DOI:
10.1039/D1MA00733E
(Review Article)
Mater. Adv., 2021,
2, 7820-7841
Surface engineering of biodegradable implants: emerging trends in bioactive ceramic coatings and mechanical treatments
Received
17th August 2021
, Accepted 31st October 2021
First published on 2nd November 2021
Abstract
Biodegradable polymers, metals, and ceramics have emerged as promising alternatives to prepare degradable implants in recent years, which are better alternatives to conventional non-degradable implants to treat a broad range of disorders. Surface engineering strategies are widely applied for enhancing their performance, including orthopedic, cardiovascular, and craniofacial devices, among many others. Bioactive ceramic coatings on degradable biomedical implants can enhance bio-integration and augment healing while tailoring the degradation rate of the device. Bioactive ceramics form a chemical bond with the surrounding tissue, thus helping in the integration process of the implanted polymer or metal device. Several coatings also impart antibacterial activity to minimize infections. Additionally, mechanical surface treatments have emerged as a promising route for surface engineering and have been predominantly used to modify the surface of degradable metallic implants. The mechanical treatment of the surface primarily enhances the bio-corrosion resistance of the implants without compromising biocompatibility. Surface mechanical treatments also improve the biomechanical performance of the implant through surface nanocrystallization. The present review gives an overview of bioactive ceramics commonly used as a coating material for degradable biomedical implants and the effect of bioactive ceramic coating on their biological, corrosion, and mechanical responses. This review also features state-of-the-art mechanical techniques utilized for surface modification of degradable implants. Gaps in the current technology that can be addressed in future research are identified to improve the clinical success of surface-modified degradable implants as they rapidly emerge as the next-generation devices.
1. Introduction
Biomedical implants are used clinically to replace, support, or enhance non-functional tissues and organs owing to disease, trauma, or congenital defects. In the last few decades, implants have increasingly been developed to assist in the repair and regeneration of tissues as an alternative to transplants, which are scarce and prone to eliciting an adverse immune response.1 Biomedical implants can be broadly categorized into two groups, non-degradable and degradable implants based on the biomaterials used. Non-degradable implants are typically used for permanent or long-term applications as replacements. In many applications, these implants will need a second surgery for removal after the completion of the desired healing response to minimize undesired effects and are prone to infections. In contrast, degradable implants are attractive for short-term applications to provide an appropriate response to the healing site. These implants are designed to degrade and would not need a second surgery. The degradable implant can be fabricated using various degradable materials, including polymers, metals and their alloys, and ceramics.2 Degradable polymers include naturally sourced polymers such as chitosan,3,4 collagen,5 and silk fibroin,6 and several synthetic polymers, including polyesters (such as poly(lactic acid) (PLA),7–9 polycaprolactone (PCL)10,11), polyanhydrides,12 and polyorthoesters,13 among others. Metals such as Mg,14,15 Zn,16,17 and Fe18 and their alloys are the most widely studied degradable metallic biomaterials. Bioactive glasses19 and calcium phosphates20 are among the most commonly studied bioresorbable ceramics. Many comprehensive reviews are available in the literature on each of these classes of degradable materials.21–23
Bioceramics are often classified into bio-inert, bioactive, and resorbable categories based on the biological response to the implanted ceramics. After implantation, a thin fibrous tissue layer forms on the surface of a bio-inert ceramic to generate a minimum tissue response and poor bio-integration because of the separation between the surrounding tissue and the implant surface. Bioactive and resorbable ceramics tend to form chemical bonds with the host tissue that facilitate bio-integration. Resorbable materials are resorbed over time and are replaced by host tissues. Some of the key challenges associated with using bioinert implants other than fibrous capsule formation include bacterial infection, chronic inflammation, and constant physical discomfort.24 However, bioactive ceramics help in bio-integration with the surrounding tissue and they offer additional benefits by imparting antibacterial activity25 and augmenting angiogenesis.26 Bioactive ceramics are brittle and have poor strength and fatigue resistance. Thus, bioactive ceramics are not preferred for use alone as load-bearing implants. The application of bioactive ceramics as a coating material on implants can overcome this limitation and offer the desired combination of load-bearing properties of the substrate and the bioactivity of the coating. Aside from bioactivity, coatings can help in modulating the degradation rate of rapidly degrading materials and enhance their surface hardness.
Besides coatings, mechanical surface treatments of the near-surface or bulk biomaterials are considered potentially cost-effective and efficient strategies to enhance the bio-corrosion performance. As these processes do not deal with any additional new materials except for the original base substrate material, there are no additional challenges associated with biocompatibility. A unique advantage of the mechanical treatments over the coating process is that it improves the biomechanical integrity of the material in terms of increased strength27 and fatigue resistance28,29 of implants. Different mechanical surface treatments such as machining, shot peening, ball burnishing, and laser peening have been studied predominantly on Mg-based alloys, with fewer reported attempts on other biodegradable alloys. Biodegradable polymers are generally too soft and are not subjected to such treatments.
Recognizing the benefits of coating and surface mechanical treatments on degradable materials, there have been several attempts in recent years to modify the surface of resorbable implants. In this review article, we first focus on the overview of the polymeric and metallic materials used to develop biodegradable implants. Next, the emerging trends and current state of the art in the field of bioactive ceramic-based coatings and surface mechanical treatments on degradable biomedical implants are summarized in the review. The first part of the article highlights the various categories of bioactive ceramics and their application in the coating of biomaterials. We also describe the deposition methodologies used to prepare the coatings. The second part presents various surface mechanical treatments to modify the corrosion and biomechanical properties of degradable Mg-based biomaterials. Finally, the gaps and opportunities for the future for this field are highlighted.
2. Clinical significance of biodegradable implant materials
In order to address the stress shielding and secondary surgical intervention associated with permanent metallic implants, biodegradable materials have attracted significant attention and are being widely considered for orthopedic implants and cardiovascular stenting.22,30,31 Gradual degradation with a suitable rate while providing mechanical and surface integrity is crucial to realize the functional benefit of biodegradable materials. Despite recognizing their potential benefits, there are still limited choices of biodegradable materials, and researchers are continuously exploring new types of materials or improving the existing ones to achieve the desired functionality. Biodegradable metals and polymers are widely used biomaterials as implants/devices. The following section briefly summarizes the state-of-the-art development of these two types of biodegradable materials in recent times.
2.1 Fe-based degradable alloys
Owing to their superior mechanical properties, such as high strength (up to 1450 MPa) and high ductility (up to 80% elongation), Fe-based biodegradable metal alloys have been studied as potential degradable implants such as stents in earlier times.32In vivo animal tests reported that while Fe-based alloys did not show any significant evidence of inflammatory response or systemic toxicity to the host immune cell, they exhibited two main limitations – (1) slow degradation (Fe stent remained intact and maintained its mechanical integrity in 6 to 18 months follow up post-surgery). Fe-based implants usually have a degradation rate of less than 1 mm/year, and the release of Fe ions takes place at ppm level in vitro,33,34 (2) ferromagnetism of Fe causing incompatibility with crucial medical devices such as MRI for diagnosis and assessment. It may limit full-body scanning at security checkpoints. These observations motivated researchers to explore new alternative approaches to increasing the degradation rate and minimizing the detrimental effect of ferromagnetism.
One alternative was to introduce different alloying elements such as manganese (Mn), palladium (Pd), tungsten (W), tin (Sn), boron (B), carbon (C), silicon (Si), cobalt (Co), etc., into Fe to accelerate the corrosion rate while maintaining mechanical integrity. For instance, the Fe30Mn6Si alloy showed a higher degradation rate than the Fe30Mn alloy while minimizing magnetic susceptibility.35 Other alloying elements, namely, Co, W, B, and C, were shown to improve the yield and ultimate strength of Fe, but Sn caused a reduction in overall mechanical properties.36 Adding Mn and Pd elements together into Fe in an appropriate ratio also increased the corrosion rate. For example, the degradation rate of Fe–10Mn–1Pd accelerated ten times that of low carbon steel.37
In addition to the alloying elements, the choice of manufacturing route also affects the microstructural and surface characteristics of Fe-based alloys. For example, a highly porous Fe–Mn alloy produced by powder metallurgy, inkjet 3D printing, and electroforming showed faster in vitro degradation than the same alloy made by the traditional casting process.38–40 In this regard, the primary reason for the faster degradation is smaller grains with more grain boundaries and microstructure defects which are more susceptible to corrosive attack. Furthermore, the porous surface texture allows the corrosion medium to penetrate into the materials, thereby accelerating the degradation rate.
To achieve a faster degradation rate of pure Fe, a variety of secondary phases were added into the Fe matrix to generate micro-galvanic corrosion between the Fe matrix (anode) and secondary phases (cathode). W and carbon nanotubes (CNTs) are conductive and act as superior cathodes. Yet, the in vitro corrosion tests demonstrated a marginal enhancement of the degradation rate, other than an increase in strength, when secondary phases (W and CNTs) are added into the Fe alloy.41 Therefore, they might be a good choice for high load-bearing permanent implants instead of biodegradable ones.
Unfortunately, there are limited animal trials showing an unfavorable degradation rate of Fe-based biodegradable materials. For instance, Feng et al. deployed bio-corrodible nitrided iron stents in juvenile pig iliac arteries, and at three or six months post-operation, the stented vessels remained well patented.42 So, the results indicate that the degradation of Fe is minimal. In other words, Fe-based alloys do not completely degrade. Because of these, Fe-based alloys still face challenges for potential use in clinical applications, and perhaps more studies are needed to revolutionize the desired properties of Fe-based biodegradable materials.
2.2 Mg-based degradable alloys
Magnesium (Mg) is essential to human metabolism and is the fourth most abundant cation in the human body. In addition, Mg is a cofactor for many enzymes, which stabilizes the structure of DNA and RNA. Mg exhibits fast corrosion in the chloride-containing physiological environment. Thus, Mg has emerged as a biodegradable and biocompatible material for potential use as implants. Initially introduced by the Australian-German physician Erwin Payr, Mg has been used for recovering joint motion for bone fracture fixation with wires and pegs as intramedullary rods.43 Later, the use of Mg sheets was studied for restoring joint motion in animals and humans. However, the rapid generation of hydrogen/hydroxide anions due to the corrosion in Mg could pose serious problems for patients. For instance, such severe side effects of rapid generation of hydrogen may prevent the possible application of an Mg stent in the blood circulating vascular system.44 Therefore, the rapid degradation of pure Mg due to accelerated corrosion in a physiological environment is found to be the main concern and prevents the use of Mg as an implant in bone or joint repair procedures.
In order to overcome the challenge posed by pure Mg, researchers have explored the potential opportunity of improving the corrosion performance by alloying various appropriately selected elements with Mg. For instance, metals such as zinc (Zn), calcium (Ca), aluminum (Al), silver (Ag), yttrium (Y), zirconium (Zr), neodymium (Nd), and Mn have been used as alloying elements to enhance the mechanical properties and corrosion behavior. Mg–Ca, Mg–Ca–Zn, and Mg–Zn are a few examples of Mg-based alloys. Cha et al. implanted and observed a bone screw made of as-extruded biodegradable Mg–5.0Ca–1.0Zn alloy on the femoral condyle of a New Zealand white rabbit for 24 weeks.45 Histological and micro-computed tomographic analysis shows the new bone formation and modeling with reduced bubble formation and foreign body response around the progressively degraded implant sample.
Thus, with a suitable selection of alloying elements and their compositions, the microstructure of a Mg material can be designed in such a way that the mechanical properties become similar to those of cancellous bone, hence making it ideal for use as a bone substitute. Recently, Farraro et al.46 conducted case studies exploring the efficacy of Mg-based alloys in tissue engineering. Both in vitro and in vivo studies reveal an improved performance of the interference screw in terms of joint stability and graft function with gradual complete degradation of Mg alloys.
Li et al.47 developed pins made of Mg–Ca alloys by varying the Ca concentration and using different fabrication techniques, implanted them into left and right rabbit femoral shafts, and monitored the degradation process for 1, 2, and 3 months. Initial cytotoxicity tests showed that the Mg–1.0Ca alloy did not induce any toxicity in the cells. Periodic observation of implanted pins revealed that Mg–Ca alloy pins degraded gradually during the test period, as observed by the reduction of the pin diameter. Furthermore, new bone was found to be formed around the Mg–Ca alloy pins, indicating the superior mechanical integrity and osteogenesis of Mg–Ca alloy pins around the bones. A ternary alloy of magnesium with alloying elements such as Zn and cerium (Ce) was studied by Behera et al.48 The ternary alloy showed the best corrosion resistance potential compared to binary Mg–Zn and Mg–Ce alloys. Furthermore, they showed that the ternary alloy exhibited the highest osteogenic differentiation potential compared to other alloys with a good combination of mechanical and degradation properties.
Mg alloys containing Zn and Mn elements (e.g., Mg–2Zn–0.2Mn and Mg–1.2Mn–1.0Zn) were studied by Zhang et al.49 The alloys showed satisfactory mechanical properties and biodegradation performance. However, it was shown that Mn and Zn-based alloys degraded relatively faster. For instance, a 9-week implant of Mg–Zn–Mn-based alloy revealed 10–17% degradation, whereas, after 8 weeks, the degradation of the same implant increased up to 54%.
Therefore, it is evident that unlike Fe-based alloys, Mg-based alloys showed very promising results in terms of degradation and are highly regarded as potential biodegradable implant material candidates. Nevertheless, the faster degradation with simultaneous loss of mechanical integrity is still the primary impediment in the clinical use of Mg-based alloys. This indicates that new ways of controlling the degradation rate matching with bone healing while maintaining favorable biomechanical integrity are crucial to leverage the full benefits of the Mg-based degradable implant/device material.
2.3 Polymer-based degradable materials
Like metal-based degradable materials, biodegradable polymers are widely used as important bone repair implants and for tissue engineering applications, where load-bearing by an implant is not an essential requirement.50 There are two major classes of polymers – natural (e.g., chitosan, silk fibroin, collagen, etc.) and synthetic (e.g., PLA, PCL) polymers. However, because of high physiological activity, high degradation rate, and poor mechanical properties, natural polymers have limited application. Collagen is generally used as a decellularized tissue construct prepared by physical, chemical, or enzymatic decellularization of tissues. The immune response and sterilization are some of the key limitations associated with collagen.5 Chitosan is another naturally occurring degradable polymer. Owing to its poor strength, chitosan is primarily used as a hydrogel and sponge in biomedical applications.4 Silk fibroin is obtained by degumming of silk cocoons. It is used in drug delivery and tissue engineering applications.6 Silk fibroin-based coating was applied on magnesium alloys to improve the corrosion resistance.51,52 Instead, researchers have intensely focused on developing synthetic polymers with tailored properties to address the stringent requirements of medical devices/implants. Broadly, three synthetic degradable polymers, namely PLA, PGA, and PCL, are commonly studied, as their safe use and degradation within the body have been demonstrated. Historically, surgical sutures and meshes have been prepared from these polymers.53 PLA was first reported in 1960 and used as rods for mandibular fractures in dogs. Since then, the use of degradable PLA has ramped up with the continual development of new derivatives of PLA, such as L-PLA and DL-PLA.8 Based on glycolic acid, synthetic PGA has high crystallinity, melting point, and low solubility and was first introduced in a similar timeline of PLA as degradable suture lines.54 But PGA degrades at a relatively faster rate due to its hydrophilic surface properties. To address this limitation, a combination of a copolymer of PLA and PGA, forming a new PLGA variant, is developed and used as biodegradable fibers. A very unique and popular PLGA bone fixation product is the Lactosorb Craniomaxillofacial Fixation System developed by Biomet. On the other hand, PCL, a biodegradable aliphatic polyester, is predominantly employed for use in implants. Due to its hydrophobicity and high crystallinity, PCL shows much slower degradation than PLA and PGA.55 Furthermore, PCL can be easily formed into various geometric shapes, making PCL a popular candidate as a medical implant/device in the medical industry.56 Apart from these, synthetic degradable polymers have been successfully prepared from naturally occurring monomers such as polyols57 and plant oils58,59 for various biomedical applications, including drug delivery and bone tissue engineering scaffolds. Several factors such as easy availability, low cost, and high reactivity of the monomers make them attractive substitutes prepared from renewable resources for degradable polymer synthesis over polymer derived from petrochemical sources.
Biodegradation of polymeric implants in the human body fluid environment occurs through two key mechanisms. First, it starts with physical biodegradation where microorganism attacks the polymer, causing hydrolysis and ionization to form polymer-like oligomer debris. Second, the polymer degrades through chemical reactions and direct interaction with the abundance of microorganisms or enzymes, resulting in micro molecules forming carbon dioxide and water by-products.60 Despite excellent degradation properties and biocompatibility, biodegradable polymers have very limited use for diverse medical devices applications. This is mainly due to its very low stiffness and strength, X-ray transparency, and in many cases, accelerated degradation and foreign body reactivity, depending on individual patients. As a result, further surface modifications of biodegradable polymers are often sought after to control the degradation rate, enhance the biocompatibility, and improve the mechanical properties.
3. Bioactive ceramic coatings
In this section, we describe a variety of bioactive ceramics that have been successfully applied to degradable biomaterials. They offer several benefits such as antimicrobial activity, tissue formation activity such as osteogenesis, angiogenesis, and other properties such as corrosion resistance and mechanical properties (Fig. 1).
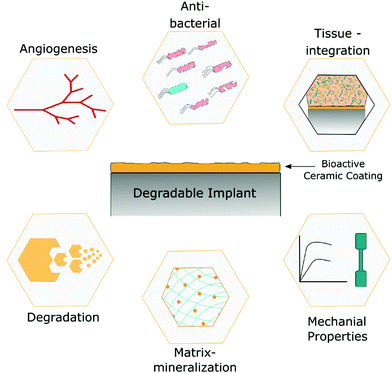 |
| Fig. 1 Bioactive ceramic-coated implants and their potential role in improving the overall performance of the implant. | |
3.1 Zinc oxide
Zinc oxide (ZnO) is widely used in food packaging and textile coating applications as an antimicrobial agent. A variety of mechanisms underlying the antibacterial activity of ZnO particles have been reported. Zn2+ ions released from the particles damage the bacterial membrane, resulting in leakage of intracellular matter. The generation of reactive oxygen species (ROS) by ZnO and its penetration into the bacterial cell envelope are among the other proposed mechanisms.61 Nevertheless, the mechanism underlying the antimicrobial action of ZnO particles continues to be an active topic of research. There are also several mechanisms reported in the literature that describe the osteogenic activity of ZnO. The release of Zn2+ ions from the ZnO particles primarily enhances osteocalcin expression by activating the mitogen-activated protein kinase pathway. Upregulation of Z1P1 expression, a transporter of Zn2+ ions, promotes the RUNX2 gene expression.62 Upregulation of these genes is shown to improve new bone formation. On contact with water molecules, ZnO particles generate Zn–OH functional groups at the surface. These groups act as nucleation sites for hydroxyapatite (HA) formation and further enhance the mineralization process to support osteogenesis.63 It has also been shown that ZnO coating helped in stimulating the macrophage polarization to both M1 and M2 phenotypes and improve the osteogenic activity. Thus, ZnO also enhances osteogenesis by modulation of the immune response.64 ZnO particles have been widely explored in anticancer applications. The large release of Zn ions causes an increase in ROS levels that induced cancer cell death by apoptosis.65 ZnO exhibits anti-inflammatory activity by suppressing the gene and protein expression of pro-inflammatory cytokines such as iNOS, COX-2, IL-1β, IL-6, and TNF-α. These antioxidant properties make ZnO an attractive ingredient in sunscreens and for the treatment of acne and piles.66 These numerous properties of ZnO make it ideal for modifying the surface of the degradable implant.
Cho et al. showed that a coating of ZnO on a 3D printed PCL/HA composite enhanced the antibacterial properties of the scaffold. In addition to its antibacterial action, the release of ions from the ZnO coating also improved the cell attachment and promoted mesenchymal stem cell differentiation. They further showed that the release of ions from the coating depends on the coating thickness on the scaffold surface, which was deposited by magnetron sputtering. The antibacterial potential increased with the increased thickness of ZnO coating, as revealed by the plate count method along with SEM and live–dead imaging (Fig. 2). A coating thickness of 100 nm imparted the best combination of antibacterial and bioactive properties to the scaffold.67 Bacterial attachment and encrustation are the significant clinical challenges encountered after implanting urinal stents, which cause inflammation and discomfort to the patient. A coating of ZnO was applied on PLA films using the doctor blade technique. ZnO-coated PLA films showed improved antibacterial potential compared to the bare film. The coating of ZnO was fully degraded after three weeks of incubation in artificial urine and displayed limited deposition of encrustation on films, demonstrating the potential of such ZnO-coated polymers for developing resorbable stents.68
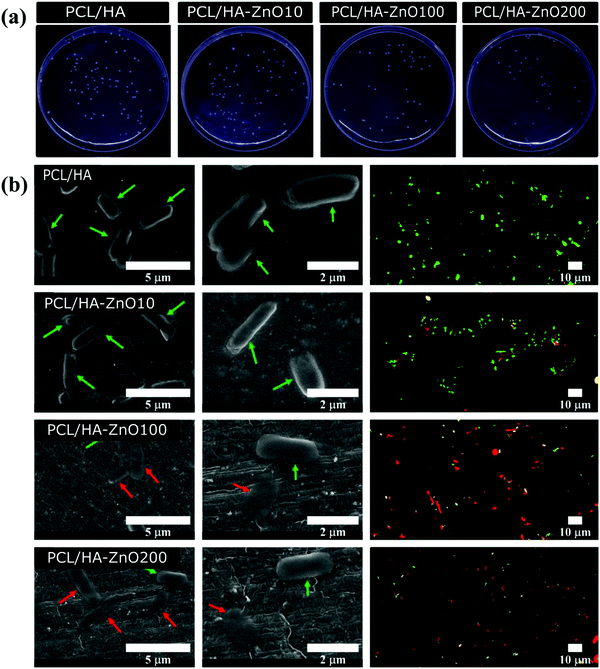 |
| Fig. 2 Antibacterial activity of ZnO coatings of different thicknesses on the PCL/HA 3D printed scaffold analyzed by (a) plate count method, (b) SEM and live–dead fluorescence imaging (dead bacteria in red and live bacteria in green) [Reprinted with permission from ref. 67. Copyright 2020 MDPI]. | |
As stated above, the rapid corrosion rate of Mg limits its clinical use to prepare implants. The surface modification with a suitable bioactive ceramic coating is one of the promising solutions to control the corrosion of Mg-based implants. ZnO coating has been applied on Mg implants to improve their corrosion resistance along with antibacterial potential. Undoped ZnO coating was deposited on magnesium implants by magnetron sputtering to improve their corrosion resistance and surface hardness.69 Composite ZnO coatings on Mg alloys such as Ag-doped ZnO,70 PLA–ZnO,71 PCL–chitosan (CS)–ZnO,72 and polypyrrole (Ppy)–ZnO73 were reported to improve the corrosion resistance and antibacterial potential compared to the bare surface.
3.2 Zirconium oxide
Zirconium oxide (zirconia) has widespread applications as dental implants and in the coating on metallic implants to increase their corrosion resistance. Hip implants74 and dental implants75 prepared from zirconia have shown long-term clinical success because of their good biocompatibility, strength, and wear resistance. Zirconia has been reported to activate the BMP4 gene.76 This gene is involved in early bone development and induces bone growth. Furthermore, it was reported that zirconia influences the spreading and proliferation of osteoblasts and stem cells with improved cell division and helps in forming a well-organized monolayer of cells. These cell-implant interactions prevent the aseptic loosening of the implant and facilitate its bio-integration.77 Thomas et al. demonstrated that zirconia-polydimethylsiloxane hybrid films improve the growth and viability of osteoblasts and fibroblasts. Thus, coatings prepared using this hybrid material enhance the bio-integration at the tissue–implant interface.76 Seweryn et al. reported the atomic layer deposition technique to generate uniform thickness zirconia coating on the substrate. They showed that the coating had biomimetic properties when tested with osteoblasts and thus increased the osteogenic potential with enhanced bone integration.78 Zirconia is also used as an antimicrobial agent in biomedical applications. The following antibacterial mechanism has been proposed in the case of zirconia. Zirconia interacts with the thiol groups found in the respiratory enzymes in bacterial cells. It binds to the bacterial cell membrane and disturbs the respiratory process of bacteria.79 Khanmohammadi et al. described the antimicrobial activity of zirconia against E. coli and S. aureus. Zirconia-doped PCL/cellulose nanofibers were prepared by electrospinning. These coated scaffolds also showed antifungal activity when tested against Candida albicans.80 These studies show the use of zirconia as a material to improve osseointegration along with effective osteogenic and antibacterial properties.
Huang et al. showed that the coating of zirconia on the AZ91 Mg alloy improved the corrosion resistance along with surface hardness.81 Furthermore, Yang et al. showed that a zirconia coating on the Mg–Sr alloy improved the corrosion resistance along with enhanced attachment, proliferation, and differentiation of osteoblasts. They used atomic layer deposition to coat the alloy surfaces with zirconia with a different number of cycles. The coating thickness increased with the number of cycles, which improved the corrosion resistance, as shown in Fig. 3.82 Therefore, zirconia coating can be used to tailor the biocompatibility and corrosion resistance of degradable orthopedic and dental implants.
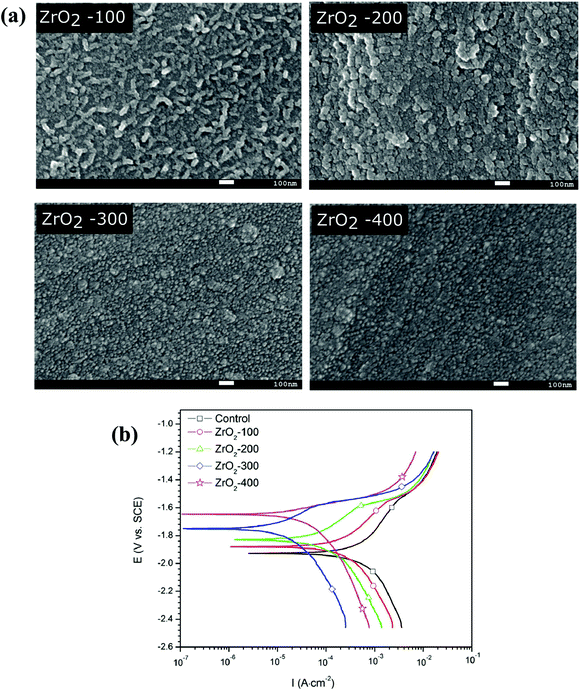 |
| Fig. 3 (a) Surface morphologies of zirconia (ZrO2) with different cycles of atomic layer deposition (i.e., 100, 200, and 300) imaged by SEM, (b) potentiodynamic polarization curves of different coated samples along with the uncoated sample [Reprinted with permission from ref. 82. Copyright 2017 Elsevier]. | |
3.3 Titanium oxide
Titanium oxide is widely used in biomedical applications as an antibacterial and anticorrosive agent. Titanium oxide exhibits photocatalytic activity that generates electron–hole pairs on exposure to UV light. These electron–hole pairs produce ROS in the vicinity of the particle. ROS oxidizes bacterial intracellular enzymes, reduces respiratory action, and subsequently causes bacterial death.83 Improvement in the antibacterial properties of polylactic acid/titanium oxide films after the doping of titanium oxide nanoparticles was reported by Feng et al.84 They showed that the composite film inhibits the growth of bacteria when compared with the pristine film. According to Matsuno et al.,85 PLA surface modified with titanium oxide improves the initial attachment of fibroblasts and their subsequent proliferation. Titanium and its alloys have been frequently used in the orthopedic field due to their high strength and low density. Titanium and alloys are completely inert as they spontaneously form a layer of titanium dioxide on the surface. This oxide layer protects the implant from body fluids and tissue corrosion. As the oxide layer comes in contact with water, it forms Ti–OH groups on the surface. Similar to the ZnO layer, these groups act as nucleation sites for different calcium phosphate compounds owing to the close match between the crystallographic planes of the oxide and calcium phosphate.86 The similarity of calcium phosphate with the bone mineral phase augments the integration of the implant with contacting tissue. Thus, the titanium oxide layer imparts anticorrosive properties and tissue bonding ability to Mg implants, which has been leveraged in a few studies.87–89
The in vivo osseointegration potential of the polyetheretherketone implant coated with titanium oxide was reported by Tsou et al.90 They showed that a titanium oxide coating prepared by arc ion plating not only improves the compatibility when tested with osteoblasts but also enhances the new bone formation and tissue integration. This study demonstrates that titanium oxide coating can be deposited on degradable polymer implants to enhance osseointegration and antibacterial properties, although such coatings have not been applied on degradable polymeric implants.
3.4 Vanadium oxide
Vanadium is one of the transition metal elements. Like most of the transition metals, vanadium also has multiple oxidation states. Among many oxides of vanadium, vanadium pentoxide (vanadia) nanostructures have been used in biomedical applications as an antibacterial agent. There are two mechanisms reported for the antibacterial properties of vanadia nanostructures. The first mechanism was reported by Anicic et al. in 2017.91 They showed that vanadia nanostructures mimic the myeloperoxidase-like catalytic activity of human neutrophils. The role of this enzyme is to kill the bacteria by the transformation of hydrogen peroxide to hypochlorite. In addition to this activity, it also promotes the growth of mammalian cells via the insulin-mimicking effect. This bioactivity was observed at a low concentration of vanadate ions in the system. At low concentrations, these ions also help in tissue repair and improve the bone formation rate in vivo. In contrast, a high concentration of vanadate ions is toxic to human cells.91 Hence, control and sustained release of vanadate ions are key to leverage the above said beneficial properties. Vanadia nanowires/poly(lactic-co-glycolic acid) composites have been shown to satisfy the above criteria and enhance the antibacterial activity. Vanadia nanoplates have been shown to increase the generation of ROS in bacterial biofilms. Increased ROS production causes bacterial death. Raman spectral mapping demonstrated the changes in biomolecular signals from bacteria following interactions with vanadia nanoplates.92 Menazea et al. showed that the addition of vanadia to chitosan/polyvinyl alcohol coating enhances the antibacterial properties of the film. The antibacterial activity of the films increased with an increase in the doping of vanadia nanoparticles up to a saturating amount.93 In addition to this, vanadia coating deposited on Mg alloys was prepared by dip-coating94 and micro-arc oxidation95 to improve the corrosion and wear resistance. All these observations from several studies demonstrate the potential of vanadia as a surface coating material for implants to improve the corrosion resistance and antibacterial properties toward minimizing implant-associated infections and facilitate healing by controlling the release of hydrogen gas in the process of corrosion.
3.5 Copper oxide
Copper oxide nanoparticles have been successfully applied in a wide variety of fields, including nanoelectronics, solar cells, and catalysis. Apart from these applications, it also possesses remarkable antibacterial properties. The antibacterial properties of copper oxide are attributed to the release of Cu2+ ions, which causes severe damage to the negatively charged lipids and proteins of the bacterial cell wall. Moreover, copper ions have high redox properties, which generate ROS. ROS causes DNA damage and modification of cellular components in bacterial cells.96 According to Escobar et al., doping of copper oxide nanoparticles into the PCL electrospun fibers resulted in significant bactericidal action on Gram-negative bacteria compared to Gram-positive bacteria. This difference is because Gram-negative bacteria have thin cell walls in contrast to Gram-positive bacteria, resulting in easier exposure to copper ions.97
Munoz et al. prepared copper oxide infused PCL nanofibers by electrospinning. Their work reveals that the infused fibrous mats showed an antifungal effect against various species of candida. Furthermore, they also observed that the cell morphology of candida was disrupted in a dose-dependent manner on the infused mat.98 The toxicity of copper ions released from nanoparticles on mammalian cells along with bacterial cells was first reported by Balcucho et al.99 They prepared a composite film of PCL and copper nanoparticles by the solution blending method. They observed that the composite film showed minimal DNA damage and significant cell viability of human foreskin fibroblasts while showing excellent antibacterial potential against S. aureus. Badaraev et al. coated the surface of the electrospun PLA scaffold with copper/copper oxide by magnetron sputtering. They observed that the coating remarkably improved the antibacterial potential of the scaffold.100 Such a strategy of depositing copper/copper oxide coatings on the degradable implants has significant potential for enhancing their antibacterial properties.
Copper ions have been shown to enhance the proliferation of endothelial cells and promote angiogenesis. Copper is required to activate hypoxia-induced factor-1, which is the primary transcription factor controlling the expression of vascular endothelial growth factor (VEGF). VEGF is mainly involved in the creation and maturation of blood vessels.101 This potential imparts one more biochemical aspect to the coating of copper oxide, which has been utilized in several studies to enhance angiogenesis.102–105
3.6 Iron oxide
Like most transition metal oxides, iron oxide also shows antibacterial potential. Various studies have reported this activity of iron oxide against Gram-positive and Gram-negative bacterial strains. The ions released from the nanoparticles attracted negatively charged bacterial cells and attached to the cell membrane or penetrated inside the bacterial cells. The primary mechanism was the production of ROS due to these ions, which disrupt the bacterial cell wall and induce DNA damage.106–108 Several strategies have been reported on the use of iron oxide to prepare coatings on polymer surfaces to improve their antibacterial activity.109,110
Apart from their antimicrobial activity, superparamagnetic iron oxide particles generate heat after applying an alternating magnetic field. Initially, this property was investigated for treating cancerous tumors, but such magnetic hyperthermia is being explored for treating implant-associated infections in more recent times. Rovers et al.111 developed a system of iron oxide particles and thermoresponsive polymer poly(butyl methacrylate-stat-methyl methacrylate) for the delivery of antibacterial drugs based on magnetic hypothermia induced by the iron oxide particles. On the application of a magnetic field to the system, the heat generated causes an increase in the temperature of the thermoresponsive polymer to its glass transition temperature. This heating causes the release of the drug-loaded in thermoresponsive polymers due to the increased diffusion rate near Tg. They observed that the drug release increased up to 5-fold after the application of the magnetic field, and the release is dependent upon the iron oxide content. A similar strategy can be implemented for the smart release of antibiotics using biodegradable and thermoresponsive polymers such as poly(lactide-co-trimethylene carbonate) to tackle implant-based bacterial infections. Numerous studies have reported using iron oxide particle's magnetic hypothermia to eradicate bacterial infection.112,113 These various properties of iron oxide can be utilized to develop drug/biomolecule eluting coatings on degradable implant surfaces to enhance the bioactivity of the materials.
3.7 Cerium oxide
Cerium is a rare earth metal placed second in the lanthanide series of the periodic table. Most of the rare earth metals exist in their trivalent state. However, cerium occurs in both trivalent (+3) as well as tetravalent (+4) states.114,115 Consequently, cerium oxide (ceria) has the unique ability to switch between the two oxidation states (Ce2O3 and CeO2). During oxygen exchange, loss of lattice oxygen atoms in the cubic fluorite lattice causes the generation of oxygen vacancies in ceria. This vacancy can be filled by atomic oxygen, which generates a new vacancy, causing lattice oxygen mobility and rapid refilling of the surface oxygen vacancy sites.116 Ceria is widely used in various applications, such as catalytic converters, solid oxide fuel cells,117 electronics, and electrochemistry.118
In recent years, ceria has been attracting considerable attention in biomedical applications due to its redox properties. Ceria mimics superoxide dismutase (SOD) and catalase (CAT) enzymes, which act as cellular antioxidants. Korsvik et al. demonstrated that the oxygen vacancy created on the surface is mainly responsible for the SOD-mimicking activity of ceria.119 Ceria is used as an antibacterial agent in various cases. Faris et al. described the antimicrobial activity of ceria nanoparticles against both Gram-positive and Gram-negative bacteria. The interchange of the oxidation state disrupts the bacterial cell membrane and results in bacterial death.120 Antifungal activity of ceria has also been observed.121 Several studies described the ability of ceria nanoparticles to induce angiogenesis, which makes it an attractive candidate for a bioactive implant. Das et al. described that ceria nanoparticles induce angiogenesis in human umbilical vein endothelial cells (HUVECs) by regulating intracellular oxygen.122 Corroborating the angiogenic activity of these particles, Augustine et al. showed that infusion of nanoceria in electrospun PCL nanofibers promoted angiogenesis compared to the neat fibers.123
The ceria-loaded gelatin–alginate nanocomposite was developed by a freeze-dried method having an interconnected porous morphology. The addition of ceria to the composite helped in the reduction of ROS and promoted bone formation. Apart from biological cues, the addition of ceria enhances the composite's mechanical, swelling, and degradation properties.124 Our group also demonstrated the development of ceria particle coated PCL–gelatin electrospun nanofibrous mats. Depletion of ROS is reported to be in relation to the suppression of hypotrophy in cardiac tissue. The H2O2 stress-induced oxidative stress level showed a remarkable reduction in cardiomyocytes cultured on decorated scaffolds. Thus it showed that coating ceria particles imparts antioxidant and anti-hypertrophic properties to the cardiac patch.125 The deposition of ceria conversion coatings on Mg alloys with different additives and pre-treatments has been reported in numerous studies to improve the corrosion resistance.126,127 The ceria-coated titanium substrate fabricated by spin coating showed improved antibacterial and anti-inflammatory potential.128 A similar strategy can be applied to degradable implants to impart various properties, but such studies are not reported.
3.8 Europium hydroxide/oxide
Europium is also one of the rare earth metals and is the most reactive lanthanide series element. Europium hydroxide/oxide ceramic materials have been studied in the last decade as pro-angiogenic materials in biomedical applications such as tissue engineering, wound healing, and treating ischemic conditions.129,130 Europium hydroxide nanorods cause an increase in the production of H2O2 ROS. This surge of ROS activates the enzyme called nitric oxide synthase 3, which induces the secretion of nitric oxide in the system. These molecular events stimulate new capillaries to sprout from the existing blood vessel in a process called angiogenesis.131 Patra et al. were the first to report the biological effects of europium hydroxide nanorods on the HUVECs. They showed that these ceramic nanorods promote the proliferation of HUVECs and vascular sprouting in chick embryo chorioallantoic membrane (CAM) assay, which are functional evidence of their angiogenic activity.132 These observations laid the foundation for several subsequent investigations on the pro-angiogenic activity of this material for various biomedical applications. Infusion of europium hydroxide nanorods in PCL nanofibrous mats augmented angiogenesis in vitro and in vivo. HUVECs seeded on the composite fibers showed better proliferation in comparison to neat fibers. Western blot analysis revealed increased expression of angiogenic markers in cells cultured on the nanorod-loaded electrospun mats.133 Angiogenesis and vascularization are essential phenomena in the process of wound healing and skin regeneration. Luo et al. showed the benefit of doping europium oxide nanorods in hydrogels for wound healing and skin tissue regeneration by aiding angiogenesis and suppressing inflammation at the wounded site.130 Furthermore, europium-doped bioglass also showed the ability to stimulate osteogenesis in vitro and in vivo.129 These several studies exhibit the potential of europium oxide/hydroxide ceramic materials as coating materials for polymeric or metallic implants. However, studies on their use as surface coatings on degradable materials have not been reported.
3.9 Bioactive glass
Specific chemical compositions of silica-based glass-ceramics containing Ca and phosphate ions are known for their unique binding ability to living tissues, thereby forming a strong tissue–biomaterial interface. This class of ceramics is known as bioactive glass. The first bioactive glass was reported way back in 1969, which is a quaternary system of SiO2–Na2O–CaO–P2O5 and is known as 45S5 Bioglass®.134 Subsequently, several phosphate-, silicate-, and borate-based bioactive glass systems have been explored for biomedical applications.19 Bioactive glasses develop a strong bonding with hard tissues such as bone and dental tissues and also with several soft tissues.135 After forming a stable interface, a bioactive glass elicits a variety of biological responses, including new tissue formation, angiogenesis,102 bactericidal action,136,137etc. The above said properties are associated with the time-dependent dynamic modification of the surface after implantation in a series of reactions. The bioactive glass has a natural tendency for biodegradation and releases various ions into its vicinity, which primarily depends upon the glass composition and the local pH. When the bioactive glass surface comes into contact with body fluid, it forms a network with Si in the glass. This network further draws the movement of Ca, and phosphate ions from the core to the Si reach layer at the surface, causing the formation of a biologically active carbonate–HA layer, which provides a bonding interface with tissue.138
The dissolution products of the bioactive glass, such as Ca and silicate ions, help the cells to form new tissue. The ions released from doped bioactive glass elicit additional biological responses. For example, Sr2+ stimulates bone growth,139,140 and Cu2+ helps in angiogenesis,102 whereas the release of Ag+ enhances the antibacterial potential136,141 of doped glass. These attributes of bioactive glass make it a suitable material for coating implants. The coating of glass on metallic implants imparts bioactivity, augments bio-integration, and enhances corrosion resistance. The coating of bioactive glass on the metallic implant surface has been described in numerous review papers. For example, Yadav et al. highlighted the coating of bioactive glass on Mg and its alloys to improve their corrosion resistance. They also summarized the effect of interfacial aspects such as the composition of bioactive glass, coating thickness, adhesive strength, and wettability on corrosion of Mg and its alloys.142 Sergi et al. reviewed the different methods of coating bioactive glass on metallic implants.135
On polymeric implants, bioactive glass coating provides mechanical reinforcement and tunable degradation of the polymeric substrate in addition to improved biocompatibility and bioactivity. Roether et al. reported bioactive glass coating on microporous PLA foam for tissue engineering applications. They utilized the slurry dipping method to get a uniform coating on the porous polymer scaffold. The in vitro biomineralization study in simulated body fluid (SBF) generated an HA layer on the surface. They further showed that the thickness of the HA layer increased with the time of incubation in SBF.143 Zhu et al. developed a mesoporous bioactive glass coating on the microporous PLA scaffold for the sustained release of an antimicrobial drug. They showed that mesoporous bioactive glass coating resulted in sustained release of an antimicrobial drug loaded in the coating to eradicate the bacterial infection, apart from improved bioactivity due to the development of the HA layer.144
Bioactive coatings were initially employed primarily for hard tissue engineering, but such coatings have been increasingly explored for soft tissue engineering over the last decade. Blaker et al. developed Ag-doped degradable polymer sutures using the dip-coating process. Their study revealed that the coated suture showed improved in vitro bioactivity and antibacterial potential compared to uncoated sutures.141 Zn-doped bioactive glass coating on sutures for antibacterial application is also reported in the literature.137 Thus, all these studies reveal that the bioactive glass coating provides a combination of enhanced biological and mechanical properties with tunable degradation to degradable polymeric implants.
3.10 Calcium phosphate-based ceramics
The family of calcium phosphate (CaP)-based ceramics are the most common bioceramics used in biomedical applications. HA is the most stable of CaP compounds at physiological pH, because of which HA is the most exploited CaP phase among them. Other CaP-based compounds that have shown promise in biomedical applications include tricalcium phosphate (TCP),145 dicalcium phosphate dihydrate (DCPD),146 amorphous calcium phosphate,147 and octacalcium phosphate.137 HA is the bioceramic of choice due to its similarity to the mineral phase of natural bone. HA has been shown to form a chemical bond with the bone and establish a robust interface between the implant and tissue, thereby providing early fixation to fasten implants with the surrounding tissue. Apart from this, fluoridated HA evokes antibacterial potential against several bacterial strains.148 In addition to fluorine, the incorporation of Ag149,150 and Zn151,152 into HA also imparts antibacterial potential. A porous HA layer can also act as a reservoir for antibacterial drugs and biomolecules, ensuring the sustained release of the stored molecules.153,154 The doping of copper in HA is known to enhance angiogenesis.103,104 Implants made out of CaP alone tend to be brittle, limiting their use in many applications. Consequently, HA emerged as an attractive coating material for biomedical implants.
The dissolution rate is important as it determines the release of Ca and phosphate ions from the CaP layer, which modulates the cellular response. For a slow degrading CaP layer, the surface topography drives the cell behavior, whereas the release of ions plays a more dominant role in fast degrading ceramic coatings. As HA has a much slower dissolution rate at physiological pH compared to β-TCP, biphasic calcium phosphate, a mixture of HA and β-TCP, is used to tailor the degradation rate. It has been shown that increasing the concentration of β-TCP in the mixture improved the differentiation of stem cells by upregulating osteogenic genes. This effect is associated with the increased release of the ions from the β-TCP component.145,155
HA coating on Mg implants can change the surface morphology and enhance corrosion resistance along with improved osseointegration. The use of CaP-based coating on Mg implants has been comprehensively reviewed in various articles.156–158 Although HA is a promising material for coating implants, it suffers from some limitations, such as poor adhesion strength to the substrate and insufficient chemical stability, which might compromise the implant's long-term benefit. The shot peening with ceramic balls followed by electrodeposition of DCPD coating on magnesium AZ31 alloys was shown to improve the adhesion strength of the coating along with improved corrosion resistance.159 Shi et al. prepared a composite coating of the oxide layer by micro-arc oxidation followed by the DCPD layer through electrodeposition on a magnesium AZ80 alloy substrate. They showed that the composite coating imparted excellent corrosion resistance along with enhanced deposition of appetite.146 Like bioactive glass coatings, CaP coatings improve the bioactivity along with mechanical stability and degradation of polymeric substrates. The CaP-based coating has been applied on several types of polymeric scaffolds, such as porous scaffolds prepared by porogen leaching,160,161 electrospun nanofibrous mats,162,163 and 3D printed scaffolds.164,165 Treatment of a porous composite PLA/HA scaffold with air plasma improved the nucleation of apatite when the scaffolds were incubated in SBF. Rapid healing and faster integration with bone were observed for plasma-treated composite scaffolds in vivo than pure PLA scaffolds.161 Guo et al. fabricated porous PLA scaffolds maintaining the HA layer on the pore wall using ice–HA hybrid particles as porogens. They showed that the method could be tailored to control the pore size and deposit uniformly thick HA on pore walls.160
Different surface treatments were incorporated to reduce the time duration of the biomineralization process in SBF and deposit an apatite layer. These treatments include silicate,166 ammonia alkali,167 and grafting of citric acid-polyethylene imine.168 For example, our group reported that the surface treatment with citric acid-polyethylene imine reduced the time required to form the apatite layer to 7 days from 21 to 28 days required in traditional techniques. Citric acid helps to increase the precipitation of Ca and phosphate ions when incubated in SBF.168 Poh et al. developed CaP-coated and two different bioactive glass-doped 3D printed PCL scaffolds. Their study revealed that the coating and incorporation of both the ceramics showed improved osteogenic potential in vitro. They implanted neat PCL scaffolds and sheep bone marrow stromal cell-seeded PCL scaffolds in Rowett Nude rats subcutaneously. The study revealed that under all the conditions, combinations of the fibrous connective tissue and adipose tissue formed inside the pores of the scaffold, confirming tissue infiltration in the scaffold. In spite of that, mature bone was not observed in vivo in any group (Fig. 4).169 Plasma spraying of HA particles on the carbon fiber/polyamide implant increased the osteogenic potential of the substrate.170 A similar kind of coating method can be used for degradable polymer implants for orthopedic applications.
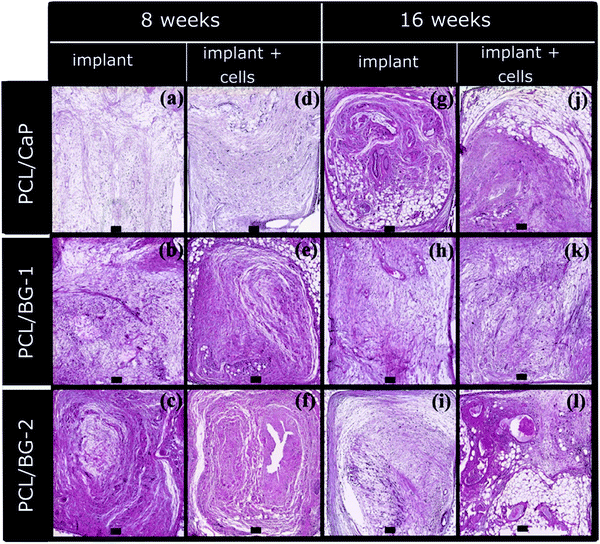 |
| Fig. 4 Hematoxylin and eosin-stained images of CaP coated 3D printed PCL and two different bioactive glass-incorporated 3D printed PCL scaffolds implanted with and without cell seeding after 8 and 16 weeks of implantation. [Reprinted with permission from ref. 169. Copyright 2016 Elsevier]. | |
3.11 Carbide and nitride-based ceramics
Carbides and nitrides are a major class of ceramics popularly used as coatings on biomedical implants after oxides. Generally, nitrides and carbides are extremely hard ceramic materials with very high moduli. These ceramics are difficult to fabricate into different implant shapes. Hence coatings of nitrides and carbides are used on biomedical implants to improve the surface properties of substrates. Coatings of carbides and nitrides are primarily applied on metallic implants. Among the carbides, titanium carbide and silicon carbide are the most widely reported in the scientific literature. Titanium carbide is known to have superior adhesion to different metallic substrates,171 good wear resistance under dry and wet conditions,172 good corrosion resistance,173 and improved biocompatibility that mediates bio-integration when applied as a coating on a metallic substrate. The oxide layer formed on metallic implants such as titanium is brittle in nature and can fracture easily. Consequently, the underlying titanium surface can be exposed to severe corrosion in the biological environment that releases titanium particle ions inducing toxicity to adjacent tissues, resulting in inflammation and implant failure. Application of titanium carbide on the implant surface overcomes this problem by increasing the corrosion and wear resistance while maintaining biocompatibility.174 Brama et al. developed a titanium carbide coating on titanium by pulsed laser deposition. They showed that the coating improved the corrosion resistance along with enhanced growth, attachment, and osteogenic differentiation of osteoblasts in vitro. In vivo experiments in rabbit and sheep models demonstrated significant bone formation on titanium carbide-coated samples compared to neat titanium samples.172 Pushpanathan et al. showed that electrodeposited titanium carbide on the AZ8A Mg alloy demonstrated improved corrosion and wear potential.175
Silicon carbide has also been used to coat metallic implants to improve their corrosion resistance under biological conditions. Silicon carbide has been known for its high strength, resistance to the corrosive biological environment, and ease of deposition.176 Li et al. showed that silicon carbide-coated WE43 Mg alloys demonstrated anti-thrombogenic potential and improved hemocompatibility along with better corrosion resistance; thus, it can be used to coat coronary stents.177
Nitride-based ceramics are also used to coat metallic implants, given their corrosion and wear resistance, which augment their long-term success in vivo. Titanium nitride has been widely used to coat Mg implants.178–180 Moreover, titanium nitride coating also improves the biocompatibility of implants in a manner similar to that of carbides. Annunziata et al. showed that coating of titanium nitride supported the attachment and growth of mesenchymal stem cells, whereas decreased bacterial attachment and growth were observed.181 The application of titanium nitride for coating medical implants has been summarized in other review articles.182,183
3.12 Miscellaneous ceramics
Some of the bioceramics have received significant attention and were used as coatings on various biomaterials, including degradable biomaterials, as described above. However, several other ceramic coatings have also been attempted, although they have received less attention. Moreover, these coatings have been attempted on non-degradable substrates, although they can also be tried for degradable biomaterials. This section briefly compiles the work done on these less explored ceramic coatings.
Strontium is present in a minimal amount in human bones. Strontium ions have a charge to size ratio that is similar to that of and can thus replace Ca2+ ions in various molecular events of osteogenesis. Strontium in a low concentration promotes the activity of osteoblasts and stimulates new bone formation.184 At the same time, it suppresses the differentiation of osteoclasts and reduces bone resorption.185 Based on these properties, strontium-containing ceramics have been used as a coating material for biomedical implants. Strontium-containing bioactive glass coatings,186 strontium oxide doped titanium oxide coatings,187 and strontium carbonate incorporated PCL electrospun fibers188 release strontium ions, thereby enhancing the osteogenic activity of the implants. Our group has developed strontium-eluting PCL nanofibrous mats by doping strontium carbonate into nanofibers. They demonstrated that doping improves the proliferation of mesenchymal stem cells in addition to enhanced osteogenic activity.189
Niobium pentoxide coating has been reported to facilitate the growth of the apatite layer when incubated in a concentrated solution of Ca and phosphate ions. Nanostructured niobium pentoxide coating on the Mg substrate prepared by the sol–gel method improved attachment, growth, and differentiation of osteoblasts.190 Improvement in the differentiation of mesenchymal stem cells was observed in the case of niobium oxide-doped glass-ceramics.191 In another study, niobium pentoxide-coated titanium implants exhibited improved bioactivity and corrosion resistance compared to uncoated ones.192
The antibacterial mechanism of magnesium oxide nanoparticles is unclear, but many researchers correlate it with ROS production, which alters the cellular components of bacteria resulting in cell death.193 Hickey et al. developed a magnesium oxide coating on PLA using electrophoretic deposition. Magnesium oxide coating showed improved antibacterial potential against both Gram-positive and Gram-negative bacteria. The coating also exhibited improved osteoblast attachment by activating integrins through the release of magnesium ions and increased hydrophilicity after coating. They also showed that the fast degrading characteristics of magnesium nanoparticles could be used to tailor the mechanical properties of an implant over time.194
3.13 Doped ceramics
The application of a single-component ceramic coating may not be sufficient to meet different clinical needs, such as improving osseointegration, inhibiting bacterial infection, enhancing corrosion-wear resistance, and promoting angiogenesis. To address this wide variety of requirements, doping different elements in the ceramic coating offers a promising strategy. As mentioned above, it is a general practice to incorporate different elements into bioactive glass and CaP coatings to elicit additional biological responses.195 Similarly, several studies have reported the use of doped ceramic coatings on medical implants.
As discussed above, the release of Zn ions from ZnO particles ruptures bacterial membranes and induces leakage of intracellular components. Strontium has been known to promote bone formation, as discussed above. Deposition of a titanium oxide layer doped with Zn and strontium on the titanium surface by micro-arc oxidation was reported by Zhao et al. The study revealed that incorporating elements improved the antibacterial performance and osseointegration of the implant.196 Copper-doped titanium oxide coating demonstrated improved antibacterial, angiogenic, and osteogenic abilities compared to pure titanium oxide coating.197 Manganese dioxides exhibit antioxidant catalase mimicking activity, which is attributed to the presence of oxygen vacancies on the surface. Similar to cerium oxide, manganese oxide suppresses oxidative stress by controlling the ROS content. The doping of Zn into manganese dioxide helped in generating more oxygen vacancies with increased structural instability, inhibiting the manganese dioxide crystal growth and reducing its layer thickness. Consequently, the doped manganese oxide-coated titanium implant showed enhanced antioxidant catalase-like activity compared to undoped coating. The doped coating also demonstrated improved attachment, proliferation, and differentiation of osteoblasts due to increased hydrophilicity and release of Mn and Zn ions from the coating.198
Xu et al. developed a HA coating on the Mg AZ60 alloy by a chemical conversion method, imparting enhanced bioactivity and bio-integration. But owing to the porous nature of the HA coating, it cannot improve the corrosion resistance. This challenge was resolved by depositing another coating of copper oxide-doped titanium oxide with improved corrosion resistance. The layered coating showed good biocompatibility in addition to enhanced antibacterial activity.199 Thus, doped ceramic coatings impart better biological properties in comparison to undoped coating.
4. Coating strategies
Coating strategies play a pivotal role in maximizing the benefits of an implant by improving the physical performance and biocompatibility of the biomaterial. Several popular strategies of applying coating materials on biomedical implants have been reported in the literature, including dip-coating, electrophoretic deposition, plasma electrolyte oxidation/micro-arc oxidation, hydrothermal synthesis, plasma spraying, and magnetron sputtering, as depicted in Fig. 5. Some of the popular techniques are briefly summarized here.
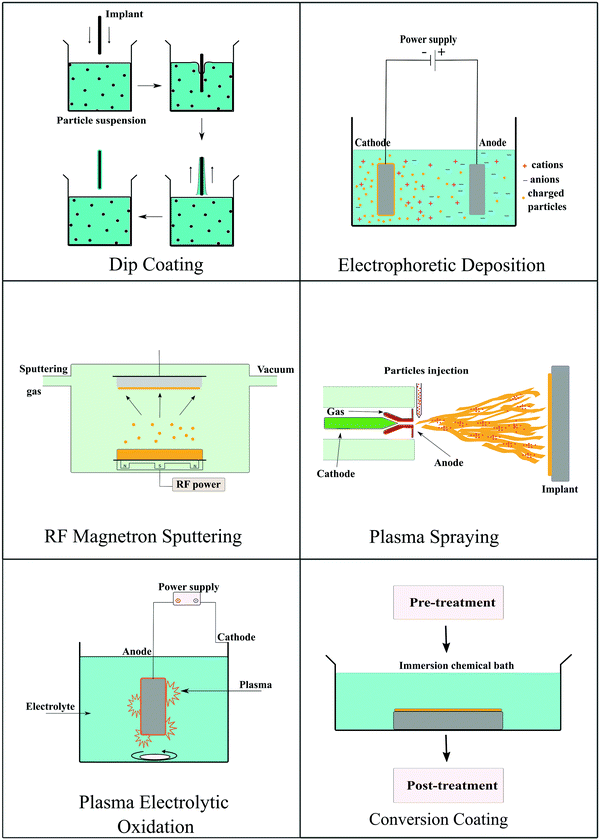 |
| Fig. 5 Schematic summarizing the coating strategies utilized for preparing bioactive ceramic coatings on the surface of degradable implants. | |
4.1 Dip coating
Dip coating is a conventional chemical process to apply a coating on a biomedical implant. In this process, implants are dipped in a suspension of colloidal particles for an appropriate period of time. A wide range of ceramic coatings has been applied on implant surfaces with porous structures using this technique. Porous coatings provide an increased surface area for interaction with body fluids and to interact with the surrounding tissue. The process is technically simple and cost-effective in comparison to other coating techniques.200 CaP coatings are prepared by dip-coating using the two-layer method. The implant is first incubated in a solution of Ca ions, such as CaCl2 or CaNO3·4H2O, and subsequently dipped in a solution of phosphate ions, such as (NH4)2HPO4 or K2HPO4·3H2O. Sometimes several dipping cycles are performed to achieve the desired thickness of the coating.201 Besides CaP coating, this technique can also be used to deposit titanium oxide, zirconia, and ZnO on Mg implants.202,203
4.2 Electrophoretic deposition
Electrophoretic deposition of coatings is based on the principle of electrophoresis. In electrophoresis, charged particles suspended in the solution migrate towards counter-charged electrodes under the influence of an electric field. The migrating particles accumulate at the oppositely charged electrode surface generating a uniform coating on the substrate. The solvent used in the process should have a suitable dielectric constant. The size of particles should be appropriate to remain stable in the colloidal state in the solvent. Hence, viscosity, conductivity, and zeta potential affect the thickness of the deposited film.204 Electrophoretic deposition is a simple, cheap, and rapid process that can deposit coatings on different substrates, even on complex shapes other than flat surfaces. Codeposition of different ceramic particles such as bioactive glass–HA,205 chitosan/alginate HA,206,207 and ZnO–HA208 has also been achieved using this method. The coating of homogenous ZnO–HA on a silicone substrate was done by using the electrophoretic deposition method for improving the antibacterial activity. The silicone substrate was pretreated with oxygen plasma, and a thin titanium layer was deposited by DC sputtering. This thin titanium film-covered silicone was used as a substrate electrode in the deposition process.208 Many studies have reported the deposition of HA on Mg alloys using the electrophoretic deposition technique.209–211
4.3 Radiofrequency magnetron sputtering
Radiofrequency magnetron sputtering is a low-pressure technique used to deposit controlled and uniformly thick coatings on biomedical implants. In this method, the desired coating material prepared in the form of a target, is bombarded by reactive ions from gaseous plasma generating free atoms. Liberated atoms then travel through the vacuum and deposit on the substrate to form a uniform coating. The thickness, structure, and deposition rate of the coating depend upon deposition pressure and reactive gas flow rate along with the duration of the process.212 Cho et al. fabricated a PCL/HA 3D printed scaffold with ZnO coating by this method. They demonstrated that the deposited coating was uniform, and the thickness could be controlled by changing the duration of sputtering.67 Al-doped ZnO coating was fabricated on PLA films by magnetron sputtering. ZnO/Al2O3 was used as a target to produce nanostructured coatings by varying the sputtering power.213
4.4 Plasma spraying
Plasma spraying is the most commonly used thermal spraying method to create a coating on an implant surface. In this technique, an electric arc of high temperature and pressure is used to create a plasma jet. Fine particles of coating material are then injected into the jet to form molten particles. These molten particles are further deposited onto the substrate in the form of flattened drops to yield a coating on the implant. The plasma spray process has a high deposition rate, and the thickness, morphology, and structure of the coating can be tailored by modifying the deposition parameters.214 The plasma spray strategy has been used for coating metallic and polymeric implants. HA powder was plasma sprayed on a polyamide-carbon fiber surface using a plasma gun and argon gas. The coating was highly crystalline with good mechanical adhesion to the composite substrate.170
4.5 Plasma electrolytic oxidation
This method has been commonly used to deposit bioactive ceramic coatings on Mg and its alloys. It is also known as the micro-arc oxidation process. This process is carried out in an electrolytic bath, and Mg acts as the working electrode. This process is similar to anodization, but it uses a higher potential to generate plasma/micro-arc. The resulting micro-arc/plasma helps in developing a thick deposit layer. The coating formed by this method has a high bonding strength with the substrate, and the thick hard coating can impart hardness, corrosion, and wear resistance. The composition of the electrolytic bath, substrate composition, and process parameters affect the coating performance.215 Zirconia,216 titanium oxide,89 and CaP217 based coatings are primarily deposited on Mg and its alloys using this technique.
4.6 Conversion coating
Conversion coating is a process of in situ formation of coating through chemical reactions between a substrate and a reaction additive. The nature of coating mainly depends upon the pre-treatment of the substrate and the type of treatment additive used. This process is very widely used for Mg and its alloys owing to their high reactivity.218 Magnesium fluoride and magnesium hydroxide-based conversion coatings are the most popular among all such coatings to improve the corrosion resistance of the substrate. The fluoride coating can stimulate osteoblast proliferation and increase mineral deposition.219 Cerium oxide126,127 and phosphate220,221 based conversion coatings on Mg implants have also been reported in the literature.
Except for the strategies mentioned above, there are other methods to deposit a bioactive ceramic coating on the implant surface. However, the single coating technique does not offer the desired outcome. Hence, two or more deposition strategies are being used in more recent times, mainly for Mg and its alloys.
5. Mechanical surface treatments
Mechanical surface treatments have been applied successfully to significantly improve the biomechanical performance and cellular response of several conventional metallic biomaterials, including stainless steels222 and titanium alloys.223,224 Severe plastic deformation is often found to induce nanocrystallization at the surface.225 In this section, we describe the recent advancements in applying surface mechanical treatment strategies, which have been deployed for degradable Mg-based biomaterials. These treatments provide benefits like corrosion resistance, tunable degradation, wear resistance, fatigue performance, surface hardness, and strength. Some of the extensively used surface mechanical treatment strategies and their effect on degradable implant properties are briefly summarized here. Fig. 6 compiles some of these strategies, such as shot peening, laser shock peening, ball burnishing, and sand blasting.
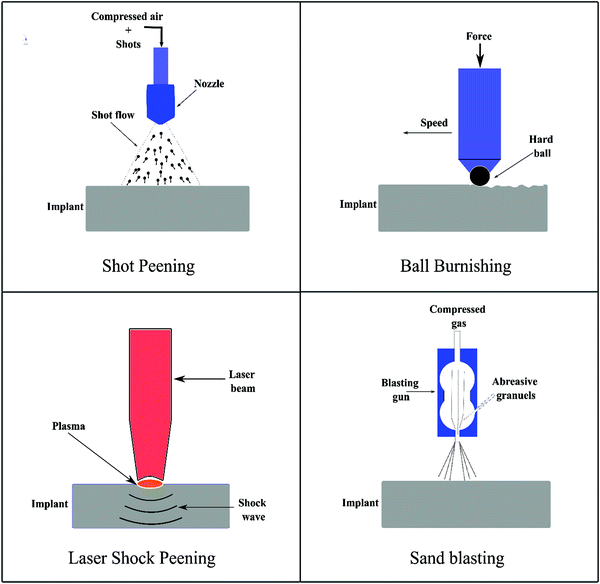 |
| Fig. 6 Schematic summarizing the various surface mechanical treatments deployed to modify the surface of degradable implants. | |
Shot peening (SP) is a cold surface work hardening process that introduces near-surface compressive residual stress into the material to prevent fatigue crack initiation and growth. For instance, Liu et al.,28 Wagner et al.,226 and Fouad et al.227 have applied SP on Mg-based alloys, e.g., Mg–10Gd–3Y, AZ31 and AZ80, and ZK60 alloys, and reported that the surface modifications with SP generated effective compressive stresses which shifted the fatigue crack nucleation site from the surface to subsurface regions, thus improving the overall fatigue strength and corrosion resistance. Mhaede et al.159 applied SP with ceramic shots (Z850, ∅ 850 μm) on the AZ31 magnesium alloy followed by the electrodeposition of a calcium phosphate coating, e.g., DCPD. The SP + DCPD coated sample enabled better coating adhesion and exhibited a higher corrosion resistance as compared to the ground + DCPD coated sample. This clearly indicates the impact of SP in combination with the coating in controlling the corrosion resistance of Mg-based alloys.
Ball burnishing as a controlled process is shown to increase the corrosion resistance and mechanical surface integrity of Mg-based alloys.30,228 Salahshoor and Guo27,229 studied the process mechanics of ball burnishing and low plasticity burnishing and investigated the effect of different process parameters on improving the corrosion resistance and the mechanical surface integrity of Mg–Ca alloys. Unlike low plasticity burnishing, severe plasticity burnishing integrated with cryogenic cooling has been employed to enhance the corrosion resistance of Mg–Al–Zn alloys,230 and the results were compared with the performance from the traditional grinding process. Cryogenic burnishing produced a fine-grained microstructure at a relatively larger depth, inducing severe compressive stress as well as generating a fine surface finish. Hydrogen evolution tests in NaCl solutions suggested that cryogenic burnishing reduced the corrosion rate by almost 50% as compared to the ground-only sample. Recently, Uddin et al.231 reported a synergistic approach combining burnishing and HA coating on AZ31B alloys, demonstrating that burnishing increased the surface activation energy enabling the growth of HA coating, and as a result, the corrosion resistance of the burnished + HA sample increased significantly without showing any negative response to immune cells as presented in Fig. 7.
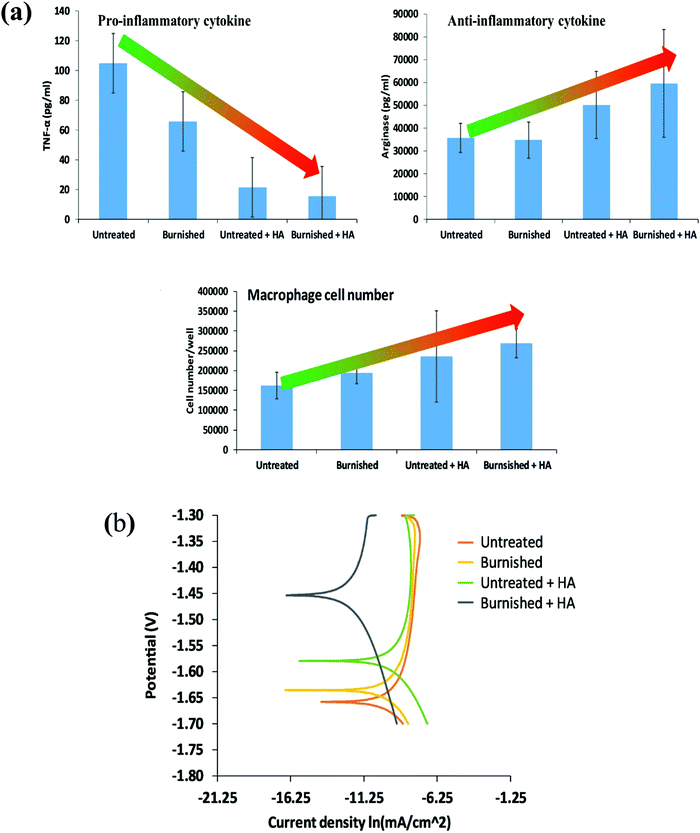 |
| Fig. 7 (a) Immune cell response to various conditions such as untreated, burnished, and electrodeposited HA on the untreated and burnished surface and (b) potentiodynamic polarization curves of different samples [Reprinted with permission from ref. 231. Copyright 2021 Elsevier]. | |
Sealy et al.232 used the laser shock peening (LSP) method to impart compressive residual stress to the Mg–Ca alloy and found that high compressive residual stress has great potential to slow down the corrosion rates. Zhang et al.29 applied LSP on AZ31B Mg alloys and demonstrated that LSP not only improves the hardness, strength, wear, and fatigue performance but also significantly increases the corrosion resistance by releasing less amount of Mg ions and H2 gas as by-products. Recently, Liu et al.233 showed that LSP pre-treated AZ31B Mg alloys enabled more formation and adhesion of phosphate conversion coating, which eventually increased the corrosion resistance of the alloy. Similar results were reported for ZK60 Mg alloys with a 50% increase in corrosion resistance when modified by LSP compared to the untreated sample.234
Von der Höh et al.235 employed turning, cylinder threading, and sandblasting on Mg–0.8Ca alloy implants and investigated their effect on the in vivo degradation behavior of the alloy for a 3–6 month period. It was shown that turned and threaded ones have higher corrosion resistance than the sanded sample. In addition, after 3 months, the turned implants showed the best bone-implant integration as compared to sandblasted and thread surfaces. Using turning, Denkena and Lucas236 attempted to control the degradation rate of Mg–3.0Ca alloys via modifying the surface integrity, including surface roughness and residual stress. It was reported that higher cutting forces due to a low cutting speed induced higher and deeper compressive stress and hence decreased the corrosion rate, while a fine surface finish under the same conditions was observed to have a negligible impact.
6. Conclusion and future directions
The surface of a biomaterial plays a crucial role in determining the performance of the medical device as several physical and chemical events are associated with the surface. The surface characteristics drive the biological response to the biomaterial. Modifying the surface of the biomaterial with a bioactive coating, mechanical treatment, or even their combination can have profound effects on the success of the implant as they provide enhanced biocompatibility in vivo along with other biomechanical and electrochemical benefits. The bioactive ceramic-coated degradable implant gives synergistic properties of both the coating and implants. Some bioactive ceramics such as bioactive glass, titanium oxide, and calcium phosphate-based ceramics are well studied, and their application as a coating for implants is now well established in the scientific literature. However, even as some other ceramics have the ability to impart specific responses to implants, their utility has not yet been extensively analyzed. At the same time, surface mechanical treatment is a promising method to improve the bio-corrosion potential along with enhanced fatigue resistance and ultimate tensile strength without compromising biocompatibility. A few studies have successfully combined mechanical treatments with bioactive ceramic coating to demonstrate the synergistic effects. In this review, we compiled and critically described several bioactive ceramics that have been applied as coatings to modulate the performance of implants focusing on resorbable biomaterials. Some of the common deposition methodologies used to coat implant surfaces were also described. Furthermore, several surface mechanical processes used for Mg-based degradable alloys and their effects on the alloy properties were discussed in this review.
Most of the bioactive coatings and surface mechanical treatments are reported for modification of non-degradable biomaterials. It is well known that degradable implants offer several benefits over conventional non-degradable devices, and thus, studies on surface modifications of degradable implants are essential and need more attention in the future. Another challenge in this field is the need for developing coating methods for uniformly coating porous foams or 3D printed scaffolds. Several of the widely employed coating strategies that are optimal for flat substrates are not suitable for porous implants. Similarly, the main limitation of mechanical surface treatments is that it is often difficult to modify the implant components with complex geometric shapes. Also, excessive cold working via these processes may damage the surface texture and geometric and dimensional accuracy, resulting in a detrimental effect on surface integrity. Design of appropriate process parameters in relation to the type of degradable materials being treated is crucial to achieve the desired performance in terms of degradation and mechanical integrity.
The kinetics and rate of degradation of the biomaterial can change after the application of a bioactive ceramic coating. It primarily depends on the nature, uniformity, and thickness of the coating material and the size, shape, and implantation site. A mismatch between the degradation rate of the implant and rate of tissue regeneration can cause implant failure. Thus, there is a need to develop predictive mathematical models and tools to characterize the degradation for minimizing failures. In addition to the degradation rate, strategies to enhance the adhesion between the coating and the substrates also need to be addressed. Thus, the rapidly growing field of biodegradable implants can benefit immensely from the modification of the surface of degradable implants by the application of bioactive coatings or mechanical treatments, but the clinical success of such surface-modified implants will need sustained efforts to resolve several challenges in this field.
Conflicts of interest
There are no conflicts to declare.
Acknowledgements
Support from the Department of Science and Technology (DST), Government of India, (DST/NM/NB/2018/119(G)) is gratefully acknowledged.
References
- C. W. Kang and F. Z. Fang, Adv. Manuf., 2018, 6, 20–40 CrossRef CAS.
- R. Oriňaková, R. Gorejová, Z. Orságová Králová and A. Oriňak, Coatings, 2020, 10, 819 CrossRef.
- B. Sultankulov, D. Berillo, K. Sultankulova, T. Tokay and A. J. B. Saparov, Biomolecules, 2019, 9, 470 CrossRef CAS PubMed.
- F. Croisier and C. Jérôme, Eur. Polym. J., 2013, 49, 780–792 CrossRef CAS.
- R. Parenteau-Bareil, R. Gauvin and F. J. M. Berthod, Materials, 2010, 3, 1863–1887 CrossRef CAS.
- T. P. Nguyen, Q. V. Nguyen, V.-H. Nguyen, T.-H. Le, V. Q. N. Huynh, D.-V. N. Vo, Q. T. Trinh, S. Y. Kim and Q. V. J. P. Le, Polymers, 2019, 11, 1933 CrossRef CAS PubMed.
- R. P. Pawar, S. U. Tekale, S. U. Shisodia, J. T. Totre and A. J. Domb, Recent Pat. Regener. Med., 2014, 4, 40–51 CAS.
- G. Narayanan, V. N. Vernekar, E. L. Kuyinu and C. T. Laurencin, Adv. Drug Delivery Rev., 2016, 107, 247–276 CrossRef CAS PubMed.
- R. Kulkarni, K. Pani, C. Neuman and F. Leonard, Arch. Surg., 1966, 93, 839–843 CrossRef CAS PubMed.
- D. Mondal, M. Griffith and S. S. Venkatraman, Int. J. Polym. Mater. Polym. Biomater., 2016, 65, 255–265 CrossRef CAS.
- R. M. Mohamed and K. Yusoh, Adv. Mater. Res., 2016, 1134, 249–255 Search PubMed.
- A. Basu and A. J. Domb, Adv. Mater., 2018, 30, 1706815 CrossRef PubMed.
- J. Heller, B. Fritzinger, S. Ng and D. Penhale, J. Controlled Release, 1985, 1, 225–232 CrossRef CAS.
- Y. Chen, Z. Xu, C. Smith and J. Sankar, Acta Biomater., 2014, 10, 4561–4573 CrossRef CAS PubMed.
- S. Kamrani and C. Fleck, Biometals, 2019, 32, 185–193 CrossRef CAS PubMed.
- I. Pospíšilová and D. Vojtěch, Mater. Sci. Forum, 2014, 782, 457–460 Search PubMed.
- G. Katarivas Levy, J. Goldman and E. Aghion, Metals, 2017, 7, 402 CrossRef.
- M. Schinhammer, A. C. Hänzi, J. F. Löffler and P. J. Uggowitzer, Acta Biomater., 2010, 6, 1705–1713 CrossRef CAS PubMed.
- J. R. Jones, Acta Biomater., 2013, 9, 4457–4486 CrossRef CAS PubMed.
- N. Eliaz and N. Metoki, Materials, 2017, 10, 334 CrossRef PubMed.
- C. G. Ambrose and T. O. Clanton, Ann. Biomed. Eng., 2004, 32, 171–177 CrossRef PubMed.
- M. Prakasam, J. Locs, K. Salma-Ancane, D. Loca, A. Largeteau and L. Berzina-Cimdina, J. Funct. Biomater., 2017, 8, 44 CrossRef PubMed.
- G. Hofmann, Arch. Orthop. Trauma Surg., 1995, 114, 123–132 CrossRef CAS PubMed.
- R. Narayanan, S. Seshadri, T. Kwon and K. Kim, J. Biomed. Mater. Res., Part B, 2008, 85, 279–299 CrossRef CAS PubMed.
- J. S. Fernandes, P. Gentile, R. A. Pires, R. L. Reis and P. V. Hatton, Acta Biomater., 2017, 59, 2–11 CrossRef CAS PubMed.
- A. A. Gorustovich, J. A. Roether and A. R. Boccaccini, Tissue Eng., Part B, 2010, 16, 199–207 CrossRef CAS PubMed.
- M. Salahshoor and Y. Guo, Int. J. Adv. Manuf. Technol., 2013, 64, 133–144 CrossRef.
- W. Liu, J. Dong, P. Zhang, A. Korsunsky, X. Song and W. Ding, Mater. Sci. Eng., A, 2011, 528, 5935–5944 CrossRef CAS.
- R. Zhang, X. Zhou, H. Gao, S. Mankoci, Y. Liu, X. Sang, H. Qin, X. Hou, Z. Ren and G. L. Doll, Surf. Coat. Technol., 2018, 339, 48–56 CrossRef CAS.
- M. Uddin, C. Hall and P. Murphy, Sci. Technol. Adv. Mater., 2015, 16, 053501 CrossRef CAS PubMed.
- C. Li, C. Guo, V. Fitzpatrick, A. Ibrahim, M. J. Zwierstra, P. Hanna, A. Lechtig, A. Nazarian, S. J. Lin and D. L. Kaplan, Nat. Rev. Mater., 2020, 5, 61–81 CrossRef.
- M. Peuster, C. Hesse, T. Schloo, C. Fink, P. Beerbaum and C. von Schnakenburg, Biomaterials, 2006, 27, 4955–4962 CrossRef CAS PubMed.
- M. Ulum, A. Arafat, D. Noviana, A. Yusop, A. Nasution, M. A. Kadir and H. Hermawan, Mater. Sci. Eng., C, 2014, 36, 336–344 CrossRef CAS PubMed.
- M. Sikora-Jasinska, P. Chevallier, S. Turgeon, C. Paternoster, E. Mostaed, M. Vedani and D. Mantovani, RSC Adv., 2018, 8, 9627–9639 RSC.
- B. Liu, Y. Zheng and L. Ruan, Mater. Lett., 2011, 65, 540–543 CrossRef CAS.
- H. Li, Y. Zheng and L. Qin, Prog. Nat. Sci.: Mater. Int., 2014, 24, 414–422 CrossRef CAS.
- M. Schinhammer, P. Steiger, F. Moszner, J. F. Löffler and P. J. Uggowitzer, Mater. Sci. Eng., C, 2013, 33, 1882–1893 CrossRef CAS PubMed.
- H. Hermawan, H. Alamdari, D. Mantovani and D. Dube, Powder Metall., 2008, 51, 38–45 CrossRef CAS.
- D.-T. Chou, D. Wells, D. Hong, B. Lee, H. Kuhn and P. N. Kumta, Acta Biomater., 2013, 9, 8593–8603 CrossRef CAS PubMed.
- M. Moravej, S. Amira, F. Prima, A. Rahem, M. Fiset and D. Mantovani, Mater. Sci. Eng., B, 2011, 176, 1812–1822 CrossRef CAS.
- J. Cheng and Y. Zheng, J. Biomed. Mater. Res., Part B, 2013, 101, 485–497 CrossRef CAS PubMed.
- Q. Feng, D. Zhang, C. Xin, X. Liu, W. Lin, W. Zhang, S. Chen and K. Sun, J. Mater. Sci.: Mater. Med., 2013, 24, 713–724 CrossRef CAS PubMed.
- F. Witte, Acta Biomater., 2010, 6, 1680–1692 CrossRef CAS PubMed.
- Y.-W. Song, D.-Y. Shan, R.-S. Chen and E.-H. Han, Surf. Eng., 2007, 23, 334–338 CrossRef.
- P.-R. Cha, H.-S. Han, G.-F. Yang, Y.-C. Kim, K.-H. Hong, S.-C. Lee, J.-Y. Jung, J.-P. Ahn, Y.-Y. Kim and S.-Y. Cho, Sci. Rep., 2013, 3, 1–6 Search PubMed.
- K. F. Farraro, K. E. Kim, S. L. Woo, J. R. Flowers and M. B. McCullough, J. Biomech., 2014, 47, 1979–1986 CrossRef PubMed.
- Z. Li, X. Gu, S. Lou and Y. Zheng, Biomaterials, 2008, 29, 1329–1344 CrossRef CAS PubMed.
- M. Behera, M. Rajput, S. Acharya, N. Nadammal, S. Suwas and K. Chatterjee, Biomed. Mater., 2021, 16, 044109 CrossRef CAS PubMed.
- S. Zhang, X. Zhang, C. Zhao, J. Li, Y. Song, C. Xie, H. Tao, Y. Zhang, Y. He and Y. Jiang, Acta Biomater., 2010, 6, 626–640 CrossRef CAS PubMed.
- R. Song, M. Murphy, C. Li, K. Ting, C. Soo and Z. Zheng, Drug Des., Dev. Ther., 2018, 12, 3117 CrossRef CAS PubMed.
- H. Fang, C. Wang, S. Zhou, Z. Zheng, T. Lu, G. Li, Y. Tian and T. Suga, Corros. Sci., 2020, 168, 108466 CrossRef CAS.
- H. Fang, C. Wang, S. Zhou, G. Li, Y. Tian and T. Suga, J. Magnesium Alloys, 2021, 9, 1578–1594 CrossRef CAS.
- M. Alizadeh-Osgouei, Y. Li and C. Wen, Bioact. Mater., 2019, 4, 22–36 CrossRef PubMed.
- F. Haghighat and S. A. H. Ravandi, Fibers Polym., 2014, 15, 71–77 CrossRef CAS.
- S. I. Jeong, B.-S. Kim, S. W. Kang, J. H. Kwon, Y. M. Lee, S. H. Kim and Y. H. Kim, Biomaterials, 2004, 25, 5939–5946 CrossRef CAS PubMed.
- O. S. Manoukian, M. R. Arul, N. Sardashti, T. Stedman, R. James, S. Rudraiah and S. G. Kumbar, J. Appl. Polym. Sci., 2018, 135, 46068 CrossRef PubMed.
- Q. Dasgupta, G. Madras and K. Chatterjee, Int. Mater. Rev., 2019, 64, 288–309 CrossRef CAS.
- S. Nilawar, Q. Dasgupta, G. Madras and K. Chatterjee, Emergent Mater., 2019, 2, 153–168 CrossRef CAS.
- J. Natarajan, S. Rattan, U. Singh, G. Madras and K. Chatterjee, Ind. Eng. Chem. Res., 2014, 53, 7891–7901 CrossRef CAS.
- A. Banerjee, K. Chatterjee and G. Madras, Mater. Sci. Technol., 2014, 30, 567–573 CrossRef CAS.
- J. Pasquet, Y. Chevalier, J. Pelletier, E. Couval, D. Bouvier and M.-A. Bolzinger, Colloids Surf., A, 2014, 457, 263–274 CrossRef CAS.
- Y. Li, Y. Yang, R. L. Yun’an Qing, X. Tang, D. Guo and Y. Qin, Int. J. Nanomed., 2020, 15, 6247 CrossRef CAS PubMed.
- K. S. Suh, Y. S. Lee, S. H. Seo, Y. S. Kim and E. M. Choi, Biol. Trace Elem. Res., 2013, 155, 287–294 CrossRef CAS PubMed.
- R. Zhang, X. Liu, Z. Xiong, Q. Huang, X. Yang, H. Yan, J. Ma, Q. Feng and Z. Shen, Artif. Cells, Nanomed., Biotechnol., 2018, 46, 1123–1130 CrossRef CAS PubMed.
- G. Bisht and S. Rayamajhi, Nanobiomedicine, 2016, 3, 3–9 CrossRef PubMed.
- P. Nagajyothi, S. J. Cha, I. J. Yang, T. Sreekanth, K. J. Kim and H. M. Shin, J. Photochem. Photobiol., B, 2015, 146, 10–17 CrossRef CAS PubMed.
- Y. S. Cho, H.-K. Kim, M.-S. Ghim, M. W. Hong, Y. Y. Kim and Y.-S. Cho, Polymers, 2020, 12, 2193 CrossRef CAS PubMed.
- C. Venkatesh, M. Laurenti, M. Bandeira, E. Lanzagorta, L. Lucherini, V. Cauda and D. M. Devine, Coatings, 2020, 10, 1002 CrossRef CAS.
- A. Kania, W. Pilarczyk and R. Babilas, Acta Phys. Pol., A, 2018, 133, 222–224 CrossRef CAS.
- H. R. Bakhsheshi-Rad, E. Hamzah, A. F. Ismail, M. Aziz, M. Kasiri-Asgarani, H. Ghayour, M. Razzaghi and Z. Hadisi, Mater. Corros., 2017, 68, 1228–1236 CrossRef CAS.
- H. M. Mousa, A. Abdal-Hay, M. Bartnikowski, I. M. Mohamed, A. S. Yasin, S. O. Ivanovski, C. H. Park and C. S. Kim, ACS Biomater. Sci. Eng., 2018, 4, 2169–2180 CrossRef CAS PubMed.
- H. R. Bakhsheshi-Rad, E. Hamzah, W. S. Ying, M. Razzaghi, S. Sharif, A. F. Ismail and F. Berto, Materials, 2021, 14, 1930 CrossRef CAS PubMed.
- Y. Guo, S. Jia, L. Qiao, Y. Su, R. Gu, G. Li and J. Lian, Colloids Surf., B, 2020, 194, 111186 CrossRef CAS PubMed.
- I. Clarke, M. Manaka, D. Green, P. Williams, G. Pezzotti, Y.-H. Kim, M. Ries, N. Sugano, L. Sedel and C. Delauney, J. Bone Jt. Surg., 2003, 85, 73–84 CrossRef PubMed.
- A. Apratim, P. Eachempati, K. K. K. Salian, V. Singh, S. Chhabra and C. Shah, J. Int. Soc. Prev. Community Dent., 2015, 5, 147 CrossRef PubMed.
- N. P. Thomas, N. Tran, P. A. Tran, J. L. Walters, J. D. Jarrell, R. A. Hayda and C. T. Born, J. Mater. Sci.: Mater. Med., 2014, 25, 347–354 CrossRef CAS PubMed.
- A. Śmieszek, J. Szydlarska, A. Mucha, M. Chrapiec and K. Marycz, J. Biomater. Appl., 2017, 32, 570–586 CrossRef PubMed.
- A. Seweryn, A. Pielok, K. Lawniczak-Jablonska, R. Pietruszka, K. Marcinkowska, M. Sikora, B. S. Witkowski, M. Godlewski, K. Marycz and A. Smieszek, Int. J. Nanomed., 2020, 15, 1595 CrossRef CAS PubMed.
- M. R. Goudarzi, M. Bagherzadeh, M. Fazilati, F. Riahi, H. Salavati and S. S. Esfahani, IET Nanobiotechnol., 2019, 13, 449–455 CrossRef PubMed.
- R. Karimian, M. G. Mehrabani, B. Mehramuz, K. Ganbarov, L. Ejlali, A. Tanomand, F. S. Kamounah, M. A. Rezaee, M. Yousefi and E. Sheykhsaran, Adv. Pharm. Bull., 2020, 10, 577 CrossRef PubMed.
- K. Huang, X. Lin, C. Xie and T. M. Yue, Bull. Mater. Sci., 2013, 36, 99–105 CrossRef CAS.
- Q. Yang, W. Yuan, X. Liu, Y. Zheng, Z. Cui, X. Yang, H. Pan and S. Wu, Acta Biomater., 2017, 58, 515–526 CrossRef CAS PubMed.
- H. K. Tsou and P. Y. Hsieh, Appl. Titanium Dioxide, 2017, 103–122 CAS.
- S. Feng, F. Zhang, S. Ahmed and Y. Liu, Coatings, 2019, 9, 525 CrossRef CAS.
- H. Matsuno, R. Matsuyama, A. Yamamoto and K. Tanaka, Polym. J., 2015, 47, 505–512 CrossRef CAS.
- W. Xia, C. Lindahl, J. Lausmaa and H. Engqvist, Adv. Biomimetics, 2011, 20, 429–452 Search PubMed.
- S. Hou, W. Yu, Z. Yang, Y. Li, L. Yang and S. Lang, Coatings, 2020, 10, 999 CrossRef CAS.
- V. Hernández-Montes, C. P. Betancur-Henao and J. F. Santa-Marín, Dyna, 2017, 84, 261–270 CrossRef.
- L. White, Y. Koo, Y. Yun and J. Sankar, J. Nanomater., 2013, 2013, 319437 Search PubMed.
- H.-K. Tsou, M.-H. Chi, Y.-W. Hung, C.-J. Chung and J.-L. He, BioMed Res. Int., 2015, 2015, 328943 Search PubMed.
- N. Anicic, M. Vukomanovic and D. Suvorov, RSC Adv., 2017, 7, 38647–38658 RSC.
- P. R. P. Suma, R. V. Nair, W. Paul and R. S. Jayasree, Mater. Lett., 2020, 269, 127673 CrossRef CAS.
- A. Menazea, M. H. El-Newehy, B. M. Thamer and M. E. El-Naggar, J. Mol. Struct., 2021, 1225, 129163 CrossRef CAS.
- S. R. S. R. Kachupalli, M. V. Prasanth, K. N. Murthy, G. Subbaih, H. Janarthanam, S. K. Jayapalan and M. Sangeetha, AIP Conf. Proc., 2020, 2311, 080002 CrossRef CAS.
- Z. Wang, Y. Ma and Y. Wang, Metals, 2020, 10, 1146 CrossRef CAS.
- S. Meghana, P. Kabra, S. Chakraborty and N. Padmavathy, RSC Adv., 2015, 5, 12293–12299 RSC.
- A. Muñoz-Escobar, Á. D. J. Ruíz-Baltazar and S. Y. Reyes-López, Dose-Response, 2019, 17, 1559325819869502 CrossRef PubMed.
- A. Muñoz-Escobar and S. Y. Reyes-López, PLoS One, 2020, 15, e0228864 CrossRef PubMed.
- J. Balcucho, D. M. Narváez and J. L. Castro-Mayorga, Nanomaterials, 2020, 10, 1692 CrossRef CAS PubMed.
- A. Badaraev, A. Nemoykina, E. N. Bolbasov and S. I. Tverdokhlebov, Resour.-Effic. Technol., 2017, 3, 204–211 Search PubMed.
- H. Xie and Y. J. Kang, Curr. Med. Chem., 2009, 16, 1304–1314 CrossRef CAS PubMed.
- C. Stähli, M. James-Bhasin, A. Hoppe, A. R. Boccaccini and S. N. Nazhat, Acta Biomater., 2015, 19, 15–22 CrossRef PubMed.
- L. R. Rivera, A. Cochis, S. Biser, E. Canciani, S. Ferraris, L. Rimondini and A. R. Boccaccini, Bioact. Mater., 2021, 6, 1479–1490 CrossRef CAS PubMed.
- Z. Lin, Y. Cao, J. Zou, F. Zhu, Y. Gao, X. Zheng, H. Wang, T. Zhang and T. Wu, Mater. Sci. Eng., C, 2020, 114, 111032 CrossRef CAS PubMed.
- L. Jaidev, S. Kumar and K. Chatterjee, Colloids Surf., B, 2017, 159, 293–302 CrossRef CAS PubMed.
- M. Arakha, S. Pal, D. Samantarrai, T. K. Panigrahi, B. C. Mallick, K. Pramanik, B. Mallick and S. Jha, Sci. Rep., 2015, 5, 1–12 Search PubMed.
-
S. Pal, Antibacterial properties of iron oxide nanoparticles, MS thesis, NIT, Rourkela, 2014 Search PubMed.
- P. Mohan and R. Mala, Mater. Res. Express, 2019, 6, 115077 CrossRef.
- P. Nehra, R. Chauhan, N. Garg and K. Verma, Br. J. Biomed. Sci., 2018, 75, 13–18 CrossRef CAS PubMed.
- M. Thukkaram, S. Sitaram and G. Subbiahdoss, Int. J. Biomater., 2014, 2014, 716080 Search PubMed.
- S. A. Rovers, R. Hoogenboom, M. F. Kemmere and J. T. Keurentjes, Soft Matter, 2012, 8, 1623–1627 RSC.
- C. Xu, O. U. Akakuru, J. Zheng and A. Wu, Front. Bioeng. Biotechnol., 2019, 7, 141 CrossRef PubMed.
- T.-J. Yu, P.-H. Li, T.-W. Tseng and Y.-C. Chen, Nanomedicine, 2011, 6, 1353–1363 CrossRef CAS PubMed.
- T. Suzuki, I. Kosacki, H. U. Anderson and P. Colomban, J. Am. Ceram. Soc., 2001, 84, 2007–2014 CrossRef CAS.
- Z. Hu, S. Haneklaus, G. Sparovek and E. Schnug, Commun. Soil Sci. Plant Anal., 2006, 37, 1381–1420 CrossRef CAS.
- R. Di Monte and J. Kašpar, J. Mater. Chem., 2005, 15, 633–648 RSC.
- C. Laberty-Robert, J. W. Long, K. A. Pettigrew, R. M. Stroud and D. R. Rolison, Adv. Mater., 2007, 19, 1734–1739 CrossRef CAS.
- F. R. Cassee, E. C. Van Balen, C. Singh, D. Green, H. Muijser, J. Weinstein and K. Dreher, Crit. Rev. Toxicol., 2011, 41, 213–229 CrossRef PubMed.
- C. Korsvik, S. Patil, S. Seal and W. T. Self, Chem. Commun., 2007, 1056–1058 RSC.
- I. A. P. Farias, C. C. L. d. Santos and F. C. Sampaio, BioMed Res. Int., 2018, 2018, 1923606 Search PubMed.
- I. A. Farias, C. C. Santos, A. L. Xavier, T. M. Batista, Y. M. Nascimento, J. M. Nunes, P. M. Silva, R. A. Menezes-Junior, J. M. Ferreira and E. O. Lima, Arabian J. Chem., 2021, 14, 102888 CrossRef CAS.
- S. Das, S. Singh, J. M. Dowding, S. Oommen, A. Kumar, T. X. Sayle, S. Saraf, C. R. Patra, N. E. Vlahakis and D. C. Sayle, Biomaterials, 2012, 33, 7746–7755 CrossRef CAS PubMed.
- R. Augustine, Y. B. Dalvi, P. Dan, N. George, D. Helle, R. Varghese, S. Thomas, P. Menu and N. Sandhyarani, ACS Biomater. Sci. Eng., 2018, 4, 4338–4353 CrossRef CAS PubMed.
- S. D. Purohit, H. Singh, R. Bhaskar, I. Yadav, C.-F. Chou, M. K. Gupta and N. C. Mishra, Mater. Sci. Eng., C, 2020, 116, 111111 CrossRef CAS PubMed.
- A. Jain, M. Behera, C. Mahapatra, N. R. Sundaresan and K. Chatterjee, Mater. Sci. Eng., C, 2021, 118, 111416 CrossRef CAS PubMed.
- A. P. Loperena, I. L. Lehr and S. B. Saidman, J. Magnesium Alloys, 2016, 4, 278–285 CrossRef CAS.
- L. Lingjie, L. Jinglei, Y. Shenghai, T. Yujing, Q. Jiang and P. Fusheng, J. Rare Earths, 2008, 26, 383–387 CrossRef.
- X. Li, M. Qi, X. Sun, M. D. Weir, F. R. Tay, T. W. Oates, B. Dong, Y. Zhou, L. Wang and H. H. Xu, Acta Biomater., 2019, 94, 627–643 CrossRef CAS PubMed.
- C. Wu, L. Xia, P. Han, L. Mao, J. Wang, D. Zhai, B. Fang, J. Chang and Y. Xiao, ACS Appl. Mater. Interfaces, 2016, 8, 11342–11354 CrossRef CAS PubMed.
- M. Luo, M. Wang, W. Niu, M. Chen, W. Cheng, L. Zhang, C. Xie, Y. Wang, Y. Guo and T. Leng, Chem. Eng. J., 2021, 412, 128471 CrossRef CAS.
- H. Nosrati, R. A. Khouy, A. Nosrati, M. Khodaei, M. Banitalebi-Dehkordi, K. Ashrafi-Dehkordi, S. Sanami and Z. Alizadeh, J. Nanobiotechnol., 2021, 19, 1–21 CrossRef PubMed.
- C. R. Patra, R. Bhattacharya, S. Patra, N. E. Vlahakis, A. Gabashvili, Y. Koltypin, A. Gedanken, P. Mukherjee and D. Mukhopadhyay, Adv. Mater., 2008, 20, 753–756 CrossRef CAS.
- R. Augustine, S. K. Nethi, N. Kalarikkal, S. Thomas and C. R. Patra, J. Mater. Chem. B, 2017, 5, 4660–4672 RSC.
- L. L. Hench, J. Mater. Sci.: Mater. Med., 2006, 17, 967–978 CrossRef CAS PubMed.
- R. Sergi, D. Bellucci and V. Cannillo, Coatings, 2020, 10, 757 CrossRef CAS.
- A. Wren, A. Coughlan, P. Hassanzadeh and M. Towler, J. Mater. Sci.: Mater. Med., 2012, 23, 1331–1341 CrossRef CAS PubMed.
- A. S. Hameed, R. J. Al-Warid and I. A. Obaid, J. Univ. Babylon, 2016, 24, 1395–1400 Search PubMed.
- N. O. Joy-anne, Y. Su, X. Lu, P.-H. Kuo, J. Du and D. Zhu, Bioact. Mater., 2019, 4, 261–270 CrossRef PubMed.
- N. Lakhkar, E. Abou Neel, V. Salih and J. Knowles, J. Mater. Sci.: Mater. Med., 2009, 20, 1339–1346 CrossRef CAS PubMed.
- M. O’donnell and R. Hill, Acta Biomater., 2010, 6, 2382–2385 CrossRef PubMed.
- J. Blaker, S. Nazhat and A. Boccaccini, Biomaterials, 2004, 25, 1319–1329 CrossRef CAS PubMed.
- V. Yadav, M. Sankar and L. Pandey, J. Magnesium Alloys, 2020, 8, 999–1015 CrossRef CAS.
- J. Roether, A. R. Boccaccini, L. Hench, V. Maquet, S. Gautier and R. Jérôme, Biomaterials, 2002, 23, 3871–3878 CrossRef CAS PubMed.
- M. Zhu, L. Zhang, Q. He, J. Zhao, G. Limin and J. Shi, J. Mater. Chem., 2011, 21, 1064–1072 RSC.
- S. Bansal, V. Chauhan, S. Sharma, R. Maheshwari, A. Juyal and S. Raghuvanshi, Indian J. Orthop., 2009, 43, 234 CrossRef PubMed.
- Y. Shi, M. Qi, Y. Chen and P. J. M. L. Shi, Mater. Lett., 2011, 65, 2201–2204 CrossRef CAS.
- K. Chatterjee, L. Sun, L. C. Chow, M. F. Young and C. G. J. B. Simon Jr, Biomaterials, 2011, 32, 1361–1369 CrossRef CAS PubMed.
- X. Ge, Y. Leng, C. Bao, S. L. Xu, R. Wang and F. Ren, J. Biomed. Mater. Res., Part A, 2010, 95, 588–599 CrossRef PubMed.
- M. Riaz, R. Zia, A. Ijaz, T. Hussain, M. Mohsin and A. Malik, Mater. Sci. Eng., C, 2018, 90, 308–313 CrossRef CAS PubMed.
- C. S. Ciobanu, S. L. Iconaru, M. C. Chifiriuc, A. Costescu, P. Le Coustumer and D. Predoi, BioMed Res. Int., 2013, 2013, 916218 Search PubMed.
- E. A. Ofudje, A. I. Adeogun, M. A. Idowu and S. O. Kareem, Heliyon, 2019, 5, e01716 CrossRef PubMed.
- D. Predoi, S. L. Iconaru, M. V. Predoi, M. Motelica-Heino, R. Guegan and N. Buton, Nanomaterials, 2019, 9, 515 CrossRef CAS PubMed.
- O. Geuli, N. Metoki, T. Zada, M. Reches, N. Eliaz and D. Mandler, J. Mater. Chem. B, 2017, 5, 7819–7830 RSC.
- M. Stigter, J. Bezemer, K. De Groot and P. Layrolle, J. Controlled Release, 2004, 99, 127–137 CrossRef CAS PubMed.
- S. B. Sulaiman, T. K. Keong, C. H. Cheng, A. B. Saim and R. B. H. Idrus, Indian J. Med. Res., 2013, 137, 1093 Search PubMed.
- S. Shadanbaz and G. J. Dias, Acta Biomater., 2012, 8, 20–30 CrossRef CAS PubMed.
- M. Rahman, Y. Li and C. Wen, J. Magnesium Alloys, 2020, 8, 929–943 CrossRef CAS.
- M. A. F. Zaludin, Z. A. Z. Jamal, S. B. Jamaludin and M. N. Derman, AIP Conf. Proc., 2016, 1756, 080001 CrossRef.
- M. Mhaede, F. Pastorek and B. Hadzima, Mater. Sci. Eng., C, 2014, 39, 330–335 CrossRef CAS PubMed.
- C. Guo, X. Guo, N. Cai and Y. Dong, Mater. Lett., 2012, 74, 197–199 CrossRef CAS.
- H. Deplaine, M. Lebourg, P. Ripalda, A. Vidaurre, P. Sanz-Ramos, G. Mora, F. Prósper, I. Ochoa, M. Doblaré and J. L. Gómez Ribelles, J. Biomed. Mater. Res., Part B, 2013, 101, 173–186 CrossRef CAS PubMed.
- J. Araujo, A. Martins, I. Leonor, E. D. Pinho, R. Reis and N. Neves, J. Biomater. Sci., Polym. Ed., 2008, 19, 1261–1278 CrossRef CAS PubMed.
- E. Prosecká, M. Buzgo, M. Rampichová, T. Kocourek, P. Kochová, L. Vysloužilová, D. Tvrdík, M. Jelínek, D. Lukáš and E. Amler, J. Biomed. Biotechnol., 2012, 2012, 428503 Search PubMed.
- M. Schneider, C. Günter and A. Taubert, Polymers, 2018, 10, 275 CrossRef PubMed.
- J. Jaroszewicz, J. Idaszek, E. Choinska, K. Szlazak, A. Hyc, A. Osiecka-Iwan, W. Swieszkowski and S. Moskalewski, Mater. Sci. Eng., C, 2019, 96, 319–328 CrossRef CAS PubMed.
- C. Choong, J. Triffitt and Z. Cui, Food Bioprod. Process., 2004, 82, 117–125 CrossRef CAS.
- W. Chen, L. Nichols, F. Brinkley, K. Bohna, W. Tian, M. W. Priddy and L. B. Priddy, Mater. Sci. Eng., C, 2021, 120, 111686 CrossRef CAS PubMed.
- L. Jaidev and K. Chatterjee, Mater. Des., 2019, 161, 44–54 CrossRef CAS.
- P. S. Poh, D. W. Hutmacher, B. M. Holzapfel, A. K. Solanki, M. M. Stevens and M. A. Woodruff, Acta Biomater., 2016, 30, 319–333 CrossRef CAS PubMed.
- C. Auclair-Daigle, M. Bureau, J. G. Legoux and L. H. Yahia, J. Biomed. Mater. Res., Part A, 2005, 73, 398–408 CrossRef CAS PubMed.
- S. Ahangarani, A. Sabour Rouhaghadam and M. Azadi, Int. J. Eng., 2016, 29, 677–687 Search PubMed.
- M. Brama, N. Rhodes, J. Hunt, A. Ricci, R. Teghil, S. Migliaccio, C. Della Rocca, S. Leccisotti, A. Lioi and M. Scandurra, Biomaterials, 2007, 28, 595–608 CrossRef CAS PubMed.
- S. P. Devaneyan, D. P. Pushpanathan, T. Senthilvelan and R. Ganesh, Mater. Today: Proc., 2018, 5, 11491–11497 CAS.
- A. Vladescu, V. Pruna, S. Kulesza, V. Braic, I. Titorencu, M. Bramowicz, A. Gozdziejewska, A. Parau, C. M. Cotrut and I. Pana, Ceram. Int., 2019, 45, 1710–1723 CrossRef CAS.
- D. P. Pushpanathan, N. Alagumurthi and S. P. Devaneyan, Mater. Today: Proc., 2020, 22, 2565–2572 Search PubMed.
- C. Fares, S.-M. Hsu, M. Xian, X. Xia, F. Ren, J. J. Mecholsky, L. Gonzaga and J. Esquivel-Upshaw, Materials, 2020, 13, 3321 CrossRef CAS PubMed.
- M. Li, Y. Cheng, Y. Zheng, X. Zhang, T. Xi and S. Wei, Appl. Surf. Sci., 2012, 258, 3074–3081 CrossRef CAS.
- M. Tacikowski, M. Betiuk, K. Cymerman, M. Pisarek, I. Pokorska and T. Wierzchoń, J. Magnesium Alloys, 2014, 2, 265–273 CrossRef CAS.
- M. Tacikowski, J. Morgiel, M. Banaszek, K. Cymerman and T. Wierzchon, Trans. Nonferrous Met. Soc. China, 2014, 24, 2767–2775 CrossRef CAS.
- H. Altun and H. Sinici, Mater. Charact., 2008, 59, 266–270 CrossRef CAS.
- M. Annunziata, A. Oliva, M. A. Basile, M. Giordano, N. Mazzola, A. Rizzo, A. Lanza and L. Guida, J. Dent., 2011, 39, 720–728 CrossRef CAS PubMed.
- Y. S. A. Jabbari, J. Fehrman, A. C. Barnes, A. M. Zapf, S. Zinelis and D. W. Berzins, Coatings, 2012, 2, 160–178 CrossRef.
- S. J. Gobbi, V. J. Gobbi, G. Reinke and Y. Rocha, Biomed. J. Sci. Tech. Res., 2019, 16, 1–3 Search PubMed.
- S. Takaoka, T. Yamaguchi, S. Yano, M. Yamauchi and T. Sugimoto, Horm. Metab. Res., 2010, 42, 627–631 CrossRef CAS PubMed.
- R. Baron and Y. Tsouderos, Eur. J. Pharmacol., 2002, 450, 11–17 CrossRef CAS PubMed.
- S. Kargozar, M. Montazerian, E. Fiume and F. Baino, Front. Bioeng. Biotechnol., 2019, 7, 161 CrossRef PubMed.
- Z. Xu, H. Lu, J. Lu, C. Lv, X. Zhao and G. Wang, RSC Adv., 2018, 8, 3051–3060 RSC.
- Q. Zhang, Y. Ji, W. Zheng, M. Yan, D. Wang, M. Li, J. Chen, X. Yan, Q. Zhang and X. Yuan, J. Nanomater., 2020, 2020, 1257646 Search PubMed.
- S. R. K. Meka, S. Jain and K. Chatterjee, Colloids Surf., B, 2016, 146, 649–656 CrossRef CAS PubMed.
- P. Amaravathy, S. Sowndarya, S. Sathyanarayanan and N. Rajendran, Surf. Coat. Technol., 2014, 244, 131–141 CrossRef CAS.
- J. H. Lopes, L. P. Souza, J. A. Domingues, F. V. Ferreira, M. de Alencar Hausen, J. A. Camilli, R. A. Martin, E. A. de Rezende Duek, I. O. Mazali and C. A. Bertran, J. Biomed. Mater. Res., Part B, 2020, 108, 1372–1387 CrossRef CAS PubMed.
- M. Mazur, M. Kalisz, D. Wojcieszak, M. Grobelny, P. Mazur, D. Kaczmarek and J. Domaradzki, Mater. Sci. Eng., C, 2015, 47, 211–221 CrossRef CAS PubMed.
- D. J. Hickey, D. Muthusamy and T. J. Webster, J. Biomed. Mater. Res., Part A, 2017, 105, 3136–3147 CrossRef CAS PubMed.
- D. J. Hickey, B. Ercan, L. Sun and T. J. Webster, Acta Biomater., 2015, 14, 175–184 CrossRef CAS PubMed.
- M. Šupová, Ceram. Int., 2015, 41, 9203–9231 CrossRef.
- Q. Zhao, L. Yi, L. Jiang, Y. Ma, H. Lin and J. Dong, Nanomedicine, 2019, 16, 149–161 CrossRef CAS PubMed.
- Q. Wu, J. Li, W. Zhang, H. Qian, W. She, H. Pan, J. Wen, X. Zhang, X. Liu and X. Jiang, J. Mater. Chem. B, 2014, 2, 6738–6748 RSC.
- S. Liu, K. Li, T. Hu, D. Shao, S. Huang, Y. Xie and X. Zheng, Colloids Surf., B, 2021, 202, 111666 CrossRef CAS PubMed.
- Y. Xu, T. Wang, Y. Guo, G. Li and J. Lian, Langmuir, 2020, 36, 13937–13948 CrossRef CAS PubMed.
- C. Brinker, A. Hurd, P. Schunk, G. Frye and C. Ashley, J. Non-Cryst. Solids, 1992, 147, 424–436 CrossRef.
- K. Ishikawa and A. Kareiva, Chemija, 2020, 31, 25–41 CrossRef CAS.
- H. Hornberger, S. Virtanen and A. R. Boccaccini, Acta Biomater., 2012, 8, 2442–2455 CrossRef CAS PubMed.
- J. E. López Herrera, V. Hernández Montes, C. P. Betancur Henao, J. F. Santa Marín and R. Buitrago Sierra, INGE CUC, 2018, 14, 45–54 CrossRef.
- A. R. Boccaccini, J. A. Roether, B. J. Thomas, M. S. Shaffer, E. Chavez, E. Stoll and E. J. Minay, J. Ceram.
Soc. Jpn., 2006, 114, 1–14 CrossRef CAS.
- D. Zhitomirsky, J. Roether, A. Boccaccini and I. Zhitomirsky, J. Mater. Process. Technol., 2009, 209, 1853–1860 CrossRef CAS.
- M. Đošić, S. Eraković, A. Janković, M. Vukašinović-Sekulić, I. Z. Matić, J. Stojanović, K. Y. Rhee, V. Mišković-Stanković and S.-J. Park, J. Ind. Eng. Chem., 2017, 47, 336–347 CrossRef.
- X. Pang and I. Zhitomirsky, Int. J. Nanosci., 2005, 4, 409–418 CrossRef CAS.
- T. G. P. Galindo, T. Kataoka, S. Fujii, M. Okuda and M. Tagaya, Colloid Interface Sci. Commun., 2016, 10, 15–19 CrossRef.
- R. M. Kumar, K. K. Kuntal, S. Singh, P. Gupta, B. Bhushan, P. Gopinath and D. Lahiri, Surf. Coat. Technol., 2016, 287, 82–92 CrossRef.
- S. Kaabi Falahieh Asl, S. Nemeth and M. J. T. Tan, Prog. Cryst. Growth Charact. Mater., 2013, 60, 3–4 Search PubMed.
- A. Saadati, H. Hesarikia, M. R. Nourani and R. A. Taheri, Surf. Eng., 2020, 36, 908–918 CrossRef CAS.
- P. J. Kelly and R. D. Arnell, Vacuum, 2000, 56, 159–172 CrossRef CAS.
-
D. Valerini, L. Tammaro, F. Di Benedetto, L. Capodieci, R. Terzi and A. Rizzo, Workshop on Nanotechnology in Instrumentation and Measurement (NANOFIM), 2015, 2015, 17990442.
- M. F. Smith, A. C. Hall, J. D. Fleetwood and P. Meyer, Coatings, 2011, 1, 117–132 CrossRef CAS.
- G. B. Darband, M. Aliofkhazraei, P. Hamghalam and N. Valizade, J. Magnesium Alloys, 2017, 5, 74–132 CrossRef.
- P. B. Srinivasan, J. Liang, C. Blawert and W. Dietzel, Appl. Surf. Sci., 2010, 256, 3265–3273 CrossRef.
- B. Wang, J. Gao, L. Wang, S. Zhu and S. Guan, Mater. Lett., 2012, 70, 174–176 CrossRef CAS.
- X. Chen, N. Birbilis and T. Abbott, Corrosion, 2011, 67, 035005 CrossRef.
- M. Uddin, C. Hall and P. Murphy, Sci. Technol. Adv. Mater., 2015, 16, 053501 CrossRef CAS PubMed.
- X.-B. Chen, H.-Y. Yang, T. B. Abbott, M. A. Easton and N. Birbilis, Surf. Eng., 2014, 30, 871–879 CrossRef CAS.
- R. Zeng, Z. Lan, L. Kong, Y. Huang and H. Cui, Surf. Coat. Technol., 2011, 205, 3347–3355 CrossRef CAS.
- S. Bahl, P. Shreyas, M. Trishul, S. Suwas and K. Chatterjee, Nanoscale, 2015, 7, 7704–7716 RSC.
- S. Bahl, S. R. K. Meka, S. Suwas and K. Chatterjee, ACS Biomater. Sci. Eng., 2018, 4, 3132–3142 CrossRef CAS PubMed.
- S. Acharya, A. G. Panicker, V. Gopal, S. S. Dabas, G. Manivasagam, S. Suwas and K. Chatterjee, Mater. Sci. Eng., C, 2020, 110, 110729 CrossRef CAS PubMed.
- S. Acharya, S. Suwas and K. Chatterjee, Nanoscale, 2021, 13, 2286–2301 RSC.
- L. Wagner, M. Hilpert, J. Wendt and B. Küster, Mater. Sci. Forum, 2003, 419, 93–102 Search PubMed.
- Y. Fouad, M. Mhaede and L. Wagner, Fatigue Fract. Eng. Mater. Struct., 2011, 34, 403–407 CrossRef CAS.
- M. S. Uddin, C. Hall, R. Hooper, E. Charrault, P. Murphy and V. Santos, Metals, 2018, 8, 136 CrossRef.
- M. Salahshoor and Y. Guo, Procedia CIRP, 2014, 13, 143–149 CrossRef.
- Z. Pu, J. Outeiro, A. Batista, O. Dillon Jr, D. Puleo and I. Jawahir, Int. J. Mach. Tools Manuf., 2012, 56, 17–27 CrossRef.
- M. Uddin, C. Hall, V. Santos, R. Visalakshan, G. Qian and K. Vasilev, Mater. Sci. Eng., C, 2021, 118, 111459 CrossRef CAS PubMed.
- M. Sealy and Y. Guo, J. Mech. Behav. Biomed. Mater., 2010, 3, 488–496 CrossRef CAS PubMed.
- H. Liu, Z. Tong, W. Zhou, Y. Yang, J. Jiao and X. Ren, J. Alloys Compd., 2020, 846, 155837 CrossRef CAS.
- Y. Guo, S. Wang, W. Liu, T. Xiao, G. Zhu and Z. Sun, Metals, 2019, 9, 1237 CrossRef CAS.
-
N. Von der Höh, B. Von Rechenberg, D. Bormann, A. Lucas and A. Meyer-Lindenberg, Materials science and technology: development, production, testing, properties and applications of technical materials, 2009, vol. 40, pp. 88–93 Search PubMed.
- B. Denkena and A. Lucas, CIRP Ann., 2007, 56, 113–116 CrossRef.
|
This journal is © The Royal Society of Chemistry 2021 |