufacturers claimed to utilize graphene to improve strength. The year 2013 onwards, many sports goods manufacturers launched products such as tennis rackets, golf clubs, bicycle structures, etc., that claimed to have used graphene for improved strength and improved weight to strength ratio. However, the exact details of the graphene nanomaterials used in these products were not clear.
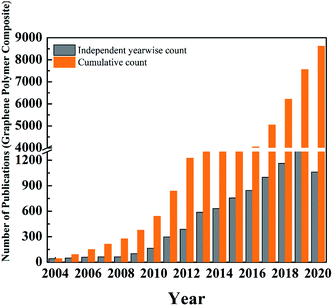 |
| Fig. 17 Statistical graphs showing cumulative and year wise independent number of publications related to graphene (data source: Pubmed). | |
Table 6 shows the list of commercial products, which have graphene components incorporated for structural applications. This is indicated by the phase between year 2012 and 2013 in the “hype cycle” where few companies tried to utilize the buzz around the new technology. Between 2016 and 2018, there was a steady increase in the number of patents and publications related to graphene-based materials (Fig. 17) and continued funding for academic and industrial research. However, there were no major announcements by the big players in aerospace and automotive companies to utilize graphene to improve the performance of the structural component of the products. This was typical of the trough region of the Gartner hype curve shown around the year 2016 (Fig. 16). The current scenario places graphene-based polymer composite research in the “slope of enlightenment region” shown in the timeline between 2019 and 2021 in Fig. 16, where industries find practical application with realistic goals to enhance the properties by using graphene and other 2D material reinforced composites. Though the number of such instances is still extremely small, a more systematic approach will increase confidence building for structural application in various industries.
6.5 Lack of standardization of graphene and other 2D materials
One of the most important reasons behind the lack of confidence in implementing graphene in industrial applications is the uncertainty regarding the reproducibility of the results. The final graphene reinforced composite properties vary significantly with average sheet size, distribution level and extent of functionalization of graphene, etc. From the literature on graphene-reinforced polymer composites, it is clear that reproducing the batch with mechanical and thermal properties is extremely difficult because of dependency on the aforementioned parameters. This is one reason behind the disparity in the extent of improvements in mechanical properties reported by different research groups. The aerospace industry works on very stringent regulations and guidelines imposed by the respective aerospace regulatory bodies of every country, as the safety of passengers is at stake and depends on the reliable performance of aircraft parts during service. Hence, the aerospace industry requires a robust material system, which should be reproducible. In a recent article, Terrance Barkan, executive director of Graphene council explained in depth the challenges about the issue of absence of “standard grade” of graphene and any reference materials.206 He also emphasized the lack of transparency regarding the grade of graphene being used by manufacturers. Many companies are manufacturing and supplying graphene and other 2D nanomaterials on a commercial scale (Table 7). Some of the companies also provide the relevant material characterization details such as XRD and SEM data. However, the user still has to perform additional characterization to understand the complete properties of the 2D nanomaterials. It may be relatively easier for researchers to validate the data provided by these companies, as most research institutes have the required characterization equipment such as XPS, XRD, FTIR, SEM and TEM etc. However, it may not be possible for industries to do such a verification because of the inaccessibility of the required resources. Also, some 2D nanomaterial manufacturers do not provide any insight about the type of graphene platelets in terms of the average number of layers, sheet size, functionalization and on the other hand, some other manufacturers provide “their own” classification and different companies give different names for the different 2D nanomaterial variants. Hence it is extremely important for international testing standards development bodies to pay attention to this need and develop dedicated standards for these unique materials similar to other conventional materials.
Table 7 Worldwide commercial manufacturers of graphene/CNTs/2D nanomaterials
Sr. no. |
Manufacturer |
Nanomaterial product |
Country of origin |
1 |
Nanocyl |
CNTs |
Belgium |
2 |
6 Carbon Technology |
CVD grown TMDs 2D films, graphene, hBN |
China |
3 |
Shenzhen Nanotech |
CNTs |
China |
4 |
2D Carbon Tech |
Graphene |
China |
5 |
Arkema |
CNTs |
France |
6 |
Ad-nanotech 2013 |
Graphene, MWCNTs |
India |
7 |
Hexorp |
Graphene, graphene oxide, reduced graphene oxide |
India |
8 |
Abalonyx |
Graphene oxide, graphene oxide derivatives |
Norway |
9 |
Advanced Graphene Products |
Graphene, graphene oxide, reduced graphene oxide |
Poland |
10 |
Akkolab |
Graphene oxide and reduced-GO materials |
Russia |
11 |
2DM |
Graphene flakes |
Singapore |
12 |
Graphenea |
Graphene oxide |
Spain |
13 |
2D Fab AB |
Graphene flakes |
Sweden |
14 |
Applied Graphene Materials |
Graphene |
United Kingdom |
15 |
Cambridge Nanosystems |
Graphene |
United Kingdom |
16 |
Directa plus |
Graphene |
United Kingdom |
17 |
Haydale |
Graphene |
United Kingdom |
18 |
Nanointegris |
Graphene, CNTs, boron nanotubes |
USA |
19 |
Nano C |
CNTs |
USA |
20 |
G6 Materials Corp. |
Graphene |
USA |
21 |
2D Semiconductors |
CVD grown TMDs |
USA |
22 |
XG Sciences |
Graphene |
USA |
In 2019, ISO released a standard207 specifically for graphene and 2D materials titled “ISO/TR 19733:2019 Nanotechnologies—Matrix of properties and measurement techniques for graphene and related two-dimensional (2D) materials” which related properties of the graphene & other 2D materials to measurement techniques using a matrix. In future, more such initiatives to standardize and classify the 2D nanomaterials will pave the way for true commercialization of graphene for all the applications envisioned by the researchers. And industries like aerospace will be more likely to adopt these “wonder materials” for potential weight saving (Fig. 18).
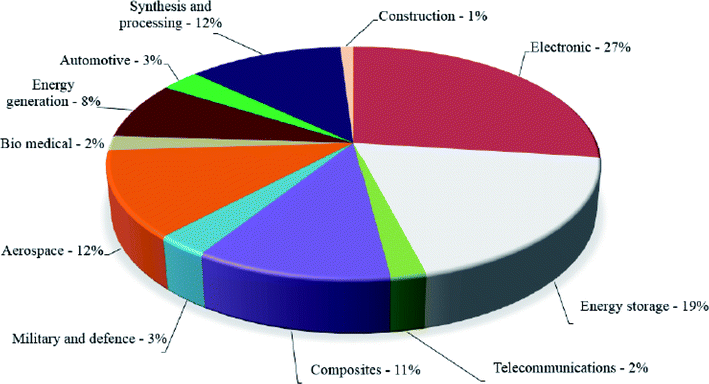 |
| Fig. 18 Commercial applications of graphene-based materials.208 Reprinted with permission from ref. 208 (Crown Copyright © 2017 Published by Elsevier Ltd). | |
6.6 Health hazards
As we move towards further commercialization of graphene and other 2D material-based products, a very important question needs to be answered. Are 2D materials safe for humans and the environment? Since the discovery of CNTs and graphene, few studies have focused on the interaction of these materials with biological systems and their cytotoxicity. The researchers have brought attention to the risk of occupational exposure to 2D materials. The most probable scenario of occupational exposure of humans to 2D materials is during the industrial production or disposal of lab/industrial waste via inhalation, ocular, cutaneous or oral routes (Fig. 19A). The inhalation route is the most studied route among the others.
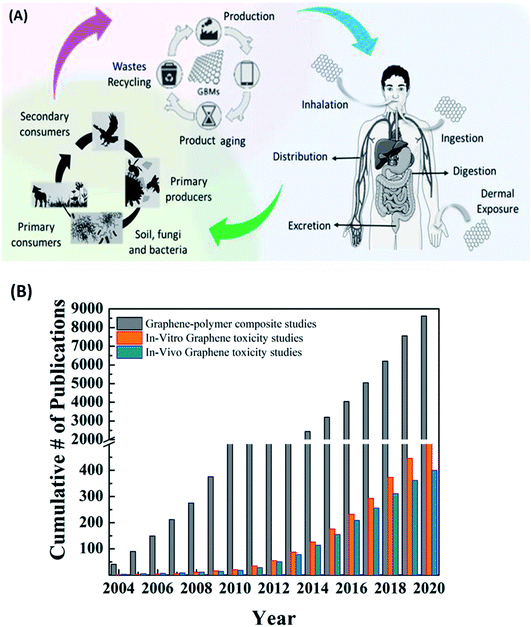 |
| Fig. 19 (A) Schematic showing health and environmental hazards of graphene exposure.223 Reprinted with permission from ref. 223 (Copyright © 2018, American Chemical Society). (B) Comparative chart of the number of publications for graphene/graphene derivative-polymer composites with the number of publications on in vivo and in vitro toxicity studies. | |
The ability of graphene and other 2D materials to exist in a form as thin as an atom and yet remain mechanically strong and robust is as problematic as it is exciting. This very nature of these 2D “nano” materials imparts the property of being airborne and poses a big risk of getting inhaled while working with these materials. Once settled in the lungs, these materials may lead to cytotoxicity and initiate cell damage. One of the initial studies about the cytotoxicity of graphene and graphene oxide was conducted by Zhang et al.209 The authors performed the studies in vitro and provided exposure to cell lines with graphene and CNT concentration ranging from 0.01 μg ml−1 to 100 μg ml−1 for 24 h. The study showed accumulation of graphene platelets on cell membranes after 24 h of exposure, and exposure to CNTs shows a relatively greater cellular membrane damage. In vitro studies have demonstrated that functionalization of graphene with biocompatible functional groups and polymers decreased the cytotoxicity of these materials.210 The review about the cytotoxicity of graphene and graphene oxide by Seabra et al.211 revealed that many factors such as concentration, functionalization, number of layers, time of exposure and synthesis route affect the toxicity of graphene. There is consensus about the mechanism of toxicity in biological systems by carbon and other nanomaterials. One of the mechanisms is via oxidative stress in cells. Oxidative stress occurs in cells when there are excess of free radicals, which may damage cells, proteins, DNA, etc. Oxidative stress occurs via the formation of reactive oxygen species (ROS). Another mechanism is the possible cell membrane damage by the sharp edges of the sheets of 2D materials.212 Many in vitro studies have shown toxic effects of graphene and GO in lung cells, skin cells and stem cells.213–218 Several in vitro studies show no toxic effect of graphene and GO on cells.219–221 Hence there are conflicting conclusions from in vivo studies about the cytotoxicity/biocompatibility of nanomaterials, especially CNTs and graphene materials. Xiaoli et al.222 have published a comprehensive review that provides detailed insights from the literature to date from in vitro and in vivo studies. The authors have conveyed that despite many research groups working to study the toxicity of the nanomaterials (Fig. 19B), it is difficult to arrive at any conclusion because of the lack of dependable experimental models and evaluation standards.
Additionally, due to the lack of standardization of graphene grades and other 2D materials, it is difficult to understand to which 2D nanomaterial is the toxicity study applicable, since graphene produced by one research group could differ from the one produced by another research group. The variations could arise from a difference in the number of graphene layers, length and width of graphene sheets, and the difference in the C to O ratio. Hence, it's very difficult to compare the results obtained by different research groups, leading to conflicting observations. Therefore, there is an urgent need for more research to evaluate the toxicity of graphene and other nanomaterials for human exposure.
7. Conclusion
Several 2D nano-sheets, including graphene and its derivatives, TMDs, hBN, MXenes, etc., have been studied as reinforcements for epoxy composites to enhance their mechanical performance. Among these nano-sheets, graphene derivatives have been on the lead due to many reasons: (i) ease of synthesis through the chemical route, (ii) ease of functionalization, (iii) inherently higher modulus and strength of graphene as compared to other 2D materials, (iv) compatibility with organic solvents and (v) higher aspect ratio. The literature available on epoxy reinforced with inorganic 2D nano-sheets is scarce in comparison to graphene reinforced epoxy. However, some authors have proven that TMDs and hBN can produce remarkable improvements in the mechanical properties of epoxy composites. TMDs are especially useful because of their high bandgap, when an electrically insulating and mechanically robust polymer composite is required. Recently, the use of hybrid fillers has become popular because the merits of two different fillers can be synergistically combined to achieve toughened epoxy composites. Not just that, the nature of multiscale hybrid fillers is such that they form favourable architecture that is capable of establishing a strong filler matrix interaction and hence facilitate better load transfer.
Through this review, we have tried to bring the research community's attention to the challenges and future strategic approach in 2D nano-material reinforced epoxy composites. More focus and work are required in terms of the health and environmental effects of these nanomaterials. Also, the regulatory bodies should come up with standard protocols for disposal of these nanomaterials to prevent any environmental damage.
A clear understanding of the classification based on chemical composition, physical properties, constituents, etc., is available for commercial metal alloys and plastic. There should be efforts to standardize 2D nano-materials like all other standard commercial materials. Since the nature of 2D nano-sheets is different than bulk materials, the classification categories could be based on sheet size and thickness, defects, extent and type of functionalization, purity, etc. The standardization will help accelerate the journey of 2D reinforced epoxy composites towards commercialization and industrialization.
As the endeavors to address the challenges mentioned above are pursued, and progress is made, it should also be noticed that industrialization depends on various technologies available for the processing of these nano-materials. As we know, currently, there is no focused effort to develop new technology dedicated to processing these nanomaterials to utilize the full potential of these few atom-thick nano-sheets. Hence, there is a great need to develop solutions and technologies to enable better dispersion of 2D sheets in the matrix for achieving large-scale production while maintaining enhanced mechanical properties.
If the above challenges are addressed in the coming decade, then a revolution can be expected in terms of a larger spectrum of industries successfully utilizing these 2D wonder materials to enhance the performance of their products. We may witness another breakthrough like the one experienced in carbon fiber reinforced epoxy composites implemented for aerospace structural components after 30 years of rigorous research and development by academic and industrial organizations. In the coming decades, if the gap between lab-scale research and industry is bridged, 2D nanosheets can lead the way for extremely light and tough composites to reduce the carbon footprint by enabling aerospace industries to manufacture lightweight aircraft. The automotive and sports industry can also benefit from graphene and other 2D materials if these challenges are systematically addressed.
Conflicts of interest
There are no conflicts to declare.
Acknowledgements
The authors are grateful to the Ministry of Human Resources and Development, Government of India for granting Radhika Wazalwar the senior research fellowship.
References
- L. C. Tang, Y. J. Wan and K. Peng,
et al., Fracture toughness and electrical conductivity of epoxy composites filled with carbon nanotubes and spherical particles, Composites, Part A, 2013, 45, 95–101 CrossRef CAS.
- L. C. Tang, X. Wang and Y. J. Wan, Mechanical properties and fracture behaviors of epoxy composites with multi-scale rubber particles, Mater. Chem. Phys., 2013, 141, 333–342 CrossRef CAS.
- L. Chen, S. Chai, K. Liu, N. Ning, J. Gao, Q. Liu, F. Chen and Q. Fu, Enhanced Epoxy/Silica Composites Mechanical Properties by Introducing Graphene Oxide to the Interface, ACS Appl. Mater. Interfaces, 2012 Aug, 4(8), 4398–4404, DOI:10.1021/am3010576.
- M. W. Ho, C. K. Lam, K.-t. Lau, D. H. L. Ng and D. Hui, Mechanical properties of epoxy-based composites using nanoclays, Compos. Struct., 2016, 75, 415–421, DOI:10.1016/j.compstruct.2006.04.051.
- E. T. Thostenson and T. W. Chou, Processing-structure-multi-functional property relationship in carbon nanotube/epoxy composites, Carbon, 2006, 44, 3022–3029 CrossRef CAS.
- L. c. Tang, H. Zhang and J. h. Han,
et al., Fracture mechanisms of epoxy filled with ozone functionalized multi-wall carbon nanotubes, Compos. Sci. Technol., 2011, 72, 7–13 CrossRef CAS.
- Z. Liu, J. Li and X. Liu, Novel Functionalized BN Nanosheets/Epoxy Composites with Advanced Thermal Conductivity and Mechanical Properties, ACS Appl. Mater. Interfaces, 2020, 12(5), 6503–6515, DOI:10.1021/acsami.9b21467.
- M. Sahu, L. Narashimhan, O. Prakash and A. M. Raichur, Noncovalently Functionalized Tungsten Disulfide Nanosheets for Enhanced Mechanical and Thermal Properties of Epoxy Nanocomposites, ACS Appl. Mater. Interfaces, 2017, 9(16), 14347–14357, DOI:10.1021/acsami.7b01608.
- C. Lee, X. Wei, J. W. Kysar and J. Hone, Measurement of the elastic properties and intrinsic strength of monolayer graphene, Science, 2008, 321(5887), 385–388, DOI:10.1126/science.1157996.
- N. Domun, H. Hadavinia, T. Zhang, T. Sainsbury, G. H. Liaghat and S. Vahid, Improving the fracture toughness and the strength of epoxy using nanomaterials – a review of the current status, Nanoscale, 2015, 7, 10294, 10.1039/c5nr01354b.
- B. T. Marouf, Y.-W. Mai, R. Bagheri and R. A. Pearson, Toughening of Epoxy Nanocomposites: Nano and Hybrid Effects, Polym. Rev., 2016, 56(1), 70–112, DOI:10.1080/15583724.2015.1086368.
- U. Szeluga, S. Pusz, B. Kumanek, K. Olszowska, A. Kobyliukh and B. Trzebicka, Effect of graphene filler structure on electrical, thermal, mechanical, and fire retardant properties of epoxy-graphene nanocomposites – a review, Crit. Rev. Solid State Mater. Sci., 2021, 46, 152–187, DOI:10.1080/10408436.2019.1708702.
- R. Atif, I. Shyha and F. Inam, Mechanical, Thermal, and Electrical Properties of Graphene-Epoxy Nanocomposites—A Review, Polymers, 2016, 8, 281 CrossRef PubMed.
- H. B. Kulkarni, P. Tambe and G. M. Joshi, Influence of covalent and non-covalent modification of graphene on the mechanical, thermal and electrical properties of epoxy/graphene nanocomposites: a review, Compos. Interfaces, 2018, 25, 381–414, DOI:10.1080/09276440.2017.1361711.
- N. P. Singh, V. K. Gupta and A. P. Singh, Graphene and carbon nanotube reinforced epoxy nanocomposites: a review, Polymer, 2019, 180, 121724, DOI:10.1016/j.polymer.2019.121724.
- A. K. Geim and K. S. Novoselov, The rise of graphene, Nat. Mater., 2007, 6, 183–191 CrossRef CAS PubMed.
- K. I. Bolotin, K. J. Sikes, Z. Jiang, M. Klima, G. Fudenberg, J. Hone, P. Kim and H. L. Stormer, Ultrahigh electron mobility in suspended graphene, Solid State Commun., 2008, 146, 351–355, DOI:10.1016/j.ssc.2008.02.024.
- S. V. Morozov, K. S. Novoselov, M. I. Katsnelson, F. Schedin, D. C. Elias, J. A. Jaszczak and A. K. Geim, Giant Intrinsic Carrier Mobilities in Graphene and Its Bilayer, Phys. Rev. Lett., 2008, 100, 016602, DOI:10.1103/PhysRevLett.100.016602.
- A. A. Balandin, S. Ghosh, W. Bao, I. Calizo, D. Teweldebrhan, F. Miao and C. N. Lau, Superior Thermal Conductivity of Single-Layer Graphene, Nano Lett., 2008, 8(3), 902–907 CrossRef CAS PubMed.
- P. Avouris and C. Dimitrakopoulos, Graphene: synthesis and applications, Mater. Today, 2012, 15(3), 86–97, DOI:10.1016/S1369-7021(12)70044-5.
- D. W. Boukhvalov and M. I. Katsnelson, Chemical functionalization of graphene, J. Phys.: Condens. Matter, 2009, 21(34), 344205 CrossRef CAS PubMed.
- S. Ahadian, M. Estili and V. J. Surya,
et al., Facile and green production of aqueous graphene dispersions for biomedical applications, Nanoscale, 2015, 7, 6436–6443, 10.1039/c4nr07569b.
- Q. He, S. Wu, Z. Yin and H. Zhang, Graphene-based electronic sensors, Chem. Sci., 2012, 3, 1764–1772, 10.1039/c2sc20205k.
- Y. Shao, J. Wang, H. Wu, J. Liu, I. A. Aksay and Y. Lin, Graphene Based Electrochemical Sensors and Biosensors: A Review, Electroanalysis, 2010, 22(10), 1027–1036, DOI:10.1002/elan.200900571.
- O. S. Iglesias, S. Collado, P. Oulego and M. Díaz, Graphene-family nanomaterials in wastewater treatment plants, Chem. Eng. J., 2017, 313, 121–135, DOI:10.1016/j.cej.2016.12.022.
- K. P. Loh, Q. Bao, G. Eda and M. Chhowalla, Graphene oxide as a chemically tunable platform for optical applications, Nat. Chem., 2010, 2, 1015–1024, DOI:10.1038/nchem.907.
- F. Zhai, Y. Feng, K. Zhou and L. Wang,
et al., Graphene-based chiral liquid crystal materials for optical applications, J. Mater. Chem. C, 2019, 7, 2146–2171, 10.1039/c8tc04947e.
- A. Li, C. Zhang and Y. F. Zhang, Thermal Conductivity of Graphene-Polymer Composites: Mechanisms, Properties, and Applications, Polymers, 2017, 9, 437, DOI:10.3390/polym9090437.
- M. Chhowalla, H. S. Shin, G. Eda, L. J. Li, K. P. Loh and H. Zhang, The chemistry of two-dimensional layered transition metal dichalcogenide nanosheets, Nat. Chem., 2013, 5, 263–275, DOI:10.1038/nchem.1589.
- Q. Weng, X. Wang, X. Wang, Y. Bandoa and D. Golberg, Functionalized hexagonal boron nitride nanomaterials: emerging properties and applications, Chem. Soc. Rev., 2016, 45, 3989–4012, 10.1039/c5cs00869g.
- W. J. Ong, L. L. Tan, Y. H. Ng, S. T. Yong and S. P. Chai, Graphitic Carbon Nitride (g-C3N4)-Based Photocatalysts for Artificial Photosynthesis and Environmental Remediation: Are We a Step Closer To Achieving Sustainability?, Chem. Rev., 2016, 116(12), 7159–7329, DOI:10.1021/acs.chemrev.6b00075.
- P. Chen, N. Li, X. Chen, W. J. Ong and X. Zhao, The rising star of 2D black phosphorus beyond graphene: synthesis, properties and electronic applications, 2D Materials, 2018, 5(1), 014002 CrossRef.
- M. Naguib, V. N. Mochalin, M. W. Barsoum and Y. Gogotsi, 25th Anniversary Article: MXenes: A New Family of Two-Dimensional Materials, Adv. Mater., 2014, 26(7), 992–1005, DOI:10.1002/adma.201304138.
- Q. Wang and D. O'Hare, Recent Advances in the Synthesis and Application of Layered Double Hydroxide (LDH) Nanosheets, Chem. Rev., 2012, 112(7), 4124–4155, DOI:10.1021/cr200434v.
- Y. Du, Z. Yin and J. Zhu, A general method for the large-scale synthesis of uniform ultrathin metal sulphide nanocrystals, Nat. Commun., 2012, 3, 1177, DOI:10.1038/ncomms2181.
- Y. Chen, Z. Fan, Z. Zhang, W. Niu, C. Li, N. Yang, B. Chen and H. Zhang, Two-Dimensional Metal Nanomaterials: Synthesis, Properties, and Applications, Chem. Rev., 2018, 118(13), 6409–6455, DOI:10.1021/acs.chemrev.7b00727.
- J. W. Colson and W. R. Dichtel, Rationally synthesized two-dimensional polymers, Nat. Chem., 2013, 5, 453–465, DOI:10.1038/nchem.1628.
- Y. Peng, Y. Li, Y. Ban, H. Jin, W. Jiao, X. Liu and W. Yang, Metal-organic framework nanosheets as building blocks for molecular sieving membranes, Science, 2014, 346(6215), 1356–1359, DOI:10.1126/science.1254227.
- J. W. Colson, A. R. Woll, A. Mukherjee, M. P. Levendorf, E. L. Spitler, V. B. Shields, M. G. Spencer, J. Park and W. R. Dichtel, Oriented 2D Covalent Organic Framework Thin Films on Single-Layer Graphene, Science, 2011, 332(6026), 228–231, DOI:10.1126/science.1202747.
- J. Shamsi, Z. Dang, P. Bianchini, C. Canale, F. D. Stasio, R. Brescia, M. Prato and L. Manna, Colloidal Synthesis of Quantum Confined Single Crystal CsPbBr3 Nanosheets with Lateral Size Control up to the Micrometer Range, J. Am. Chem. Soc., 2016, 138(23), 7240–7243, DOI:10.1021/jacs.6b03166.
- C. Zhi, Y. Bando, C. Tang, H. Kuwahara and D. Golberg, Large-Scale Fabrication of Boron Nitride Nanosheets and Their Utilization in Polymeric Composites with Improved Thermal and Mechanical Properties, Adv. Mater., 2009, 21(28), 2889–2893, DOI:10.1002/adma.200900323.
- P. Zhang, L. Ma, F. Fan, Z. Zeng, C. Peng, P. E. Loya, Z. Liu, Y. Gong, J. Zhang, X. Zhang, P. M. Ajayan, T. Zhu and J. Lou, Fracture toughness of graphene, Nat. Commun., 2014, 5, 3782, DOI:10.1038/ncomms4782.
- J. W. Suk, R. D. Piner, J. An and R. S. Ruoff, Mechanical Properties of Monolayer graphene oxide, ACS Nano, 2010, 4(11), 6557–6564 CrossRef CAS PubMed.
- Z. Meng, R. A. S. Crespo, W. Xia, W. Gao, L. Ruiz, H. D. Espinosa and S. Keten, A coarse-grained model for the mechanical behavior of graphene oxide, Carbon, 2017, 11, 476–487 CrossRef.
- C. G. Navarro, M. Burghard and K. Kern, Elastic Properties of Chemically Derived Single Graphene Sheets, Nano Lett., 2008, 8(7), 2045–2049, DOI:10.1021/nl801384y.
- M. M. J. Treacy, T. W. Ebbesen and J. M. Gibson, Exceptionally high Young's modulus observed for individual carbon nanotubes, Nature, 1996, 381, 678 CrossRef CAS.
- L. Yang, I. Greenfeld and H. D. Wagner, Toughness of carbon nanotubes conforms to classic fracture mechanics, Sci. Adv., 2016, 2(2), 1500969, DOI:10.1126/sciadv.1500969.
- M. F. Yu, O. Lourie, M. J. Dyer, K. Moloni, T. F. Kelly and R. S. Ruoff, Strength and Breaking Mechanism of Multiwalled Carbon Nanotubes Under Tensile Load, Science, 2000, 287(5453), 637–640, DOI:10.1126/science.287.5453.637.
- A. C. Gomez, M. Poot, G. A. Steele, H. S. J. Zant, N. Agraït and G. R. Bollinger, Elastic Properties of Freely Suspended MoS2 Nanosheets, Adv. Mater., 2012, 24(6), 772–775, DOI:10.1002/adma.201103965.
- S. Bertolazzi, J. Brivio and A. Kis, Stretching and Breaking of Ultrathin MoS2, ACS Nano, 2011, 5(12), 9703–9709, DOI:10.1021/nn203879f.
- K. Liu, Q. Yan, M. Chen, W. Fan, Y. Sun, J. Suh, D. Fu, S. Lee, J. Zhou, S. Tongay, J. Ji, J. B. Neaton and J. Wu, Elastic Properties of Chemical-Vapor-Deposited Monolayer MoS2, WS2, and Their Bilayer Heterostructures, Nano Lett., 2014, 14(9), 5097–5103 CrossRef CAS PubMed.
- S. M. Kim, A. Hsu, M. H. Park, S. H. Chae, S. J. Yun, J. S. Lee, D. H. Cho, W. Fang, C. Lee, T. Palacios, M. Dresselhaus, K. K. Kim, Y. H. Lee and J. Kong, Synthesis of large-area multilayer hexagonal boron nitride for high material performance, Nat. Commun., 2015, 6, 8662 CrossRef CAS PubMed.
- V. Georgakilas, M. Otyepka, A. B. Bourlinos, V. Chandra, N. Kim, K. C. Kemp, P. Hobza, R. Zboril and K. S. Kim, Functionalization of Graphene: Covalent and Non-Covalent Approaches, Derivatives and Applications, Chem. Rev., 2012, 112, 6156–6214 CrossRef CAS PubMed.
- S. Stankovich, D. A. Dikin, G. H. B. Dommett, K. M. Kohlhaas, E. J. Zimney, E. A. Stach, R. D. Piner, S. T. Nguyen and R. S. Ruoff, Graphene-based composite materials, Nature, 2006, 442, 282–286, DOI:10.1038/nature04969.
- M. Nonahal, H. Rastin, M. R. Saeb and M. G. Sari,
et al., Epoxy/PAMAM dendrimer-modified graphene oxide nanocomposite coatings: nonisothermal cure kinetics study, Prog. Org. Coat., 2018, 114, 233–243, DOI:10.1016/j.porgcoat.2017.10.023.
- E. Yarahmadi, K. Didehban, M. G. Sari and M. R. Saeb,
et al., Development and curing potential of epoxy/starch-functionalized graphene oxide nanocomposite coatings, Prog. Org. Coat., 2018, 119, 194–202, DOI:10.1016/j.porgcoat.2018.03.001.
- S. H. Ryu, J. H. Sin and A. M. Shanmugharaj, Study on the effect of hexamethylene diamine functionalized graphene oxide on the curing kinetics of epoxy nanocomposites, Eur. Polym. J., 2014, 52, 88–97 CrossRef CAS.
- L. Li, Z. Zeng, H. Zou and M. Liang, Curing characteristics of an epoxy resin in the presence of functional graphite oxide with amine-rich surface, Thermochim. Acta, 2015, 614, 76–84 CrossRef CAS.
- R. Wazalwar and A. M. Raichur, Model-free cure kinetics of tetra-functional epoxy reinforced with GO and p-Phenylenediamine modified GO, Thermochim. Acta, 2021, 697, 178857, DOI:10.1016/j.tca.2020.178857.
- Y. J. Wan, L. C. Tang, D. Yan, L. Zhao, Y. B. Li, L. B. Wu, J. X. Jiang and G. Q. Lai, Improved dispersion and interface in the graphene/epoxy composites via a facile surfactant-assisted process, Compos. Sci. Technol., 2013, 82, 60–68, DOI:10.1016/j.compscitech.2013.04.009.
- J. Zang, Y. J. Wan, L. Zhao and L. C. Tang, Fracture Behaviors of TRGO-Filled Epoxy Nanocomposites with Different Dispersion/Interface Levels, Macromol. Mater. Eng., 2017, 300(15), 737–749, DOI:10.1002/mame.201400437.
- J. Wei, M. S. Saharudin, T. Vo and F. Inam, Effects of surfactants on the properties of epoxy/graphene nanocomposites, J. Reinf. Plast. Compos., 2018, 37(14), 960–967, DOI:10.1177/0731684418765369.
- J. W. Yu, J. Jung, Y. M. Choi, J. H. Choi, J. Yu, J. K. Lee, N. H. You and M. Goh, Enhancement of the crosslink density, glass transition temperature, and strength of epoxy resin by using functionalized graphene oxide co-curing agents, Polym. Chem., 2016, 7, 36–43, 10.1039/c5py01483b.
- H. Ribeiro, W. M. da Silva, J. C. Neves, H. D. R. Calado, R. Paniago, L. M. Seara, D. M. Camarano and G. G. Silva, Multifunctional nanocomposites based on tetraethylenepentamine-modified graphene oxide/epoxy, Polym. Test., 2015, 43, 182–192, DOI:10.1016/j.polymertesting.2015.03.010.
- S. Chatterjee, J. W. Wang, W. S. Kuo, N. H. Tai, C. Salzmann, W. L. Li, R. Hollertz, F. A. Nüesch and B. T. T. Chu, Mechanical reinforcement and thermal conductivity in expanded graphene nanoplatelets reinforced epoxy composites, Chem. Phys. Lett., 2012, 531, 6–10, DOI:10.1016/j.cplett.2012.02.006.
- Y. J. Wan, L. X. Gong, L. C. Tang, L. B. Wu and J. X. Jiang, Mechanical properties of epoxy composites filled with silane-functionalized graphene oxide, Composites, Part A, 2014, 64, 79–89, DOI:10.1016/j.compositesa.2014.04.023.
- X. Wang, W. Xing, P. Zhang, L. Song, H. Yang and Y. Hu, Covalent functionalization of graphene with organosilane and its use as a reinforcement in epoxy composites, Compos. Sci. Technol., 2012, 72(6), 737–743, DOI:10.1016/j.compscitech.2012.01.027.
- M. Huskić, S. Bolka, A. Vesel, M. Mozetič, A. Anžlovar, A. Vizintin and E. Žagar, One-step surface modification of graphene oxide and influence of its particle size on the properties of graphene oxide/epoxy resin nanocomposites, Eur. Polym. J., 2018, 101, 211–217 CrossRef.
- Y. Wei, X. Hu, Q. Jiang, Z. Sun, P. Wang, Y. Qiu and W. Liu, Influence of graphene oxide with different oxidation levels on the properties of epoxy composites, Compos. Sci. Technol., 2018, 161, 74–84, DOI:10.1016/j.compscitech.2018.04.007.
- Y. J. Wan, L. C. Tang, L. X. Gong, D. Yan, Y. B. Li, L. B. Wu, J. X. Jiang and G. Q. Lai, Grafting of epoxy chains onto graphene oxide for epoxy composites with improved mechanical and thermal properties, Carbon, 2014, 69, 67–80, DOI:10.1016/j.carbon.2013.12.050.
- J. Li, W. Zhu, S. Zhang, Q. Gao, J. Li and W. Zhang, Amine-terminated hyperbranched polyamide covalent functionalized graphene oxide-reinforced epoxy nanocomposites with enhanced toughness and mechanical properties, Polym. Test., 2019, 76, 232–244, DOI:10.1016/j.polymertesting.2019.03.017.
- M. Sahu and A. M. Raichur, Toughening of high performance tetrafunctional epoxy with poly(allyl amine) grafted graphene oxide, Composites, Part B, 2019, 168, 15–24, DOI:10.1016/j.compositesb.2018.12.030.
- W. Li, A. Dichiara and J. Bai, Carbon nanotube–graphene nanoplatelet hybrids as high-performance multifunctional reinforcements in epoxy composites, Compos. Sci. Technol., 2013, 74, 221–227, DOI:10.1016/j.compscitech.2012.11.015.
- N. Domun, K. R. Paton, H. Hadavinia, T. Sainsbury, T. Zhang and H. Mohamud, Enhancement of Fracture Toughness of Epoxy Nanocomposites by Combining Nanotubes and Nanosheets as Fillers, Materials, 2017, 10(10), 1179, DOI:10.3390/ma10101179.
- S. Y. Yang, W. N. Lin, Y. L. Huang, H. W. Tien, J. Y. Wang, C. C. M. Ma, S. M. Li and Y. S. Wang, Synergetic effects of graphene platelets and carbon nanotubes on the mechanical and thermal properties of epoxy composites, Carbon, 2011, 49(3), 793–803, DOI:10.1016/j.carbon.2010.10.014.
- D. Quan, D. Carolan, C. Rouge, N. Murphy and A. Ivankovic, Mechanical and fracture properties of epoxy adhesives modified with graphene nanoplatelets and rubber particles, Int. J. Adhes. Adhes., 2018, 81, 21–29, DOI:10.1016/j.ijadhadh.2017.09.003.
- F. Wang, L. T. Drzal, Y. Qin and Z. Huang, Enhancement of fracture toughness, mechanical and thermal properties of rubber/epoxy composites by incorporation of graphene nanoplatelets, Composites, Part A, 2016, 87, 10–22, DOI:10.1016/j.compositesa.2016.04.009.
- Y. Che, Z. Sun, R. Zhan, S. Wang, S. Zhou and J. Huang, Effects of graphene oxide sheets-zirconia spheres nanohybrids on mechanical, thermal and tribological performances of epoxy composites, Ceram. Int., 2018, 44(15), 18067–18077, DOI:10.1016/j.ceramint.2018.07.010.
- L. Chen, S. Chai, K. Liu, N. Ning, J. Gao, Q. Liu, F. Chen and Q. Fu, Enhanced Epoxy/Silica Composites Mechanical Properties by Introducing Graphene Oxide to the Interface, ACS Appl. Mater. Interfaces, 2012, 4(8), 4398–4404, DOI:10.1021/am3010576.
- V. C. Doan, M. C. Vu, M. A. Islam and S. R. Kim, Poly(methyl methacrylate)-functionalized reduced graphene oxide-based core–shell structured beads for thermally conductive epoxy composites, J. Appl. Polym. Sci., 2019, 136(9), 47377, DOI:10.1002/app.47377.
- Y. Zhang, K. Y. Rhee and S. J. Park, Nanodiamond nanocluster-decorated graphene oxide/epoxy nanocomposites with enhanced mechanical behavior and thermal stability, Composites, Part B, 2017, 114, 111–120, DOI:10.1016/j.compositesb.2017.01.051.
- J. S. Lewis, Z. Barani, A. S. Magana, F. Kargar and A. A. Balandin, Thermal and electrical conductivity control in hybrid composites with graphene and boron nitride fillers, Mater. Res. Express, 2019, 6, 085325 CrossRef CAS.
- P. Chen, X. Liang and Y. Zhou,
et al., Effective reinforcement of amino-functionalized molybdenum disulfide on epoxy-based composites via strengthened interfacial interaction, J. Mater. Sci., 2018, 53, 8221–8231, DOI:10.1007/s10853-018-2153-3.
- S. Chhetri, N. C. Adak, P. Samanta, N. Mandal, T. Kuila and N. C. Murmu, Investigation of mechanical and thermal properties of the cetyltrimethylammonium bromide functionalized molybdenum disulfide (MoS2)/epoxy composites, Polym. Bull., 2018, 75, 327–343 CrossRef CAS.
- M. Zhao, L. Liu, B. Zhang, M. Sun, X. Zhang, X. Zhang, J. Li and L. Wang, Epoxy composites with functionalized molybdenum disulfide nanoplatelet additives, RSC Adv., 2018, 8, 35170–35178, 10.1039/c8ra07448h.
- S. Presolski and M. Pumera, Covalent functionalization of MoS2, Mater. Today, 2016, 19(3), 140–145, DOI:10.1016/j.mattod.2015.08.019.
- S. S. Chou, M. De, J. Kim, S. Byun, C. Dykstra, J. Yu, J. Huang and V. P. Dravid, Ligand Conjugation of Chemically Exfoliated MoS2, J. Am. Chem. Soc., 2013, 135(12), 4584–4587 CrossRef CAS PubMed.
- J. S. Kim, H. W. Yoo, H. O. Choi and H. T. Jung, Tunable Volatile Organic Compounds Sensor by Using Thiolated Ligand Conjugation on MoS2, Nano Lett., 2014, 14(10), 5941–5947, DOI:10.1021/nl502906a.
- D. Voiry, A. Goswami and R. Kappera,
et al., Covalent functionalization of monolayered transition metal dichalcogenides by phase engineering, Nat. Chem., 2015, 7, 45–49, DOI:10.1038/nchem.2108.
- D. Quan and A. Ivankovic, Effect of core–shell rubber (CSR) nano-particles on mechanical properties and fracture toughness of an epoxy polymer, Polymer, 2015, 66, 16–28 CrossRef CAS.
- T. H. Hsieh, A. J. Kinloch, K. Masania, J. Sohn Lee, A. C. Taylor and S. Sprenger, The toughness of epoxy polymers and fibre composites modified with rubber microparticles and silica nanoparticles, J. Mater. Sci., 2010, 45, 1193–1210, DOI:10.1007/s10853-009-4064-9.
- A. Keller, H. M. Chong, A. C. Taylor, C. Dransfeld and K. Masania, Core-shell rubber nanoparticle reinforcement and processing of high toughness fast-curing epoxy composites, Compos. Sci. Technol., 2018, 147, 78–88, DOI:10.1016/j.compscitech.2017.05.002.
- P. Dittanet and R. A. Pearson, Effect of silica nanoparticle size on toughening mechanisms of filled epoxy, Polymer, 2012, 53, 1890–1905, DOI:10.1016/j.polymer.2012.02.052.
- T. Prasad, S. Halder and S. S. Dhar, Imidazole-supported silica one-pot processed nanoparticles to enhance toughness of epoxy based nanocomposites, Mater. Chem. Phys., 2019, 231, 75–86, DOI:10.1016/j.matchemphys.2019.04.002.
- P. C. Ma, N. A. Siddiqui, G. Marom and J. K. Kim, Dispersion and functionalization of carbon nanotubes for polymer-based nanocomposites: a review, Composites, Part A, 2010, 41(10), 1345–1367, DOI:10.1016/j.compositesa.2010.07.003.
- N. Kadhim, Y. Mei, Y. Wang, Y. Li, F. Meng, M. Jiang and Z. Zhou, Remarkable Improvement in the Mechanical Properties of Epoxy Composites Achieved by a Small Amount of Modified Helical Carbon Nanotubes, Polymers, 2018, 10(10), 1103, DOI:10.3390/polym10101103.
- Y. T. Park, Y. Qian, C. Chan, T. Suh, M. G. Nejhad, C. W. Macosko and A. Stein, Epoxy Toughening with Low Graphene Loading, Adv. Funct. Mater., 2015, 25(4), 575–585, DOI:10.1002/adfm.201402553.
- G. Xue, B. Zhang, M. Sun, X. Zhang, J. Li, L. Wang and C. Song, Morphology, thermal and mechanical properties of epoxy adhesives containing well-dispersed graphene oxide, Int. J. Adhes. Adhes., 2019, 88, 11–18, DOI:10.1016/j.ijadhadh.2018.10.011.
- J. M. V. Moreno, V. Y. Sanchez, R. S. Hidalgo, R. Verdejo, M. A. L. Manchado, L. F. García, C. Blanco and R. Menéndez, Customizing thermally-reduced graphene oxides for electrically conductive or mechanical reinforced epoxy nanocomposites, Eur. Polym. J., 2017, 93, 1–7, DOI:10.1016/j.eurpolymj.2017.05.026.
- S. Wu, R. B. Ladani, J. Zhang, E. Bafekrpour, K. Ghorbani, A. P. Mouritz, A. J. Kinloch and C. H. Wang, Aligning multilayer graphene flakes with an external electric field to improve multifunctional properties of epoxy nanocomposites, Carbon, 2015, 94, 607–618, DOI:10.1016/j.carbon.2015.07.026.
- M. A. Rafiee, J. Rafiee, Z. Wang, H. Song, Z. Z. Yu and N. Koratkar, Enhanced Mechanical Properties of Nanocomposites at Low Graphene Content, ACS Nano, 2009, 3(12), 3884–3890, DOI:10.1021/nn9010472.
- J. Wei, R. Atif, T. Vo and F. Inam, Graphene Nanoplatelets in Epoxy System: Dispersion, Reaggregation, and Mechanical Properties of Nanocomposites, J. Nanomater., 2015, 561742, DOI:10.1155/2015/561742.
- S. Chandrasekaran, N. Sato, F. Tölle, R. Mülhaupt, B. Fiedler and K. Schulte, Fracture toughness and failure mechanism of graphene based epoxy composites, Compos. Sci. Technol., 2014, 97, 90–99, DOI:10.1016/j.compscitech.2014.03.014.
- L. C. Tang, Y. J. Wan, D. Yan, Y. B. Pei, L. Zhao, Y. B. Li, L. B. Wu, J. X. Jiang and G. Q. Lai, The effect of graphene dispersion on the mechanical properties of graphene/epoxy composites, Carbon, 2013, 60, 16–27, DOI:10.1016/j.carbon.2013.03.050.
- H. Yang, C. Shan, F. Li, Q. Zhang, D. Han and L. Niu, Convenient preparation of tunably loaded chemically converted graphene oxide/epoxy resin nanocomposites from graphene oxide sheets through two phase extraction, J. Mater. Chem., 2009, 19, 8856–8860, 10.1039/b915228h.
- M. A. Rafiee, J. Rafiee, I. Srivastava, Z. Wang, H. Song, Z. Z. Yu and N. Koratkar, Fracture and Fatigue in Graphene Nanocomposites, Small, 2010, 6(2), 179–183, DOI:10.1002/smll.200901480.
- D. R. Bortz, E. G. Heras and I. M. Gullon, Impressive Fatigue Life and Fracture Toughness Improvements in Graphene Oxide/Epoxy Composites, Macromolecules, 2012, 45(1), 238–245, DOI:10.1021/ma201563k.
- S. H. Muñoz, M. D. C. S. Moreno, G. J. M. Domínguez, P. A. M. Rodríguez and E. Vázquez, Experimental, Numerical, and Analytical Study on The Effect of Graphene Oxide in The Mechanical Properties of a Solvent-Free Reinforced Epoxy Resin, Polymers, 2019, 11(12), 2115, DOI:10.3390/polym11122115.
- W. S. Kang, K. Y. Rhee and S. J. Park, Influence of surface energetics of graphene oxide on fracture toughness of epoxy nanocomposites, Composites, Part B, 2017, 114, 175–183, DOI:10.1016/j.compositesb.2017.01.032.
- X. Tang, Y. Zhou and M. Peng, Green Preparation of Epoxy/Graphene Oxide Nanocomposites Using a Glycidylamine Epoxy Resin as the Surface Modifier and Phase Transfer Agent of Graphene Oxide, ACS Appl. Mater. Interfaces, 2016, 8(3), 1854–1866, DOI:10.1021/acsami.5b09830.
- F. V. Ferreira, F. S. Brito, W. Franceschi, E. A. N. Simonetti, L. S. Cividanes, M. Chipara and K. Lozano, Functionalized graphene oxide as reinforcement in epoxy based nanocomposites, Surf. Interfaces, 2018, 10, 100–109, DOI:10.1016/j.surfin.2017.12.004.
- A. Ashori, H. Rahmani and R. Bahrami, Preparation and characterization of functionalized graphene oxide/carbon fiber/epoxy nanocomposites, Polym. Test., 2015, 48, 82–88, DOI:10.1016/j.polymertesting.2015.09.010.
- M. Fang, Z. Zhang, J. Li, H. Zhang, H. Lu and Y. Yang, Constructing hierarchically structured interphases for strong and tough epoxy nanocomposites by amine-rich graphene surfaces, J. Mater. Chem., 2010, 20, 9635–9643, 10.1039/c0jm01620a.
- M. Seong and D. S. Kim, Effects of facile amine-functionalization on the physical properties of epoxy/graphene nanoplatelets nanocomposites, J. Appl. Polym. Sci., 2015, 132(58), 42269, DOI:10.1002/app.42269.
- M. Naebe, J. Wang, A. Amini, H. Khayyam, N. Hameed, L. H. Li, Y. Chen and B. Fox, Mechanical Property and Structure of Covalent Functionalised Graphene/Epoxy Nanocomposites, Sci. Rep., 2015, 4, 4375, DOI:10.1038/srep04375.
- S. Guo, L. Ma, G. Song, X. Li, P. Li, M. Wang, L. Shi, Z. Gu and Y. Huang, Covalent grafting of triazine derivatives onto graphene oxide for preparation of epoxy composites with improved interfacial and mechanical properties, J. Mater. Sci., 2018, 53, 16318–16330, DOI:10.1007/s10853-018-2788-0.
- S. Hou, S. Su, M. L. Kasner, P. Shah, K. Patel and C. J. Madarang, Formation of highly stable dispersions of silane-functionalized reduced graphene oxide, Chem. Phys. Lett., 2010, 501(1–3), 68–74, DOI:10.1016/j.cplett.2010.10.051.
- S. Pourhashem, A. Rashidi, M. R. Vaezia and M. R. Bagherzadeh, Excellent corrosion protection performance of epoxy composite coatings filled with amino-silane functionalized graphene oxide, Surf. Coat. Technol., 2017, 317, 1–9, DOI:10.1016/j.surfcoat.2017.03.050.
- S. Pourhashem, M. R. Vaezi, A. Rashidi and M. R. Bagherzadeh, Distinctive roles of silane coupling agents on the corrosion inhibition performance of graphene oxide in epoxy coatings, Prog. Org. Coat., 2017, 111, 47–56, DOI:10.1016/j.porgcoat.2017.05.008.
- Z. Li, R. Wang, R. J. Young, L. Deng, F. Yang, L. Hao, W. Jiao and W. Liu, Control of the functionality of graphene oxide for its application in epoxy nanocomposites, Polymer, 2013, 54(23), 6437–6446, DOI:10.1016/j.polymer.2013.09.054.
- B. A. Moghadam, M. Sharafimasooleh, S. Shadlou and F. Taheri, Effect of functionalization of graphene nanoplatelets on the mechanical response of graphene/epoxy composites, Mater. Des., 2014, 66(part A), 142–149, DOI:10.1016/j.matdes.2014.10.0.
- H. J. Salavagione, M. A. Gómez and G. Martínez, Polymeric Modification of Graphene through Esterification of Graphite Oxide and Poly(vinyl alcohol), Macromolecules, 2009, 42 17, 6331–6334, DOI:10.1021/ma900845w.
- M. Fang, K. Wang, H. Lu, Y. Yang and S. Nutt, Covalent polymer functionalization of graphene nanosheets and mechanical properties of composites, J. Mater. Chem., 2009, 19, 7098–7105, 10.1039/b908220d.
- S. Zhao, H. Chang, S. Chen, J. Cui and Y. Yan, High-performance and multifunctional epoxy composites filled with epoxide-functionalized graphene, Eur. Polym. J., 2016, 84, 300–312, DOI:10.1016/j.eurpolymj.2016.09.036.
- Z. Qi, Y. Tan, L. Gao, C. Zhang, L. Wang and C. Xiao, Effects of hyperbranched polyamide functionalized graphene oxide on curing behaviour and mechanical properties of epoxy composites, Polym. Test., 2018, 71, 145–155, DOI:10.1016/j.polymertesting.2018.08.029.
- X. Mi, F. Wei, L. Zeng, L. Zhong, J. Zhang, D. Zhang and Y. Luo, Incorporation of hyperbranched polyamide-functionalized graphene oxide into epoxy for improving interfacial and mechanical properties, Polym. Int., 2019, 68(8), 1492–1501, DOI:10.1002/pi.5855.
- L. Z. Guan, Y. J. Wan, L. X. Gong, D. Yan, L. C. Tang, L. B. Wu, J. X. Jiang and G. Q. Lai, Toward effective and tunable interphases in graphene oxide/epoxy composites by grafting different chain lengths of polyetheramine onto graphene oxide, J. Mater. Chem. A, 2014, 2, 15058–15069, 10.1039/c4ta02429j.
- X. Zhao, Y. Li, W. Chen, S. Li, Y. Zhao and S. Du, Improved fracture toughness of epoxy resin reinforced with polyamide 6/graphene oxide nanocomposites prepared via in situ polymerization, Compos. Sci. Technol., 2019, 171, 180–189, DOI:10.1016/j.compscitech.2018.12.023.
- L. Pan, J. Ban, S. Lu, G. Chen, J. Yang, Q. Luo, L. Wu and J. Yu, Improving thermal and mechanical properties of epoxy composites by using functionalized graphene, RSC Adv., 2015, 5(74), 60596–60607, 10.1039/c5ra09410k.
- L. Ma, Y. Zhu, G. Wu, X. Li, C. Tian, Y. Wang, L. Xu and G. Song, Hydroxyl-Terminated Triazine Derivatives Grafted Graphene Oxide for Epoxy Composites: Enhancement of Interfacial and Mechanical Properties, Polymers, 2019, 1866, DOI:10.3390/polym11111866.
- J. S. Jayan, A. Saritha, B. D. S. Deeraj and K. Joseph, Graphene Oxide as a Prospective Graft in Polyethylene Glycol for Enhancing the Toughness of Epoxy Nanocomposites, Polym. Eng. Sci., 2020, 60(4), 773–781, DOI:10.1002/pen.25335.
- L. Hou, J. Gao, H. Ruan, X. Xu and S. Lu, Mechanical and thermal properties of hyperbranched poly(ε-caprolactone) modified graphene/epoxy composites, J. Polym. Res., 2020, 27, 32, DOI:10.1007/s10965-020-2008-x.
- J. S. Jayan, A. Saritha, B. D. S. Deeraj and K. Joseph, Triblock copolymer grafted Graphene oxide as nanofiller for toughening of epoxy resin, Mater. Chem. Phys., 2020, 248, 122930, DOI:10.1016/j.matchemphys.2020.122930.
- G. Xue, B. Zhang, J. Xing, M. Sun, X. Zhang, J. Li, L. Wang and C. Liu, A facile approach to synthesize in situ functionalized graphene oxide/epoxy resin nanocomposites: mechanical and thermal properties, J. Mater. Sci., 2019, 54, 13973–13989, DOI:10.1007/s10853-019-03901-1.
- J. Sun, J. Ji, Z. Chen, S. Liu and J. Zhao, Epoxy resin composites with commercially available graphene: toward high toughness and rigidity, RSC Adv., 2019, 9, 33147–33154, 10.1039/c9ra05992j.
- E. Husamelden and H. Fan, Fluorinated functionalization of graphene oxide and its role as a reinforcement in epoxy composites, J. Polym. Res., 2019, 26, 42, DOI:10.1007/s10965-018-1687-z.
- J. Kim, J. Cha, G. H. Jun, S. C. Yoo, S. Ryu and S. H. Hong, Fabrication of Graphene Nanoplatelet/Epoxy Nanocomposites for Lightweight and High-Strength Structural Applications, Part. Part. Syst. Charact., 2018, 35(6), 1700412, DOI:10.1002/ppsc.201700412.
- S. Chhetri, N. C. Adak, P. Samanta, P. K. Mallisetty, N. C. Murmu and T. Kuila, Interface engineering for the improvement of mechanical and thermal properties of covalent functionalized graphene/epoxy composites, J. Appl. Polym. Sci., 2018, 135, 46124, DOI:10.1002/app.46124.
- H. Yao, S. A. Hawkins and H. J. Sue, Preparation of epoxy nanocomposites containing well-dispersed graphene nanosheets, Compos. Sci. Technol., 2017, 146, 161–168, DOI:10.1016/j.compscitech.2017.04.026.
- R. Moosaei, M. Sharif and A. Ramezannezhad, Enhancement of tensile, electrical and thermal properties of epoxy nanocomposites through chemical hybridization of polypyrrole and graphene oxide, Polym. Test., 2017, 60, 173–186, DOI:10.1016/j.polymertesting.2017.03.022.
- P. Katti, K. V. Kundan, S. Kumar and S. Bose, Improved mechanical properties through engineering the interface by poly(ether ether ketone) grafted graphene oxide in epoxy based nanocomposites, Polymer, 2017, 122, 184–193, DOI:10.1016/j.polymer.2017.06.059.
- S. Jahandideh, M. J. S. Shirazi and M. Tavakoli, Mechanical and thermal properties of octadecylamine-functionalized graphene oxide reinforced epoxy nanocomposites, Fibers Polym., 2017, 18, 1995–2004, DOI:10.1007/s12221-017-7417-z.
- N. Domun, H. Hadavinia, T. Zhang, G. Liaghat, S. Vahid, C. Spacie, K. R. Paton and T. Sainsbury, Improving the fracture toughness properties of epoxy using graphene nanoplatelets at low filler content, Nanocomposites, 2017, 3(3), 85–96, DOI:10.1080/20550324.2017.1365414.
- P. Bari, S. Khan, J. Njuguna and S. Mishra, Elaboration of properties of graphene oxide reinforced epoxy nanocomposites, Int. J. Plast. Technol., 2017, 21, 194–208, DOI:10.1007/s12588-017-9180-9.
- Z. Hu, C. Song, Q. Shao, J. Li and Y. Huang, One-step functionalization of graphene by cycloaddition of diarylcarbene and its application as reinforcement in epoxy composites, Compos. Sci. Technol., 2016, 135, 21–27, DOI:10.1016/j.compscitech.2016.09.008.
- F. Wang, L. T. Drzal, Y. Qin and Z. Huang, Effects of functionalized graphene nanoplatelets on the morphology and properties of epoxy resins, High Perform. Polym., 2015, 525–536, DOI:10.1177/0954008315588983.
- M. G. Prolongo, C. Salom, C. Arribas, M. S. Cabezudo, R. M. Masegosa and S. G. Prolongo, Influence of graphene nanoplatelets on curing and mechanical properties of graphene/epoxy nanocomposites, J. Therm. Anal. Calorim., 2016, 125, 629–636, DOI:10.1007/s10973-015-5162-3.
- D. Galpaya, M. Wang, G. George, N. Motta, E. Waclawik and C. Yan, Preparation of graphene oxide/epoxy nanocomposites with significantly improved mechanical properties, J. Appl. Phys., 2014, 116, 053518, DOI:10.1063/1.4892089.
- N. Yousefi, X. Lin, Q. Zheng, X. Shen, J. R. Pothnis, J. Jia, E. Zussman and J. K. Kim, Simultaneous in situ reduction, self-alignment and covalent bonding in graphene oxide/epoxy composites, Carbon, 2013, 59, 406–417, DOI:10.1016/j.carbon.2013.03.034.
- K. S. Kim, I. Y. Jeon, S. N. Ahn, Y. D. Kwon and J. B. Baek, Edge-functionalized graphene-like platelets as co-curing agent and a nano scale additive to epoxy resin, J. Mater. Chem., 2011, 21, 7337–7342, 10.1039/c0jm03504a.
- O. Eksik, J. Gao, S. A. Shojaee, A. Thomas, P. Chow, S. F. Bartolucci, D. A. Lucca and N. Koratkar, Epoxy Nanocomposites with Two-Dimensional Transition Metal Dichalcogenide Additives, ACS Nano, 2014, 8(5), 5282–5289, DOI:10.1021/nn5014098.
- B. Chen, B. J. Ni, M. X. Fu, H. Zhong, W. F. Jiang, S. Y. Liu, H. X. Zhang and K. B. Yoon, Effect of Molybdenum Disulfide Exfoliation Conditions on the Mechanical Properties of Epoxy Nanocomposites, Chin. J. Polym. Sci., 2019, 37, 687–692, DOI:10.1007/s10118-019-2239-7.
- J. Hou, G. Li, N. Yang, L. Qin, M. E. Grami, Q. Zhang, N. Wang and X. Qu, Preparation and characterization of surface modified boron nitride epoxy composites with enhanced thermal conductivity, RSC Adv., 2014, 4, 44282–44290 RSC.
- K. Kim, M. Kim, Y. Hwang and J. Kim, Chemically modified boron nitride-epoxy terminated dimethylsiloxane composite for improving the thermal conductivity, Ceram. Int., 2014, 40, 2047–2056, DOI:10.1016/j.ceramint.2013.07.117.
- J. Han, G. Du, W. Gao and H. Bai, An Anisotropically High Thermal Conductive Boron Nitride/Epoxy Composite Based on Nacre-Mimetic 3D Network, Adv. Funct. Mater., 2019, 29(13), 1900412, DOI:10.1002/adfm.201900412.
- D. Lee, S. H. Song, J. Hwang, S. H. Jin, K. H. Park, B. H. Kim, S. H. Hong and S. Jeon, Enhanced Mechanical Properties of Epoxy Nanocomposites by Mixing Noncovalently Functionalized Boron Nitride Nanoflakes, Small, 2013, 9(15), 2602–2610, DOI:10.1002/smll.201203214.
- Y. Sliozberg, J. Andzelm, C. B. Hatter, B. Anasori, Y. Gogotsi and A. Hall, Interface binding and mechanical properties of MXene-epoxy nanocomposites, Compos. Sci. Technol., 2020, 192, 108124, DOI:10.1016/j.compscitech.2020.108124.
- H. Zhang, L. Wang and A. Zhou,
et al., Effects of 2-D transition metal carbide Ti2CTx on properties of epoxy composites, RSC Adv., 2016, 6, 87341–87352, 10.1039/c6ra14560d.
- B. Chen, B. J. Ni, W. T. Liu, Q. Y. Ye, S. Y. Liu, H. X. Zhang and K. B. Yoon, Mechanical properties of epoxy nanocomposites filled with melamine functionalized molybdenum disulfide, RSC Adv., 2018, 8, 20450–20455, 10.1039/c8ra02689k.
- J. Chen, B. Chen, J. Li, X. Tong, H. Zhao and L. Wang, Enhancement of mechanical and wear resistance performance in hexagonal boron nitride-reinforced epoxy nanocomposites, Polym. Int., 2017, 66(5), 659–664, DOI:10.1002/pi.5296.
- W. Li, J. Yuan, A. Dichiara, Y. Lin and J. Bai, The use of vertically aligned carbon nanotubes grown on SiC for in situ sensing of elastic and plastic deformation in electrically percolative epoxy composites, Carbon, 2012, 50(11), 4298–4301, DOI:10.1016/j.carbon.2012.05.011.
- L. Yue, G. Pircheraghi, S. A. Monemian and I. M. Zloczower, Epoxy composites with carbon nanotubes and graphene nanoplatelets – dispersion and synergy effects, Carbon, 2014, 78, 268–278, DOI:10.1016/j.carbon.2014.07.003.
- M. Bozlar, D. He, J. Bai, Y. Chalopin, N. Mingo and S. Volz, Carbon nanotube microarchitectures for enhanced thermal conduction at ultralow mass fraction in polymer composites, Adv. Mater., 2010, 22(14), 1654–1658, DOI:10.1002/adma.200901955.
- Y. Wu, Y. He, C. Chen, H. Li, Y. Xia and T. Zhou, MoS2-CNFs composites to enhance the anticorrosive and mechanical performance of epoxy coating, Prog. Org. Coat., 2019, 128, 178–186, DOI:10.1016/j.porgcoat.2019.01.021.
- Y. Xia, Y. He, C. Chen, Y. Wu and J. Chen, MoS2 nanosheets modified SiO2 to enhance the anticorrosive and mechanical performance of epoxy coating, Prog. Org. Coat., 2019, 132, 316–327, DOI:10.1016/j.porgcoat.2019.04.002.
- H. Ribeiro, J. P. C. Trigueiro, W. M. Silva and C. F. Woellner,
et al., Hybrid MoS2/h-BN Nanofillers As Synergic Heat Dissipation and Reinforcement Additives in Epoxy Nanocomposites, ACS Appl. Mater. Interfaces, 2019, 11(27), 24485–24492, DOI:10.1021/acsami.7b09945.
- W. Yao, R. Mao, W. Gao, W. Chen, Z. Xu and C. Gaoa, Piezoresistive effect of superelastic graphene aerogel spheres, Carbon, 2020, 158, 418–425, DOI:10.1016/j.carbon.2019.11.005.
- C. Chi, H. Xu, K. Zhang, Y. Wang, S. Zhang, X. Liu, X. Liu, J. Zhao and Y. Li, 3D hierarchical porous graphene aerogels for highly improved adsorption and recycled capacity, Mater. Sci. Eng., B, 2015, 194, 62–67, DOI:10.1016/j.mseb.2014.12.026.
- G. Gorgolis and C. Galiotis, Graphene aerogels: a review, 2D Mater., 2017, 4, 032001, DOI:10.1088/2053-1583/aa7883.
- G. Lian, C. C. Tuan, L. Li, S. Jiao, Q. Wang, K. S. Moon, D. Cui and C. P. Wong, Vertically Aligned and Interconnected Graphene Networks for High Thermal Conductivity of Epoxy Composites with Ultralow Loading, Chem. Mater., 2016, 28(17), 6096–6104, DOI:10.1021/acs.chemmater.6b01595.
- Y. H. Zhao, Y. F. Zhang and S. L. Bai, High thermal conductivity of flexible polymer composites due to synergistic effect of multilayer graphene flakes and graphene foam, Composites, Part A, 2016, 85, 148–155, DOI:10.1016/j.compositesa.2016.03.021.
- H. C. Bidsorkhi, A. G. D'Aloia, A. Tamburrano, G. D. Bellis, A. Delfini, P. Ballirano and M. S. Sarto, 3D Porous Graphene Based Aerogel for Electromagnetic Applications, Sci. Rep., 2019, 9, 15719, DOI:10.1038/s41598-019-52230-5.
- G. Tang, Z. G. Jiang, X. Li, H. B. Zhang, A. Dasari and Z. Z. Yu, Three dimensional graphene aerogels and their electrically conductive composites, Carbon, 2014, 77, 592–599, DOI:10.1016/j.carbon.2014.05.063.
- H. Zhou, H. Wang, X. Du, Y. Zhang, H. Zhou, H. Yuan, H. Y. Liu and Y. W. Mai, Facile fabrication of large 3D graphene filler modified epoxy composites with improved thermal conduction and tribological performance, Carbon, 2018, 139, 1168–1177, DOI:10.1016/j.carbon.2018.07.059.
- H. Zhou, H. Wang, X. Du, Y. Mo, H. Yuan and H. Y. Liu, Hybrid three-dimensional graphene fillers and graphite platelets to improve the thermal conductivity and wear performance of epoxy composites, Composites, Part A, 2019, 123, 270–277, DOI:10.1016/j.compositesa.2019.05.016.
- J. Kim, N. M. Han, J. Kim, J. Lee, J. K. Kim and S. Jeon, Highly Conductive and Fracture Resistant Epoxy Composite Based on Non-oxidized Graphene Flake Aerogel, ACS Appl. Mater. Interfaces, 2018, 10(43), 37507–37516, DOI:10.1021/acsami.8b13415.
- A. Kordi, S. Adibnazari and A. Imam, Effects of two- and three-dimensional graphene-based nanomaterials on the fatigue behavior of epoxy nanocomposites, Mater. Today Commun., 2020, 24, 101194, DOI:10.1016/j.mtcomm.2020.101194.
- N. M. Han, Z. Wang, X. Shen, Y. Wu, X. Liu, Q. Zheng, T. H. Kim, J. Yang and J. K. Kim, Graphene Size-Dependent Multifunctional Properties of Unidirectional Graphene Aerogel/Epoxy Nanocomposites, ACS Appl. Mater. Interfaces, 2018, 10(7), 6580–6592, DOI:10.1021/acsami.7b19069.
- X. Shen, Z. Wang and Y. Wu,
et al., A three-dimensional multilayer graphene web for polymer nanocomposites with exceptional transport properties and fracture resistance, Mater. Horiz., 2018, 5, 275–284, 10.1039/c7mh00984d.
- L. Embrey, P. Nautiyal, A. Loganathan, A. Idowu, B. Boesl and A. Agarwal, Three-Dimensional Graphene Foam Induces Multifunctionality in Epoxy Nanocomposites by Simultaneous Improvement in Mechanical, Thermal, and Electrical Properties, ACS Appl. Mater. Interfaces, 2017, 9(45), 39717–39727, DOI:10.1021/acsami.7b14078.
- S. Chandrasekaran, W. V. Liebig and M. Mecklenburg,
et al., Fracture, failure and compression behaviour of a 3D interconnected carbon aerogel (aerographite) epoxy composite, Compos. Sci. Technol., 2016, 122, 50–58, DOI:10.1016/j.compscitech.2015.11.002.
- Z. Wang, X. Shen, M. A. Garakani, X. Lin, Y. Wu, X. Liu, X. Sun and J. K. Kim, Graphene Aerogel/Epoxy Composites with Exceptional Anisotropic Structure and Properties, ACS Appl. Mater. Interfaces, 2015, 7(9), 5538–5549, DOI:10.1021/acsami.5b00146.
- J. Jia, X. Sun, X. Lin, X. Shen, Y. W. Mai and J. K. Kim, Exceptional Electrical Conductivity and Fracture Resistance of 3D Interconnected Graphene Foam/Epoxy Composites, ACS Nano, 2014, 8(6), 5774–5783, DOI:10.1021/nn500590g.
- R. Ladani, M. Bhasin, S. Wu, A. R. Ravindran, K. Ghorbani, J. Zhang, A. Kinloch, A. Mouritz and C. H. Wang, Fracture and Fatigue Behaviour of Epoxy Nanocomposites Containing 1-D and 2-D Nanoscale Carbon Fillers, Eng. Fract. Mech., 2018, 203, 102–114, DOI:10.1016/j.engfracmech.2018.04.033.
- X. Wang, J. Jin and M. Song, An investigation of the mechanism of graphene toughening epoxy, Carbon, 2013, 65, 324–333 CrossRef CAS.
- R. Wazalwar, N. Tripathi and A. M. Raichur, Mechanical and curing behavior of epoxy composites reinforced with polystyrene-graphene oxide (PS-GO) core-shell particles, Composites, Part C, 2021, 5, 100128, DOI:10.1016/j.jcomc.2021.100128.
- R. Atif, I. Shyha and F. Inam, The degradation of mechanical properties due to stress concentration caused by retained acetone in epoxy nanocomposites, RSC Adv., 2016, 6, 34188–34197, 10.1039/c6ra00739b.
- B. A. Moghadam and F. Taheri, Fracture and toughening mechanisms of GNP-based nanocomposites in modes I and II fracture, Eng. Fract. Mech., 2014, 131, 329–339, DOI:10.1016/j.engfracmech.2014.08.008.
- J. Kim, J. Kim, S. Song, S. Zhang, J. Cha, K. Kim, H. Yoon, Y. Jung, K. W. Paik and S. Jeon, Strength dependence of epoxy composites on the average filler size of non-oxidized graphene flake, Carbon, 2017, 113, 379–386 CrossRef CAS.
- N. D. Alexopoulos, Z. Paragkamian, P. Poulin and S. K. Kourkoulis, Fracture related mechanical properties of low and high graphene reinforcement of epoxy nanocomposites, Compos. Sci. Technol., 2017, 150, 194–204, DOI:10.1016/j.compscitech.2017.07.030.
- H. M. Chong, S. J. Hinder and A. C. Taylor, Graphene nanoplatelet-modified epoxy: effect of aspect ratio and surface functionality on mechanical properties and toughening mechanisms, J. Mater. Sci., 2016, 51, 8764–8790 CrossRef CAS.
- S. Chatterjee, F. Nafezarefi, N. H. Tai, L. Schlagenhauf, F. A. Nüesch and B. T. T. Chu, Size and synergy effects of nanofiller hybrids including graphene nanoplatelets and carbon nanotubes in mechanical properties of epoxy composites, Carbon, 2012, 50(15), 5380–5386, DOI:10.1016/j.carbon.2012.07.021.
- H. Lee, H. D. Yong, K. Kim, Y. Seo, H. Yun and S. W. Lee, Mechanical properties of rippled structure in suspended stacks of graphene, J. Appl. Phys., 2010, 108, 014302, DOI:10.1063/1.3456007.
- Q. Zhao and S. V. Hoa, Toughening Mechanism of Epoxy Resins with Micro/Nano Particles, J. Compos. Mater., 2007, 41(2), 201–219, DOI:10.1177/0021998306063361.
- M. M. Shokrieh, S. M. Ghoreishi, M. Esmkhani and Z. Zhao, Effects of graphene nanoplatelets and graphene nanosheets on fracture toughness of epoxy nanocomposites, Fatigue Fract. Eng. Mater. Struct., 2014, 37(10), 1116–1123, DOI:10.1111/ffe.12191.
- W. Kong, H. Kum and S. Bae,
et al., Path towards graphene commercialization from lab to market, Nat. Nanotechnol., 2019, 14, 927–938, DOI:10.1038/s41565-019-0555-2.
- Z. Qi, Y. Tan, Z. Zhang, L. Gao, C. Zhang and J. Tian, Synergistic effect of functionalized graphene oxide and carbon nanotube hybrids on mechanical properties of epoxy composites, RSC Adv., 2018, 8, 38689–38700, 10.1039/c8ra08312f.
- Y. Li, H. Zhang, M. Crespo, H. Porwal, O. Picot, G. Santagiuliana, Z. Huang, E. Barbieri, N. M. Pugno, T. Peijs and E. Bilotti, In Situ Exfoliation of Graphene in Epoxy Resins: A Facile Strategy to Efficient and Large Scale Graphene Nanocomposites, ACS Appl. Mater. Interfaces, 2016, 8(36), 24112–24122, DOI:10.1021/acsami.6b07492.
- Y. Li, H. Zhang, H. Porwal, Z. Huang, E. Bilotti and T. Peijs, Mechanical, electrical and thermal properties of in situ exfoliated graphene/epoxy nanocomposites, Composites, Part A, 2017, 95, 229–236, DOI:10.1016/j.compositesa.2017.01.007.
- Z. Li, R. J. Young, R. Wang, F. Yang, L. Hao, W. Jiao and W. Liu, The role of functional groups on graphene oxide in epoxy nanocomposites, Polymer, 2013, 54(21), 5821–5829, DOI:10.1016/j.polymer.2013.08.026.
- R. Wang, Z. Li, W. Liu, W. Jiao, L. Hao and F. Yang, Attapulgite–graphene oxide hybrids as thermal and mechanical reinforcements for epoxy composites, Compos. Sci. Technol., 2013, 87, 29–35, DOI:10.1016/j.compscitech.2013.08.002.
- I. Zaman, H. C. Kuan, J. Dai, N. Kawashima, A. Michelmore, A. Sovi, S. Dong, L. Luong and J. Ma, From carbon nanotubes and silicate layers to graphene platelets for polymer nanocomposites, Nanoscale, 2012, 4, 4578–4586, 10.1039/c2nr30837a.
- C. Monteserín, M. Blanco, E. Aranzabe, A. Aranzabe, J. M. Laza, A. L. Varga and J. L. Vilas, Effects of Graphene Oxide and Chemically-Reduced Graphene Oxide on the Dynamic Mechanical Properties of Epoxy Amine Composites, Polymers, 2017, 9, 449, DOI:10.3390/polym9090449.
- K. S. Novoselov, A. K. Geim and S. V. Morozov,
et al., Electric Field Effect in Atomically Thin Carbon Films, Science, 2004, 306(5696), 666–669, DOI:10.1126/science.1102896.
-
J. Fenn, and M. Raskino, Mastering the Hype Cycle: How to Choose the Right Innovation at the Right Time, Harvard Business Press, 2008 Search PubMed.
- T. Barkan, Graphene: The Hype Versus Commercial Reality, Nat. Nanotechnol., 2019, 14(10), 904–906, DOI:10.1038/s41565-019-0556-1.
-
https://www.iso.org/standard/66188.html
.
- B. L. Dasari, J. M. Nouria, D. Brabazon and S. Naher, Graphene and derivatives – Synthesis techniques, properties and their energy applications, Energy, 2017, 140, 766–778 CrossRef CAS.
- Y. Zhang, S. F. Ali, E. Dervishi, Y. Xu, Z. Li, D. Casciano and A. S. Biris, Cytotoxicity Effects of Graphene and Single-Wall Carbon Nanotubes in Neural Phaeochromocytoma-Derived PC12 Cells, ACS Nano, 2010, 4(6), 3181–3186, DOI:10.1021/nn1007176.
- K. Yang, J. Wan, S. Zhang, Y. Zhang, S. T. Lee and Z. Liu, In Vivo Pharmacokinetics, Long-Term Biodistribution, and Toxicology of PEGylated Graphene in Mice, ACS Nano, 2011, 5(1), 516–522, DOI:10.1021/nn1024303.
- A. B. Seabra, A. J. Paula and R. de Lima,
et al., Nanotoxicity of Graphene and Graphene Oxide, Chem. Res. Toxicol., 2014, 27(2), 159–168, DOI:10.1021/tx400385x.
- O. Akhavan and E. Ghaderi, Toxicity of Graphene and Graphene Oxide Nanowalls Against Bacteria, ACS Nano, 2010, 4(10), 5731–5736, DOI:10.1021/nn101390x.
- A. Schinwald, F. A. Murphy, A. Jones, W. MacNee and K. Donaldson, Graphene-based nanoplatelets: a new risk to the respiratory system as a consequence of their unusual aerodynamic properties, ACS Nano, 2012, 6(1), 736–746, DOI:10.1021/nn204229f.
- O. Akhavan, E. Ghaderi and A. Akhavan, Size-dependent genotoxicity of graphene nanoplatelets in human stem cells, Biomaterials, 2012, 33(32), 8017–8025, DOI:10.1016/j.biomaterials.2012.07.040.
- M. C. Matesanz, M. Vila and M. J. Feito,
et al., The effects of graphene oxide nanosheets localized on F-actin filaments on cell-cycle alterations, Biomaterials, 2013, 34(5), 1562–1569, DOI:10.1016/j.biomaterials.2012.11.001.
- M. Pelin, L. Fusco and V. León,
et al., Differential cytotoxic effects of graphene and graphene oxide on skin keratinocytes, Sci. Rep., 2017, 7, 40572, DOI:10.1038/srep40572.
- D. Ali, S. Alarifi, S. Alkahtani and R. S. Almeer, Silver-doped graphene oxide nanocomposite triggers cytotoxicity and apoptosis in human hepatic normal and carcinoma cells, Int. J. Nanomed., 2018, 13, 5685–5699, DOI:10.2147/IJN.S165448.
- S. Gurunathan, M. H. Kang, M. Jeyaraj and J. H. Kim, Differential Cytotoxicity of Different Sizes of Graphene Oxide Nanoparticles in Leydig (TM3) and Sertoli (TM4) Cells, Nanomaterials, 2019, 9(2), 139, DOI:10.3390/nano9020139.
- M. Wojtoniszak, X. Chen and R. J. Kalenczuk,
et al., Synthesis, dispersion, and cytocompatibility of graphene oxide and reduced graphene oxide, Colloids Surf., B, 2012, 89, 79–85, DOI:10.1016/j.colsurfb.2011.08.026.
- Y. Chang, S. T. Yang and J. H. Liu,
et al., In vitro toxicity evaluation of graphene oxide on A549 cells, Toxicol. Lett., 2011, 200(3), 201–210, DOI:10.1016/j.toxlet.2010.11.016.
- S. Bengtson, K. Kling and A. M. Madsen,
et al., No cytotoxicity or genotoxicity of graphene and graphene oxide in murine lung epithelial FE1 cells in vitro, Environ. Mol. Mutagen., 2016, 57(6), 469–482 CrossRef CAS PubMed.
- F. Xiaoli, C. Qiyue and G. Weihong,
et al., Toxicology data of graphene-family nanomaterials: an update, Arch. Toxicol., 2020, 94, 1915–1939, DOI:10.1007/s00204-020-02717-2.
- B. Fadeel, C. Bussy, S. Merino, E. Vázquez, E. Flahaut and F. Mouchet,
et al., Safety Assessment of Graphene-Based Materials: Focus on Human Health and the Environment, ACS Nano, 2018, 12(11), 10582–10620 CrossRef CAS PubMed.
|
This journal is © The Royal Society of Chemistry 2021 |