DOI:
10.1039/D0NA00910E
(Paper)
Nanoscale Adv., 2021,
3, 4106-4118
Bi2O3 nano-flakes as a cost-effective antibacterial agent
Received
30th October 2020
, Accepted 4th June 2021
First published on 4th June 2021
Abstract
Bismuth oxide is an important bismuth compound having applications in electronics, photo-catalysis and medicine. At the nanoscale, bismuth oxide experiences a variety of new physico-chemical properties because of its increased surface to volume ratio leading to potentially new applications. In this manuscript, we report for the very first time the synthesis of bismuth oxide (Bi2O3) nano-flakes by pulsed laser ablation in liquids without any external assistance (no acoustic, electric field, or magnetic field). The synthesis was performed by irradiating, pure bismuth needles immerged in de-ionized water, at very high fluence ∼160 J cm−2 in order to be highly selective and only promote the growth of two-dimensional structures. The x- and y-dimensions of the flakes were around 1 μm in size while their thickness was 47.0 ± 12.7 nm as confirmed by AFM analysis. The flakes were confirmed to be α- and γ-Bi2O3 by SAED and Raman spectroscopy. By using this mixture of flakes, we demonstrated that the nanostructures can be used as antimicrobial agents, achieving a complete inhibition of Gram positive (MSRA) and Gram negative bacteria (MDR-EC) at low concentration, ∼50 ppm.
1 Introduction
When penicillin was discovered on September 1928 by Sir Alex Fleming, it changed the face of medicine.1,2 This “miracle drug,”, as it was known, was able to offer a cure for some of the most nefarious diseases. It was only in 1942 that penicillin could be produced at an industrial scale and started to really make an impact in people's daily life.1 However, due to the benefits of this new drug, doctors started prescribing it massively for all sorts of diseases and people started to self-medicate themselves. When antibiotics are overused or used inappropriately (i.e., when a patient does not finish a full course of treatment), it helps in the spread of resistant bacteria. After a course of antibiotics, some naturally resistant bacteria will be left behind, survival-of-the-fittest style; the more frequent antibiotics are used, the more prevalent these fiercer strains will become. According to the World Health Organization, the inappropriate use of antibiotics in animal husbandry is an underlying contributor to the emergence and spreading of antibiotic-resistant germs, and consequently the use of antibiotics as growth promoters in animal feeds should be restricted.3 Adjacent bacteria can also acquire resistance by mutating or exchanging genetic material with the resistant strains. When those treatment-resistant bacteria grow and multiply, they can lead to new infections that become difficult or even impossible to treat. It has been estimated that by 2050, antibiotic-resistant bugs could kill an estimated 10 million people each year. Bacterial infections arise almost everywhere from drinking water4,5 to medical implants.6 An answer to this problem could be found from nanoscience by investigating new morphologies of materials as new antibacterial agents.
Indeed, at the nanoscale, quantum effects and a huge surface to volume ratio are responsible for the new physico-bio-chemical properties of materials.7,8 Various materials as noble metals,9,10 transition elements,11 alloys,11,12 carbon-based,13 or metal-oxides14–17 have all been investigated for their nano-features. Due to these new properties at the nanoscale, treatments for bacterial infections have been developed. For example, gold (Au)9 and silver (Ag) nanoparticles (NPs)10 have been used to this aim and have shown great results. These chemical elements present naturally antimicrobial activity with a low associated cytotoxicity.18,19 These great results are not without drawbacks. Au and Ag are rare elements with abundances of 0.004 and 0.075 mg kg−1 in the Earth's crust,20 respectively. Consequently, their price is around 44
800 and 521 USD per kg,20 respectively; see Fig. 1. Therefore, any antibacterial treatment based on those expensive chemical elements is not affordable to a large population. This opens the following question: are there any materials achieving similar antibacterial results and being affordable? Some reports have suggested chemical elements such as selenium (Se)21 and tellurium (Te),22 and although being cheaper than gold and silver, they are still relatively expensive (21.4 and 63.8 USD per kg).20 Furthermore, these elements are extensively used in solar cell and electronic industries which keeps their price relatively high for medical applications. That is why the American Physical Society and the Materials Research Society have labelled these elements as Energy Critical Elements.
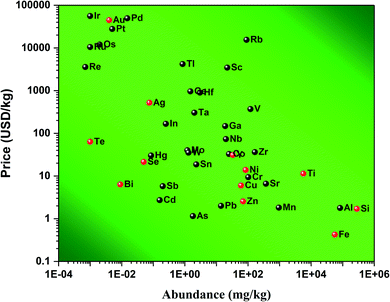 |
| Fig. 1 Price of various chemical elements versus their abundance in the Earth's crust. Data were collected from ref. 20. The chemical elements highlighted in red are common elements used for biomedical applications. | |
However, bismuth (Bi) seems to be an element of choice for biomedical applications.23,24 Many studies have demonstrated its applications in radio-sensing25 due to their high density which allows for better contrast imaging. When oxidized, bismuth forms bismuth(III) oxide, Bi2O3 which is known for its applications in electronics,26 photo-catalysis,27–32 medicine,33,34 gas sensing,35 water purification,31 and γ-ray shielding.36 As a photo-catalyst, Bi2O3 has been tested to photodegrade rhodamine B,30 methyl orange,27 rhodamine 6D, and other various water pollutants.31 In medicine, α-Bi2O3 coated with (3-aminopropyl)trimethoxysilane and methotrexate were shown to enhance radio-sensitizing features in dose enhancement radiation therapy.34 It was shown by Stewart et al.33 that α-Bi2O3 NPs have high radio-sensitizing capacity. Additionally, it was also shown that Bi2O3 is biocompatible.37 Besides those properties, Bi2O3 is a p-type semiconductor and polymorph material that exhibits six different crystal phases: α is monoclinic,38 β is tetragonal,39 γ is body-centered cubic,40 δ is face-centered cubic,41 ε is orthorhombic,42 and ω is triclinic.43 Each of these crystalline phases exhibits very distinct physico-chemical properties that allow for different potential applications, see Table 1. At room temperature, the most stable and common crystalline phase for Bi2O3 is the α-phase.
Table 1 Bismuth oxide (Bi2O3) physico-chemical properties
Phase |
Crystal structure |
Lattice parameters |
Energy bandgap (eV) |
Applications |
Ref. |
a (A) |
b (A) |
c (A) |
Not reported.
|
α |
Monoclinic |
5.848 |
8.166 |
7.510 |
2.8 |
Photo-catalysis |
38, 29 and 30 |
β |
Tetragonal |
7.730 |
— |
5.630 |
2.5 |
Water purification, photo-catalysis |
39, 29 and 31 |
γ |
b.c.c. |
10.268 |
— |
— |
1.9 |
|
40
|
δ |
f.c.c. |
5.644 |
— |
— |
1.7–2.0 |
Fuel cells, electronics |
41 and 44 |
ω |
Triclinic |
4.956 |
5.585 |
12.730 |
|
|
43
|
ε |
Orthorhombic |
7.269 |
8.639 |
11.970 |
|
|
42
|
Several methods have been proposed to synthesize Bi2O3 nanostructures of various sizes and shapes. These methods include: oxidative metal vapor transport deposition,45 sol–gel methods,46,47 wet-chemical synthesis,48 and pulsed laser ablation in liquids (PLAL).14–17,23,24,49 Of these methods, PLAL is the most non-equilibrium thermodynamic manufacturing process. Due to this, nanostructures produced by PLAL are influenced by a large number of parameters ranging from the type of target to the fluence of the laser.50 Most PLAL studies on Bi2O3 nanomaterials have been performed using lasers operating at low laser repetition rates and low laser fluence; consequently, spherical NPs were synthesized;14–17,23,24,51–53 see Table 2. Only one PLAL study, completed by Rosa et al.,52 was done at a higher repetition rate of 1.5 kHz with an ablation time of 2 minutes. It is the only study that has been completed at a high repetition rate, but the authors did not report the laser fluence or the power of the laser. The laser repetition rate strongly influences the final size and shape of the nanostructures due to the re-irradiation of the nanostructures floating within the colloidal solution during the synthesis.54 Those multiple irradiations induce fragmentation of the particles already produced by crossing the beam multiple times after their initial synthesis. By increasing the repetition rate, the concentration of particles within the solution also increases until the repetition rate is fast enough that the beam starts hitting the cavitation bubble formed during the previous irradiation.55 Another important parameter to consider is the target.54 Indeed, the initial starting shape of the target (powder or pellets) also influences the final size of the NPs.56 Pellets undergo an ablation process while a powder undergo mainly a fragmentation process.
Table 2 Bismuth oxide (Bi2O3) nanostructures by PLAL
Group |
Laser parameters |
Target parameters |
Products |
Ref. |
Laser type |
f (Hz) |
τ (ns) |
t (min) |
F (J cm−2) |
Target type |
Liquid |
V (ml) |
Given as energy per pulse.
Not reported.
|
Escobar-Alarcon |
355 nm Nd:YAG |
10 |
5 |
10 |
0.6–1.1 |
99.99% purity |
DI H2O |
|
α-Bi2O3 NPs |
15
|
Bi disk |
α,β-Bi2O3 NSs |
Gondal |
355 nm Nd:YAG |
|
|
40 |
|
99.998% purity Bi Powder |
H2O2 |
10 |
α-Bi2O3 NPs |
16
|
Dadashi |
1064 nm Nd:YAG |
10 |
12 |
5 |
118 mJ per pulsea |
99.99% purity |
DI H2O |
60 |
α,β-Bi2O3 NPs |
14
|
Ismail |
1064 nm Nd:YAG |
1 |
|
200 |
12 |
99.99% purity Bi |
DI H2O |
|
α-Bi2O3 NPs |
17
|
Rosa |
1064 nm Nd:YAG |
1500 |
200 |
2 |
|
99.999% purity Bi pellets |
DI H2O |
4 |
Bi NPs |
52
|
This work |
1064 nm Nd:YAG |
1000 |
100 |
5 |
160 |
99.97% purity Bi rods |
DI H2O |
7 |
Bi2O3 NFs |
|
Most recently, Dadashi et al.23 was able to combine PLAL of Bi-based-NP with a post process treatment coating them with gold for biomedical applications. Another recently published PLAL study detailed a new type of synthesis protocol called Sonication assisted-PLAL (S-PLAL); where without sonication the group was able to synthesize Bi2O3 nanoparticles, while with sonication (S-PLAL) they were able to synthesize Bi2O3 nano-sheets.15
To the best of our knowledge, this paper presents for the first time the synthesis of Bi2O3 nano-flakes (Bi2O3 NFs) by PLAL without any external field assistance (no acoustic, electric or magnetic field). This work includes characterization of these nano-flakes by Atomic Emission Spectroscopy (AES), Transmission Electron Microscopy (TEM) with Energy Dispersive Spectroscopy (EDS), Selected Area Electron Diffraction (SAED), X-ray Photoemission Spectroscopy (XPS), Atomic Force Microscopy (AFM), Scanning Electron Microscopy (SEM), and Raman Spectroscopy. After complete characterization, the nanostructures have been tested for their biomedical applications as antibacterial agents, towards both Gram-negative and -positive antibiotic-resistant strains.
2 Materials and methods
2.1 Synthesis of Bi2O3 NFs
A single bismuth needle of 99.97% purity from Alfa Aesar [LOT: N27E043] was placed at the bottom of a flat cylindrical beaker (Pyrex) and was covered with 7 mL of molecular biology grade de-ionized water (DI H2O) from Alfa Aesar [LOT: 177910]. The needle was then irradiated by a Q-Switched Nd:YAG laser from Electro Scientific Industries emitting in the infrared region at 1064 nm. The laser beam was reflected by a flat mirror angled at 45° with respect to the laser rail and then directed through an 83 mm lens which focused the beam onto the bismuth needle submerged in DI water. The beam size diameter at the surface of the target was measured to be around 100 μm. The laser power was measured in continuous mode to be 12.5 W. This gives a fluence of ∼160 J cm−2. The irradiation lasted for 5 minutes at a repetition rate of 1 kHz. The laser beam was hitting the same spot on the target during the entire duration of synthesis. After irradiation, the target (bismuth needle) was removed from the solution, which was dark brown in color, and a tiny dark spot was observed at the surface of the needle revealing the presence of the ablation crater. All samples were prepared for analysis just after the synthesis was completed.
2.2 Material characterization
After irradiation, the solution was poured into a 5 mL opaque plastic microtube for storage and further analysis. The solution was first analyzed by AES (AES, MP-4210 from Agilent). A droplet of the colloidal solution was then placed onto a silicon wafer for SEM analysis within a JEOL JSM7000F microscope. AFM (Bruker Icon AFM) was performed in tapping mode using a silicon AFM probe from Ted Pella, Inc [Prod No. TAP300-G-10] with a resonant frequency of 300 kHz and a force constant of 40 N m−1. A droplet was placed onto a carbon film copper grid for analysis by TEM performed in the JEOL JEM2100F and the JEOL ARM200F microscopes operated at 80 kV and 200 kV, respectively. The crystalline structure has been analyzed by SAED patterns. Off-axis electron holography has been performed to determine the thickness of the samples and compared with AFM measurements. In the optical measurement procedure (Raman spectroscopy), nano-flakes were first drop-casted onto a Si substrate. The sample was optically excited by an ultrafast laser (Coherent Chameleon Ultra II, 80 MHz, 150 fs) by a 633 nm continuous wave laser for Raman detection. The excitation spot and average power were 0.42 μm/1 mW for 633 nm laser, respectively. The Raman signal was filtered by appropriate long-pass filters, analyzed by a spectrometer (Horiba iHR550) and detected by a Charged-Coupled Device (CCD) camera. For XPS measurements, a drop of colloidal solution was placed onto a silicon wafer and then processed into a Thermo K-Alpha XPS.
2.3 Biomedical characterization
Two different strains of bacteria were tested for antimicrobial properties using the Bi2O3 NF: one Gram-negative bacteria (multidrug-resistant Escherichia coli (MDR-EC) (ATCC BAA-2471; ATCC, Manassas, VA)) and one Gram-Positive bacteria (Methicillin-resistant Staphylococcus aureus (MRSA) (ATCC 4330; ATCC, Manassas, VA)) were utilized for the antibacterial tests. The cultures were kept on agar plates at 4 °C.
Colony counting unit assays were completed by seeding the bacteria in a 96-well plate mixed with different concentrations of Bi2O3 NF. The plates were incubated at 37 °C for 8 h; after that period of time, they were removed from the incubator and diluted with PBS in a series of vials by ×104 ×105 and ×106. Three drops of 10 μL were taken of each dilution and deposited on an LB agar plate. The plates were deposited inside an incubator at 37 °C until the colonies grew enough without reaching confluency. Afterward, the numbers of colonies formed were counted, and the data was processed.
All biological experiments were repeated in triplicate (n = 3) to ensure the reliability of the results. Statistical significance was assessed using Student's t tests, with a p < 0.05 being statistically significant. Results are displayed as mean ± standard deviation.
3 Results
3.1 Effect of laser fluence on morphology
In order to better understand the effect of the laser fluence on the production of nanostructures by PLAL, a series of synthesizes were completed at ∼30, ∼100, and ∼160 J cm2; see Fig. 2a, b and c, respectively. From the SEM images, it is clear that at low laser fluence (Fig. 2a), a mixture of nano-spheres and nano-flakes were synthesized. As the laser fluence was increased, Fig. 2b and c, the spherical population was removed from solution and the nano-flakes were the only population observed. Besides the elimination of nano-spheres, another effect was noticed, in that the nano-flakes morphology became well defined as small squares. This morphology is totally unusual for the PLAL synthesis technique. Consequently, the nano-flakes synthesized at ∼160 J cm−2 were selected as the focus of the paper.
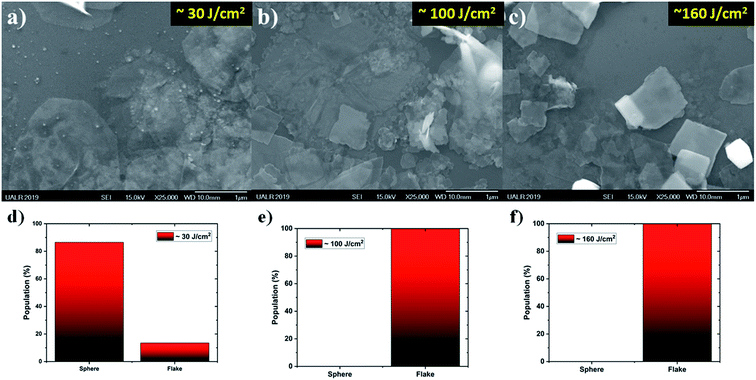 |
| Fig. 2 Effect of laser fluence on the morphology of nanostructures by PLAL ∼30 J cm−2, ∼100 J cm−2, and ∼160 J cm2; (a), (b) and (c), respectively, and distributions of morphologies for each fluence in (d), (e) and (f), respectively. | |
3.2 Physico-chemical characterization
In order to determine the morphology and chemical nature of the structures within the colloidal solution, TEM and additional SEM were performed. Fig. 3a and b, show low magnification TEM and SEM images of the structures after being drop-casted onto a copper grid and silicon wafer, respectively. From these observations, the “flake” morphology is determined. Fig. 3c shows a typical STEM image of the bismuth-based flakes. EDS was performed in order to identify the chemical nature of the flake. From the EDS analysis, the flake is made of bismuth and oxygen, as expected from the ablation process. Fig. 3d–f show the mapping of bismuth, oxygen, and bismuth plus oxygen, respectively. Bismuth and oxygen can combine according various stoichiometric ratio (1
:
1, 2
:
3, 2
:
5), so to confirm the exact stoichiometric ratio of the bismuth oxide flakes obtained by PLAL, XPS was performed (Fig. 3g and h). The Bi4f7 scan revealed a peak centered about 159 eV which has been attributed to the Bi–O bond corresponding to Bi2O3. The Bi4f5 scan revealed a peak centered about 165 eV which is also a peak that has been attributed to the Bi–O bond corresponding to Bi2O3. There are no peaks centered about 157 eV or 162 eV which are attributed to the Bi–Bi bond.57 In Fig. 3f, there is another peak centered on ∼531 eV which is commonly attributed to metal oxides. This further confirms the 2
:
3 stoichiometric ratio of our flakes. So clearly, Bi2O3 is the material compound constituting the surface of the flakes.
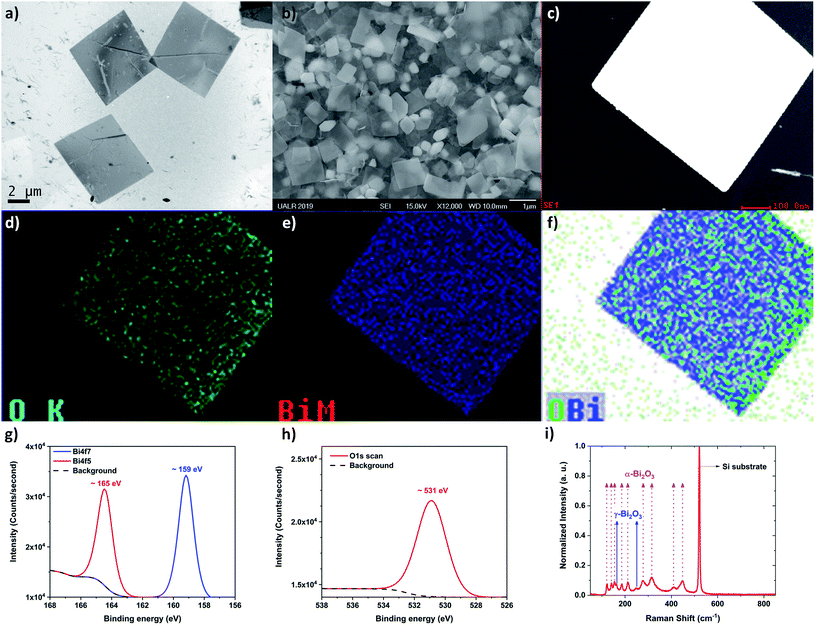 |
| Fig. 3 Bismuth oxide flakes obtained at high fluence (∼160 J cm−2) (a) Low-magnification TEM image acquired by using the JEOL JEM2100F at 80 kV. (b) Low magnification SEM image acquired by using the JEOL JSM7000F at 15 kV. (c) Typical STEM image of a flake, (d) oxygen chemical mapping on the flake, (e) bismuth mapping on the flake, and (f) chemical mapping displaying the bismuth and oxygen mapping together. The TEM used to acquire those images was the JEOL JEM2100F at 80 kV, (g) XPS spectra display the bismuth peaks Bi4f7 and Bi4f5 scan, (h) XPS spectra displaying the oxygen peak, (i) Raman spectra confirming that two crystalline phases of bismuth oxide (α and γ, see texts for details) are produced during the PLAL synthesis. | |
Raman spectroscopy was performed and confirmed the presence of α- and γ-phases of Bi2O3 (Fig. 3i). Fig. 3i displays the Raman spectra from several Bi2O3 nano-flakes at room temperature. These nano-flakes exhibited several distinguishable Raman peaks which were identified as α-Bi2O3 (123, 141, 154, 186, 213, 278, 315, 411 and 450 cm−1) and of γ-Bi2O3 (163 and 249 cm−1). From the Raman spectra, α-Bi2O3 peaks dominate as reported previously.58,59 Due to the limitation of the long-pass filter, only the Raman peaks showing up wavenumbers higher than 100 cm−1 could be detected. The 123 cm−1 mode is coming from Ag symmetry caused by mainly the Bi atoms' participation. Modes of 141 (Ag) and 154 cm−1 (Bg) may come from the displacements of both Bi and O atoms in the α-Bi2O3 lattice. The Raman peaks of the higher frequency modes 186, 213, 278, 315, 411, 450 cm−1 are attributed to the displacements of the O atoms in α-Bi2O3.60
The chemical composition of the surface of the flakes performed by XPS confirmed the formation of Bi2O3, and a mixture of α- and γ-phases were indicated by Raman spectroscopy; however, further studies were necessary in order to determine the crystallinity of the flakes. Indeed, Bi2O3 has six different allotropes and they each have their own distinct physico-chemical properties (Table 2). To determine the phase of bismuth oxide synthesized by PLAL, SAED was performed (Fig. 4). From Fig. 4b, the indexed SAED pattern corresponds to the α-Bi2O3 phase. Moreover, γ-Bi2O3 phase commensurate structures have also been found (Fig. 4c and d).61,62 The crystalline phases experimentally determined by electron diffraction is performed by finding the zone axis, and subsequently by measuring the reflections of the electron diffraction pattern, and by comparing the distances of the reciprocal lattice of the possible phases. However, despite the consideration of forbidden reflections produced by dynamical interaction, when some reflections (typically weak spots) do not correspond with the expected Bragg diffraction spots and when a simple fraction of the periodic arrangement is identified, i.e. (1/2,0,0); then, the atomic distribution can present commensurability. In the analysis performed by electron diffraction, commensurate structures of the γ-phase is measured and shown in Fig. 4c and d. Commensurability has already been observed in Bi2O3 by Zhou et al.62Fig. 4e shows a high resolution TEM (HRTEM) image in which the fast Fourier transform present a commensurate pattern as can be observed in the modulation contrast of the dark field image in Fig. 4f.
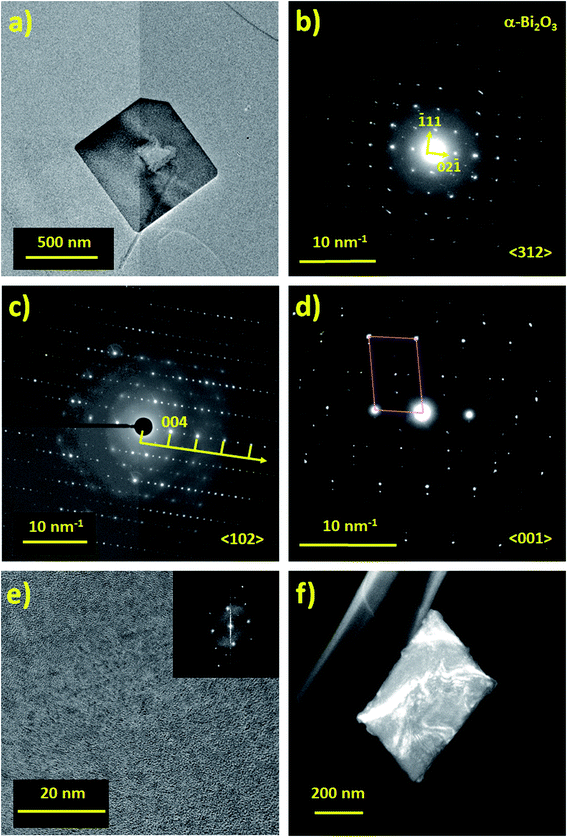 |
| Fig. 4 Bismuth oxide flake obtained at high fluence (∼160 J cm−2) (a) TEM image of an α-Bi2O3 nano-flake acquired by using the JEOL ARM200F at 200 kV and (b) its SAED pattern taken near the 〈312〉 zone axis. (c) and (d) commensurate structures of the γ-Bi2O3 nano-flake recorded in two different zone axes, (e) HRTEM image of the commensurate γ-Bi2O3 structure and (f) dark field image modulation contrast image. | |
For thickness determination of the flakes by electron holography, a field of view of 4 microns at 42 V of the bi-prism excitation was used with a fringe contrast of about 25%. Fig. 5a and b show the hologram and the reconstructed phase, respectively. Reconstructed phase of the hologram indicates a phase shift (Δφ) related to the thickness (τ), which is calculated using Δφ = CE|V0|τ, where V0 represents the mean inner potential for α-Bi2O3 (9.35 V), calculated using the atomic scattering amplitudes from ref. 63, and the parameter CE is 0.00729 rad V−1 nm−1 for the 200 kV accelerating voltage. Thickness measured by electron holography, after carbon background subtraction (∼20 nm of a lacey carbon commercial grid) was ∼68 nm as shown in Fig. 5c. Since our average thickness is below 100 nm as confirmed by electron holography, those structures are nano-flakes.
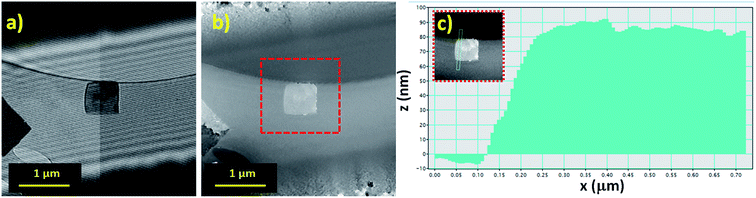 |
| Fig. 5 Thickness determination by off-axis electron holography performed with the JEOL ARM200F at 200 kV: (a) electron hologram, (b) reconstructed phase and (c) thickness intensity profile after carbon background subtraction ∼68 nm. | |
To be complete, AFM measurements were performed to determine more precisely the size distribution of the flakes; see Fig. 6a and b. The additional analysis shows that the heights of the flakes produced ranged from ∼20 nm to ∼70 nm, which supports the initial analysis; see Fig. 6c. It is also found that the length and widths of the flakes range from 0.6–1.7 μm and 0.5–1.4 μm, respectively; see Fig. 6d. For surface area and volume determination (Fig. 6e and f), it is assumed that the flakes adopt a rectangular prism morphology which seems to be a good approximation. This allows us to see that the flakes have high surface area and very high volume which results in a fairly low surface to volume ratio. Furthermore, descriptive statistics on the samples were determined and are tabulated in Table 3.
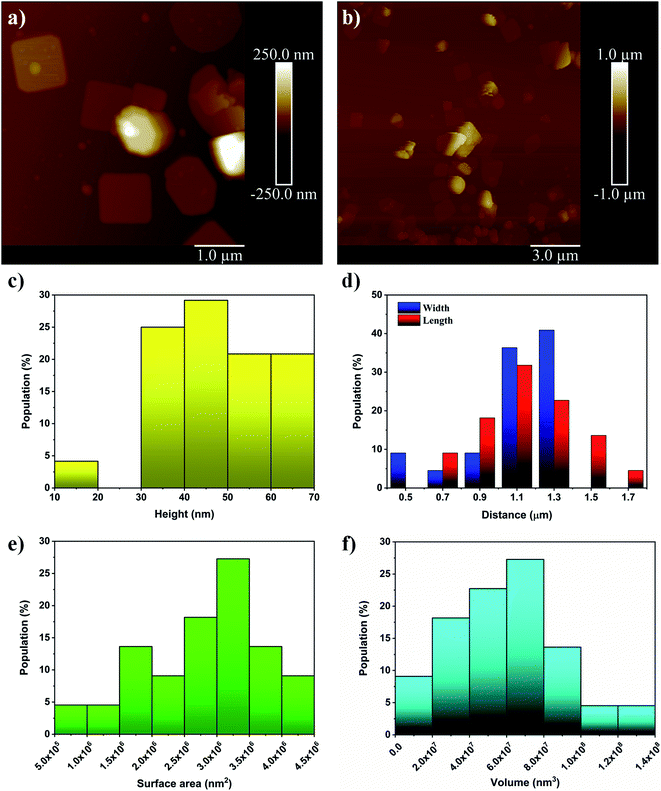 |
| Fig. 6 (a) and (b) are AFM images with scale bars of 1 μm and 3 μm, respectively. (c) and (d) show the distribution of the heights, lengths and widths of the nanoflakes, respectively. (e) and (f) show the surface area and volume distributions, respectively, which are calculated by approximating the morphology of the flakes as rectangular prisms. | |
Table 3 Descriptive statistics for the height, length, width, surface area, volume, and surface to volume ratio of Bi2O3 NFs (n = 22) as determined by AFM analysis. Values are reported as their average ± standard deviation
Height (nm) |
Length (μm) |
Width (μm) |
Surface area (nm2) |
Volume (nm3) |
Surface to volume ratio (nm−1) |
46 ± 13 |
1.15 ± 0.22 |
1.09 ± 0.25 |
(2.8 ± 0.9) × 106 |
(6.1 ± 2.9) × 107 |
0.05 ± 0.02 |
3.3 Antimicrobial assays
Colony counting assays are performed using one Gram-positive, MRSA, and one Gram-negative, MDR-EC. The results presented in Fig. 7 highlight the different responses between each type of bacteria and the Bi2O3 NFs. By comparison, the Bi2O3 NFs showed the strongest antibacterial effect on the Gram-negative bacteria.
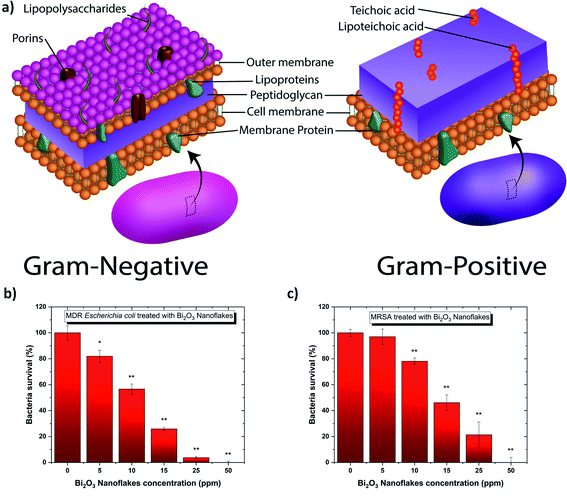 |
| Fig. 7 (a) Artistic representation showing the internal structure of Gram-negative and Gram-positive bacteria. Colony counting assay of (b) MDR-EC and (c) MRSA for 8 h in the presence of different concentrations of Bi2O3 NFs. All values represent the mean ± standard deviation. *p < 0.05, **p < 0.01 (compared to controls). | |
Then, the minimum inhibitory concentration (MIC) was estimated between 0–5 ppm and 5–10 ppm Bi2O3 NF's for the Gram-negative and -positive bacteria, respectively. This demonstrates that the Bi2O3 NF's are more effective at inhibiting the growth of Gram-negative bacteria. These reported MIC values are significantly lower than those reported in literature by Campos et. al. who reported a MIC value of 267 ppm when exposing bismuth oxide NPs to Escherichia coli and Staphylococcus aureus.64 The small MIC values obtained in our study can be explained by the “naked” surface of the nanoflakes produced by PLAL. Indeed, the surface of the nanoflakes produced by PLAL is not covered by any surfactants or left-over from chemical reactions, subsequently directly exposing the heavy metal bismuth to the bacteria's membrane. Moreover, the flake morphology (Table 3) also helped to wrap the bacteria; consequently affecting its permeability by modifying the transport through the membrane. Indeed, the spherical cells of S. aureus are up to 0.5 μm in diameter while the cells of E. coli are cylindrical with 1.0–2.0 μm long and about 0.5 μm in diameter.
A SEM investigation has been performed in order to visualize the interaction between each bacteria and the Bi2O3 NFs (Fig. 8). SEM images of control MDR-EC and MRSA (Fig. 8a and c) and bacteria after treatment with a concentration of 25 ppm of Bi2O3 NFs (Fig. 8b and d) are shown. The characterization indicates that the treatment with the nanostructures induced changes on both bacterial strains. Disruption of the outer cell membrane and cell lysis was seen after the treatment with the NFs. Therefore, evident cell damage was observed, with an abundant presence of holes and cracks all over the cell membrane, and bacterial deformation and collapse. The cell membrane damage is commonly found to be a consequence of an increased ROS production. From the SEM images of the bacteria, first, there is attachment of the NFs to the bacteria; second, the adhesion is responsible of the bacteria's membrane damage occurring by disrupting the transport of nutrients through the membrane.
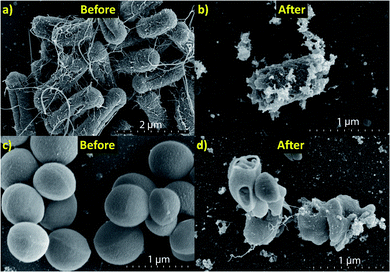 |
| Fig. 8 SEM images of the bacteria before and after interacting with the Bi2O3 NFs. (a) MDR-EC before interaction (control). (b) MDR-EC after interaction. (c) MRSA before interaction (control). (d) MRSA after interaction. | |
Finally, these experimental results are in agreement with the fact that Bi2O3 NFs can inhibit the growth of drug resistant pathogens,65 and this article successfully reports for the first time, the inhibition of the antibiotic resistant pathogens such as MDR-EC and MRSA.
3.4 Cytotoxicity
To determine the cytotoxicity of the Bi2O3 NFs on mammalian cells, in vitro MTS assays were performed with Human Dermal Fibroblasts (HDF) cells using different nano-flake concentrations, ranging from 10 and 50 ppm for between 24 and 72 hours (Fig. 9). As shown in Fig. 9, no significant cytotoxicity towards HDF cells was found for the whole range of concentrations at 24 h, showing no statistical differences compared to the control. Furthermore, a 72 h treatment led to the same cytotoxicity trend, except for the highest concentration, were there was a slight deviation compared to the control, indicating that such treatment conditions might not be the best option for a 72 h cell exposure.
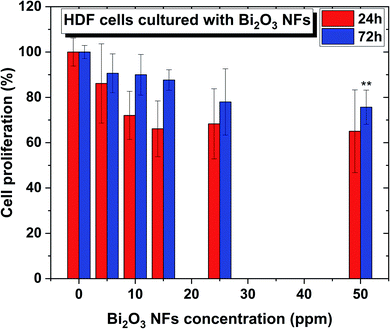 |
| Fig. 9 Human Dermal Fibroblasts (HDF) cells in the presence of Bi2O3 NFs at concentrations ranging from 0 to 50 ppm. n = 3. All values represent the mean ± standard deviation. *p < 0.05, **p < 0.01 (compared to controls). | |
4 Discussion
4.1 Nano-flake formation mechanism
Now that the 2D structure of the nanostructures has been established, it is important to understand their growth process. Laser exfoliation of the target was considered as a possible growth mechanism, but due to the chemical nature of the flake, Bi2O3, the formation of bismuth oxide cannot be explained by exfoliation (i.e. exfoliation of a pure bismuth target should result in pure bismuth nano-flakes, not Bi2O3 nano-flakes).66 Therefore, another growth mechanism was considered.67 PLAL is a straightforward physical technique to produce nanostructures. The laser beam interacts with the pure bismuth target while it is submerged in DI water. The target and the solvent get ionized and it consequently creates a plasma made of electrons, bismuth ions, hydrogen ions, and oxygen ions. Then, when the beam is off, the plasma cools down and releases the heat to the surrounding solvent creating a cavitation bubble. Within this cavitation bubble, the ions start reacting together forming bismuth oxide compounds. Bismuth oxide adopts a 2D growth in order to minimize the energy of its overall structure by privileging the growth of low surface energy facets.68 In this case, there are two competing factors, one geometric that minimizes the surface to volume ratio and one energetic that minimizes the surface energy. When the surface energy of the compound is below the surface energy of the solvent then the energetic factor wins and it allows the structure to grow like a 2D material, and when the surface energy of the compound is above that of the solvent, the structure adopts a spherical morphology in order to minimize the surface to volume ratio. The surface energy of Bi2O3 was recently measured by the Zisman method (i.e. the surface energy of the solid is the surface energy at which a liquid just completely wets the solid) to be 31.95 × 10−3 J m−2 which is obviously much less than the surface energy of water, 72.80 × 10−3 J m−2.69,70
4.2 Cost analysis
To bring this nanostructure to market, cost can ultimately be the breaking point for its use by the general public. In terms of nanoparticle treatments, the materials used are often expensive (gold and silver) and here a discussion surrounding the cost of treatment versus the MIC is provided. Fig. 10a and b shows the cost of the nanoparticle treatment for Gram-positive and Gram-negative bacteria, respectively, for various material systems versus its MIC value. No distinction was made between shapes or sizes of the NPs. From this figure, it is evident that gold and silver nanoparticle treatments are the most expensive ones whatever the MIC value considered. Cheaper alternatives based on metal oxides like TiO2, ZnO and Bi2O3 exist. Our Bi2O3 NF has been included in this analysis and was found to be very cost effective. This demonstrates that the metal oxide nanostructures produced in this work are a good alternative to noble metal nanoparticles for antibacterial applications.
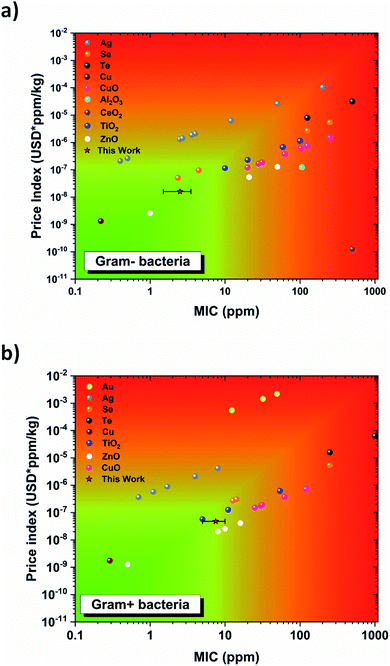 |
| Fig. 10 Cost analysis for various antibacterial materials currently used as nanoparticle treatments. The price index is the price of the material per kilogram multiplied by the MIC i.e., the cost of the treatment. Data were collected from ref. 21, 22, 71–79. (a) It shows the antibacterial cost analysis for Gram-negative bacteria. (b) It shows the antibacterial cost analysis for Gram-positive bacteria. | |
5 Conclusions
Bi2O3 nano-flakes have been successfully synthesized by PLAL at high fluence (∼160 J cm−2) without any external assistance like acoustic, electric or magnetic fields. The nano-flakes exhibited two different phases: α- and γ- (commensurate structure). The physical dimensions of the flakes were around 1 μm along the x- and y-axis while only 47.0 ± 12.7 nm thick along the z-axis. The strongest advantage of using PLAL as a synthesis methodology is the cleanliness of the Bi2O3's surface. More work is required to finely tune the irradiation time, the fluence and the repetition rate in order to control more precisely the crystalline structure, and thickness of the Bi2O3 nano-flakes. The nano-flakes can also be used as cost-effective antibacterial agents against antibiotic resistant Gram-positive and -negative bacteria, as shown in this report against MSRA and MDR-EC. More work is required in order to fully understand the mechanism of action of the flake against the bacteria's membrane.
Conflicts of interest
There are no conflicts of interests to declare.
Acknowledgements
L. D. G., M. K., S. E. and G. G. would like to thank the Center for Integrative Nanotechnology Sciences (CINS) of UA Little Rock for the use of their UV-visible spectrometer, AFM, SEM and TEM. T. B. H. acknowledges the financial support from the National Science Foundation (NSF) (Grant #DMR-1709612). P. P. and A. P. thanks the Kleberg Advanced Microscopy Center at UTSA and DoD financing support #W911NF-18-1-0439.
References
-
ACSIHC Landmarks, The discovery and development of penicillin, ACS & RSC, 1999, available from http://www.acs.org/content/acs/en/education/whatischemistry/landmarks/flemingpenicillin.html Search PubMed.
- R. I. Aminov, A Brief History of the Antibiotic Era: Lessons Learned and Challenges for the Future, Front. Microbiol., 2010, 1, 134 Search PubMed.
-
WH Organization, available from https://www.who.int/antimicrobial-resistance/en/.
- D. Berry, C. Xi and L. Raskin, Microbial ecology of drinking water distribution systems, Curr. Opin. Biotechnol., 2006, 17(3), 297–302 Search PubMed.
- J. P. Cabral, Water microbiology. Bacterial pathogens and water, Int. J. Environ. Res. Public Health, 2010, 7(10), 3657–3703 Search PubMed.
- J. M. Schierholz and J. Beuth, Implant infections: a haven for opportunistic bacteria, J. Hosp. Infect., 2001, 49(2), 87–93 Search PubMed.
- L. D. Geoffrion and G. Guisbiers, Quantum confinement: size on the grill!, J. Phys. Chem. Solids, 2020, 140, 109320 Search PubMed.
- G. Guisbiers, Advances in thermodynamic modelling of nanoparticles, Adv. Phys.: X, 2019, 4(1), 1668299 Search PubMed.
- N. Elahi, M. Kamali and M. H. Baghersad, Recent biomedical applications of gold nanoparticles: a review, Talanta, 2018, 184, 537–556 Search PubMed.
- C. Marambio-Jones and E. M. V. Hoek, A review of the antibacterial effects of silver nanomaterials and potential implications for human health and the environment, J. Nanopart. Res., 2010, 12(5), 1531–1551 Search PubMed.
- S. Kayal and R. V. Ramanujan, Anti-cancer drug loaded iron-gold core-shell nanoparticles (Fe@Au) for magnetic drug targeting, J. Nanosci. Nanotechnol., 2010, 10(9), 5527–5539 Search PubMed.
- J. Yin, S. Shan, L. Yang, D. Mott, O. Malis and V. Petkov,
et al., Gold–Copper Nanoparticles: Nanostructural Evolution and Bifunctional Catalytic Sites, Chem. Mater., 2012, 24(24), 4662–4674 Search PubMed.
- A. Magrez, S. Kasas, V. Salicio, N. Pasquier, J. W. Seo and M. Celio,
et al., Cellular toxicity of carbon-based nanomaterials, Nano Lett., 2006, 6(6), 1121–1125 Search PubMed.
- S. Dadashi, R. Poursalehi and H. H. Delavari, Formation, gradual oxidation mechanism and tunable optical properties of Bi/Bi2O3 nanoparticles prepared by Nd:YAG laser ablation in liquid: Dissolved oxygen as genesis of tractable oxidation, Mater. Res. Bull., 2018, 97, 421–427 Search PubMed.
- L. Escobar-Alarcon, E. V. Granados, D. A. Solis-Casados, O. Olea-Mejia, M. Espinosa-Pesqueira and E. Haro-Poniatowski, Preparation of bismuth-based nanosheets by ultrasound-assisted liquid laser ablation, Appl. Phys. A: Mater. Sci. Process., 2016, 122(4), 1–7 Search PubMed.
- M. A. Gondal, T. A. Saleh and Q. Drmosh, Optical Properties of Bismuth Oxide Nanoparticles Synthesized by Pulsed Laser Ablation in Liquids, Sci. Adv. Mater., 2012, 4(3–4), 507–510 Search PubMed.
- R. A. Ismail and F. A. Fadhil, Effect of electric field on the properties of bismuth oxide nanoparticles prepared by laser ablation in water, J. Mater. Sci.: Mater. Electron., 2014, 25(3), 1435–1440 Search PubMed.
- S. Jain, D. G. Hirst and J. M. O'Sullivan, Gold nanoparticles as novel agents for cancer therapy, Br. J. Radiol., 2012, 85(1010), 101–113 Search PubMed.
- B. Le Ouay and F. Stellacci, Antibacterial activity of silver nanoparticles: a surface science insight, Nano Today, 2015, 10(3), 339–354 Search PubMed.
- Available from https://en.wikipedia.org/wiki/Prices_of_chemical_elements#cite_note-fn-transient-46.
- L. D. Geoffrion, T. Hesabizadeh, D. Medina-Cruz, M. Kusper, P. Taylor and A. Vernet-Crua,
et al., Naked selenium nanoparticles for antibacterial and anticancer treatment, ACS Omega, 2020, 5, 2660–2669 Search PubMed.
- B. Zare, M. A. Faramarzi, Z. Sepehrizadeh, M. Shakibaie, S. Rezaie and A. R. Shahverdi, Biosynthesis and recovery of rod-shaped tellurium nanoparticles and their bactericidal activities, Mater. Res. Bull., 2012, 47(11), 3719–3725 Search PubMed.
- S. Dadashi, R. Poursalehi and H. Delavari, Optical and structural properties of oxidation resistant colloidal bismuth/gold nanocomposite: an efficient nanoparticles based contrast agent for X-ray computed tomography, J. Mol. Liq., 2018, 254, 12–19 Search PubMed.
- S. Dadashi, R. Poursalehi and H. Delavari, Optical and structural properties of Bi-based nanoparticles prepared via pulsed Nd:YAG laser ablation in organic liquids, Appl. Phys. A: Mater. Sci. Process., 2018, 124(6), 406 Search PubMed.
- X. Yu, A. Li, C. Zhao, K. Yang, X. Chen and W. Li, Ultrasmall Semimetal Nanoparticles of Bismuth for Dual-Modal Computed Tomography/Photoacoustic Imaging and Synergistic Thermoradiotherapy, ACS Nano, 2017, 11(4), 3990–4001 Search PubMed.
- G. Bandoli, D. Barreca, E. Brescacin, G. A. Rizzi and E. Tondello, Pure and mixed phase Bi2O3 thin films obtained by metal organic chemical vapor deposition, Chem. Vap. Deposition, 1996, 2(6), 238–242 Search PubMed.
- C. Y. Pan, Y. Yan, H. D. Li and S. Hu, Synthesis of Bismuth Oxide Nanoparticles by a Templating Method and its Photocatalytic Performance, Adv. Mater. Res., 2012, 557–559, 615–618 Search PubMed.
- F. Farzaneh, L. Jafari Foruzin, Z. Sharif and E. Rashtizadeh, Green Synthesis and Characterization of Bi2O3 Nanorods as Catalyst for Aromatization of 1,4-Dihydropyridines, J. Sci., Islamic Repub. Iran, 2017, 28, 113–118 Search PubMed.
- Y. Lu, Y. Zhao, J. Zhao, Y. Song, Z. Huang and F. Gao,
et al., Induced Aqueous Synthesis of Metastable β-Bi2O3 Microcrystals for Visible-Light Photocatalyst Study, Cryst. Growth Des., 2015, 15(3), 1031–1042 Search PubMed.
- H. Oudghiri-Hassani, S. Rakass, F. T. Al Wadaani, K. J. Al-ghamdi, A. Omer and M. Messali,
et al., Synthesis, characterization and photocatalytic activity of α-Bi2O3 nanoparticles, Journal of Taibah University for Science, 2018, 9(4), 508–512 Search PubMed.
- M. Schlesinger, M. Weber, S. Schulze, M. Hietschold and M. Mehring, Metastable beta-Bi2O3 Nanoparticles with Potential for Photocatalytic Water Purification Using Visible Light Irradiation, ChemistryOpen, 2013, 2(4), 146–155 Search PubMed.
- A. Hameed, T. Montini, V. Gombac and P. Fornasiero, Surface phases and photocatalytic activity correlation of Bi2O3/Bi2O4−x nanocomposite, J. Am. Chem. Soc., 2008, 130(30), 9658–9659 Search PubMed.
- C. Stewart, K. Konstantinov, S. McKinnon, S. Guatelli, M. Lerch and A. Rosenfeld,
et al., First proof of bismuth oxide nanoparticles as efficient radiosensitisers on highly radioresistant cancer cells, Phys. Med., 2016, 32(11), 1444–1452 Search PubMed.
- K. Bogusz, M. Tehei, C. Stewart, M. McDonald, D. Cardillo and M. Lerch,
et al., Synthesis of potential theranostic system consisting of methotrexate-immobilized (3-aminopropyl)trimethoxysilane coated α-Bi2O3 nanoparticles for cancer treatment, RSC Adv., 2014, 4(46), 24412–24419 Search PubMed.
- Y. Shimizu, E. Kanazawa, Y. Takao and M. Egashira, H2 Sensing Properties of Metal Oxide Semiconductors as Varistor-Type Gas Sensors, IEEJ Transactions on Sensors and Micromachines, 1997, 117(11), 560–564 Search PubMed.
- M. R. Ambika, N. Nagaiah and S. K. Suman, Role of bismuth oxide as a reinforcer on gamma shielding ability of unsaturated polyester based polymer composites, J. Appl. Polym. Sci., 2017, 134(13), 44657 Search PubMed.
- C. Stewart, K. Konstantinov, M. McDonald, K. Bogusz, D. Cardillo and S. Oktaria,
et al., Engineering of Bismuth Oxide Nanoparticles to Induce Differential Biochemical Activity in Malignant
and Nonmalignant Cells, Part. Part. Syst. Charact., 2014, 31(9), 960–964 Search PubMed.
- A. J. Salazar-Pérez, M. A. Camacho-López, R. A. Morales-Luckie, V. Sánchez-Mendieta, F. Ureña-Núñez and J. Arenas-Alatorre, Structural evolution of Bi2O3 prepared by thermal oxidation of bismuth nano-particles, Sociedad Mexicana de Ciencia y Tecnología de Superficies y Materiales, 2005, 18, 4–8 Search PubMed.
- F. I. Lopez-Salinas, G. A. Martinez-Castanon, J. R. Martinez-Mendoza and F. Ruiz, Synthesis and characterization of nanostructured powders of Bi2O3, BiOCl and Bi, Mater. Lett., 2010, 64(14), 1555–1558 Search PubMed.
- H. Kodama, A. Watanabe and Y. Yajima, Synthesis of a new bismuth oxide fluoride with the γ-Bi2O3 structure type, J. Solid State Chem., 1987, 67(1), 170–175 Search PubMed.
- D. S. Aidhy, J. C. Nino, S. B. Sinnott, E. D. Wachsman and S. R. Phillpot, Vacancy-Ordered Structure of Cubic Bismuth Oxide from Simulation and Crystallographic Analysis, J. Am. Ceram. Soc., 2008, 91(7), 2349–2356 Search PubMed.
- N. Cornei, N. Tancret, F. Abraham and O. Mentre, New epsilon-Bi2O3 metastable polymorph, Inorg. Chem., 2006, 45(13), 4886–4888 Search PubMed.
- A. F. Gualtieri, S. Immovilli and M. Prudenziati, Powder X-ray diffraction data for the new polymorphic compound ω-Bi2O3, Powder Diffr., 2013, 12(02), 90–92 Search PubMed.
- H. T. Fan, X. M. Teng, S. S. Pan, C. Ye, G. H. Li and L. D. Zhang, Optical properties of δ-Bi2O3 thin films grown by reactive sputtering, Appl. Phys. Lett., 2005, 87(23), 231916 Search PubMed.
- Y. Qiu, D. Liu, J. Yang and S. Yang, Controlled Synthesis of Bismuth Oxide Nanowires by an Oxidative Metal Vapor Transport Deposition Technique, Adv. Mater., 2006, 18(19), 2604–2608 Search PubMed.
- M. Anilkumar, R. Pasricha and V. Ravi, Synthesis of bismuth oxide nanoparticles by citrate gel method, Ceram. Int., 2005, 31(6), 889–891 Search PubMed.
- C. Y. Pan, X. H. Li, F. Wang and L. F. Wang, Synthesis of bismuth oxide nanoparticles by the polyacrylamide gel route, Ceram. Int., 2008, 34(2), 439–441 Search PubMed.
- R. K. Jha, R. Pasricha and V. Ravi, Synthesis of bismuth oxide nanoparticles using bismuth nitrate and urea, Ceram. Int., 2005, 31(3), 495–497 Search PubMed.
- B. Gökce, V. Amendola and S. Barcikowski, Opportunities and Challenges for Laser Synthesis of Colloids, ChemPhysChem, 2017, 18, 983–985 Search PubMed.
- D. Zhang, B. Gokce and S. Barcikowski, Laser Synthesis and Processing of Colloids: Fundamentals and Applications, Chem. Rev., 2017, 117(5), 3990–4103 Search PubMed.
- M. Flores-Castaneda, E. Camps, M. Camacho-Lopez, S. Muhl, E. Garcia and M. Figueroa, Bismuth nanoparticles synthesized by laser ablation in lubricant oils for tribological tests, J. Alloys Compd., 2015, 643, S67–S70 Search PubMed.
- R. G. T. Rosa, C. D. Duarte, W. H. Schreiner, N. P. Mattoso, A. G. Bezerra and A. Barison,
et al., Structural, morphological and optical properties of Bi NPs obtained by laser ablation and their selective detection of L-cysteine, Colloids Surf., A, 2014, 457, 368–373 Search PubMed.
- R. K. Verma, K. Kumar and S. B. Rai, Near infrared induced optical heating in laser ablated Bi quantum dots, J. Colloid Interface Sci., 2013, 390, 11–16 Search PubMed.
- D. Zhang, B. Gökce and S. Barcikowski, Laser Synthesis and Processing of Colloids: Fundamentals and Applications, Chem. Rev., 2017, 117, 3990–4103 Search PubMed.
- M. Kusper and G. Guisbiers, Synthesis of aluminum oxide nanoparticles by laser ablation in liquids, MRS Adv., 2018, 3(64), 3899–3903 Search PubMed.
- O. Van Overschelde and G. Guisbiers, Photo-fragmentation of selenium powder by Excimer laser ablation in liquids, Opt. Laser Technol., 2015, 73, 156–161 Search PubMed.
- Q. Zhang, Y. Zhou, F. Wang, F. Dong, W. Li and H. M. Li,
et al., From semiconductors to semimetals: bismuth as a photocatalyst for NO oxidation in air, J. Mater. Chem. A, 2014, 2(29), 11065–11072 Search PubMed.
- C.-H. Ho, C.-H. Chan, Y.-S. Huang, L.-C. Tien and L.-C. Chao, The study of optical band edge property of bismuth oxide nanowires α-Bi2O3, Opt. Express, 2013, 21, 11965–11972 Search PubMed.
- K. Balasubramanian, R. Udayabhaskar and A. Kishore, Optical and phonon properties of Sm-doped α-BiO micro rods, Appl. Phys. A: Mater. Sci. Process., 2014, 117, 1409–1414 Search PubMed.
- C. H. Ho, C. H. Chan, Y. S. Huang, L. C. Tien and L. C. Chao, The study of optical band edge property of bismuth oxide nanowires alpha-Bi2O3, Opt. Express, 2013, 21(10), 11965–11972 Search PubMed.
- Á. R. Landa-Cánovas, E. Vila, J. Hernández-Velasco, J. Galy and A. Castro, Transmission Electron Microscopy Study of Low Mo-content Bi–Mo–O Phases, Microsc. Microanal., 2012, 18, 71–72 Search PubMed.
- W. Zhou, D. A. Jefferson, M. Alario-Franco and J. M. Thomas, Superlattices in ternary oxides derived from bismuth oxide (Bi2O3): new families of ordered phases based on the fluorite structure, J. Phys. Chem., 1987, 91, 512–514 Search PubMed.
- P. A. Doyle and P. S. Turner, Relativistic Hartree–Fock X-ray and electron scattering factors, Acta Crystallogr., Sect. A: Cryst. Phys., Diffr., Theor. Gen. Crystallogr., 1968, A24, 390–397 Search PubMed.
- V. Campos, A. Almaguer-Flores, D. Velasco-Aria, D. Diaz and S. E. Rodil, Bismuth and Silver Nanoparticles as Antimicrobial Agent over Subgingival Bacterial and Nosocomial Strains, J. Mater. Sci. Eng. A, 2018, 8, 142–146 Search PubMed.
- Y. Luo, M. Hossain, C. Wang, Y. Qiao, J. An and L. Ma,
et al., Targeted nanoparticles for enhanced X-ray radiation killing of multidrug-resistant bacteria, Nanoscale, 2013, 5, 687–694 Search PubMed.
- H. Ma, Z. Shen and S. Ben, Understanding the exfoliation and dispersion of MoS2 nanosheets in pure water, J. Colloid Interface Sci., 2018, 517, 204–212 Search PubMed.
- A. M. Müller, Optical control of layered nanomaterial generation by pulsed-laser ablation in liquids, J. Mod. Opt., 2020, 67, 49–54 Search PubMed.
-
G. Guisbiers and M. José-Yacaman, Use of Chemical Functionalities to Control Stability of Nanoparticles, Reference Module in Chemistry, Molecular Sciences and Chemical Engineering, 2018, Encyclopedia of Interfacial Chemistry, pp. 875–885 Search PubMed.
- F. M. Tezel and İ. Afşin Kariper, Synthesis, surface tension, optical and dielectric properties of bismuth oxide thin film, Mater. Sci.-Pol., 2017, 35(1), 87–93 Search PubMed.
- G. Guenther, R. Theissmann and O. Guillon, Size-Dependent Phase Transformations in Bismuth Oxide Nanoparticles. II. Melting and Stability Diagram, J. Phys. Chem. C, 2014, 118(46), 27020–27027 Search PubMed.
- Y. N. Slavin, J. Asnis, U. O. Hafeli and H. Bach, Metal nanoparticles: understanding the mechanisms behind antibacterial activity, J. Nanobiotechnol., 2017, 15(1), 65 Search PubMed.
- S. A. Moreno-Álvarez, G. A. Martínez-Castañón, N. Niño-Martínez, J. F. Reyes-Macías, N. Patiño-Marín and J. P. Loyola-Rodríguez,
et al., Preparation and bactericide activity of gallic acid stabilized gold nanoparticles, J. Nanopart. Res., 2010, 12(8), 2741–2746 Search PubMed.
- M. Valodkar, S. Modi, A. Pal and S. Thakore, Synthesis and anti-bacterial activity of Cu, Ag and Cu–Ag alloy nanoparticles: a green approach, Mater. Res. Bull., 2011, 46(3), 384–389 Search PubMed.
- M. Ahamed, H. A. Alhadlaq, M. A. M. Khan, P. Karuppiah and N. A. Al-Dhabi, Synthesis, Characterization, and Antimicrobial Activity of Copper Oxide Nanoparticles, J. Nanomater., 2014, 2014, 1–4 Search PubMed.
- A. Azam, A. S. Ahmed, M. Oves, M. S. Khan and A. Memic, Size-dependent antimicrobial properties of CuO nanoparticles against Gram-positive and -negative bacterial strains, Int. J. Nanomed., 2012, 7, 3527–3535 Search PubMed.
- V. V. Thekkae Padil and M. Cernik, Green synthesis of copper oxide nanoparticles using gum karaya as a biotemplate and their antibacterial application, Int. J. Nanomed., 2013, 8, 889–898 Search PubMed.
- S. Siddique, Z. H. Shah, S. Shahid and F. Yasmin, Preparation, characterization and antibacterial activity of ZnO nanoparticles on broad spectrum of microorganisms, Acta Chim. Slov., 2013, 60(3), 660–665 Search PubMed.
- E.-K. Zarrindokht, Antibacterial activity of ZnO nanoparticle on Gram-positive and Gram-negative bacteria, Afr. J. Microbiol. Res., 2012, 5(12), 1368–1373 Search PubMed.
- E. Zonaro, S. Lampis, R. J. Turner, S. J. Qazi and G. Vallini, Biogenic selenium and tellurium nanoparticles synthesized by environmental microbial isolates efficaciously inhibit bacterial planktonic cultures and biofilms, Front. Microbiol., 2015, 6, 584 Search PubMed.
|
This journal is © The Royal Society of Chemistry 2021 |
Click here to see how this site uses Cookies. View our privacy policy here.