DOI:
10.1039/D1MA00439E
(Paper)
Mater. Adv., 2021,
2, 7681-7690
Oxidative burst as a continuous H2O2 supplier for tumor oxygenation in photodynamic therapy†
Received
15th May 2021
, Accepted 17th September 2021
First published on 21st September 2021
Abstract
Catalyzing H2O2 decomposition to generate O2 can relieve tumor hypoxia, but the insufficient content of endogenous H2O2 limits its effect. Here, a nanomedicine consisting of Fe3O4 and zinc phthalocyanine (ZnPc12) was fabricated. In the tumor's normoxia zone, the photodynamic therapy (PDT) effect of ZnPc12 induces cancer cell's oxidative burst to generate massive endogenous H2O2. Fe3O4 catalyzes H2O2 decomposition to generate sufficient O2 for PDT in the nearby hypoxia zone. It is the first time to utilize oxidative burst as a continuous H2O2 self-supplier for cancer treatment.
1. Introduction
Photodynamic therapy (PDT) is a clinical therapy strategy for cancer treatment. The photosensitizer (PS) will produce reactive oxygen species (ROS) to kill cancer cells post light irradiation at the tumor site in the presence of O2.1–4 Compared with other photosensitizer, zinc phthalocyanines were an 18-π electron conjugate composed of tetrapolypyrrole planar macrocycles. The outer periphery of the large ring was extended by a benzene ring, which enhanced its long-wavelength absorption and had a great application in PDT.5,6 The hypoxic tumor microenvironment seriously hinders the therapeutic effect of PDT.7–9 Catalyzing endogenous H2O2 decomposition to produce O2 is a classic method to relieve tumor hypoxia.10–12 Nevertheless, the above method's efficiency is restricted mainly by the low endogenous H2O2 in cancer cells (less than 100 μM).13–16 Significantly, in cancer cells, the pericellular O2 tension strongly influences endogenous H2O2 production, sharply decreasing under hypoxic conditions.17 Thus, it is urgent to find an effective strategy to enhance endogenous H2O2 concentration.
Several strategies were reported to enhance endogenous H2O2 concentration. For example, glucose oxidase can catalyze glucose oxidation generating gluconic acid and H2O2;18 some peroxide, such as CaO2, CuO2, or MgO2, can react with H2O to generate H2O2;19 β-lapachone can cause a large number of intracellular H2O2via a futile redox cycling;20 MoSe2/CoSe2 can react with O2 to generate H2O2 by a photocatalytic reaction.21 Thus, co-delivery of these candidates with PS to cancer cells can effectively relieve tumor hypoxia to enhance the PDT outcome.
Oxidative burst was a typical response of plant cells in the physical or biological stress. The generation of ROS such as H2O2 was a symbol in plants during the oxidative burst.22,23 The oxidative burst phenomenon was also found in immune cells or macrophages, which was infected by bacteria to release H2O2.24–26 Tang and our group's previous reports found that the PDT damage could trigger the oxidative burst effect in cancer cells.27,28 We found that such an effect significantly enhances the amount of endogenous H2O2, which could be an alternative H2O2 source for hypoxia relief. Normoxic and hypoxic zones both existed in solid tumors, depending on their distances with blood vessels. For example, breast cancer has severe O2 gradients extending from ∼50 mm Hg (7% O2) to zero.29 We proposed that such an oxidative burst could be utilized as a continuous H2O2 supplier to produce O2 for cancer PDT treatment. In the tumor's normoxic zone, the PDT effect induced the cancer cell's oxidative burst to generate massive H2O2. Catalyzing H2O2 decomposition by a catalase or nanozyme can generate sufficient O2 for PDT in the hypoxic zone nearby since O2 is a small hydrophobic molecule that can be transported to hypoxic areas through a simple diffusion pathway along a concentration gradient.
To realize this vision, firstly, ZnPc with a satisfactory oxidative burst effect was selected. We synthesized twelve ZnPcs (ZnPc1–ZnPc12) with different side-chain structures and compared their oxidative burst effect post PDT treatment. Results indicated that ZnPc12 has the best oxidative burst effect post-PDT treatment. Secondly, ZnPc12 has loaded in a histidine modified Fe3O4 aggregator, an excellent catalase-mimic nanozyme, and then encapsulated by polyethylene glycol (PEG) to form a stable nanocomplex (denoted HFZP) (Fig. 1). Fe3O4 integrates catalysis, magnetic targeting, and nuclear magnetic imaging (MRI) functions into one.30,31 After the tail vein injection into mice, HFZP can accumulate at the tumor site assisted by a magnetic field and an enhanced permeability and retention (EPR) effect. Post light irradiation at the tumor tissue, the PDT process of ZnPc12 triggers the cancer cell's oxidative burst to produce abundant H2O2 at the tumor's normoxic zone. Moreover, Fe3O4 catalyzes H2O2 decomposition to generate sufficient O2 for PDT in the nearby hypoxia zone for PDT outcome enhancement.
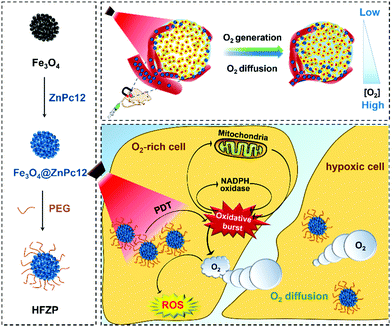 |
| Fig. 1 The schematic presentation of the fabrication and anticancer mechanism of HFZP. | |
2. Experimental section
2.1 Cells and animals
The human cervical carcinoma cell line (HeLa) and murine mammary carcinoma cell line (4T1) were purchased from the Cell Bank of the Chinese Academy of Science (Shanghai, China). The cells were cultured in a conventional incubator (5% CO2, 37°, for normoxia condition study) in DMEM based cell culture medium or a tri-gas incubator (5% CO2, 5% O2, 90% N2, 37 °C, for hypoxia condition study) using N2 saturated DMEM based cell culture medium. The female BALB/c nude mice (4–6 weeks) were purchased from the Center for Comparative Medicine of Yangzhou University. The animal experiments were conducted following the National Institute of Health Guide's guidelines for the Care and Use of Laboratory Animals. The Animal Ethics Committee approved all animal experiments of Nanjing Normal University (China).
2.2 H2O2 measurement of ZnPcs
BES–H2O2–Ac was a fluorescent probe, which was specific to H2O2. Tumor cells were incubated in 48-well plates for 24 h in an incubator (5% CO2, 37 °C, for normoxia condition study). ZnPc1–ZnPc10 (5 μM) was added and incubated for another 4 h. The cells were irradiated by a 665 nm light-emitting diode (LED) for 5 min. After 3 h, the BES–H2O2–Ac probe (5 μM) was added in the cells for 1 h, and the cells were washed with phosphate-buffered saline (PBS) three times. And an inverted fluorescence microscope detected the fluorescence intensity of different ZnPcs. The H2O2 generation in different times of ZnPc4, ZnPc11, and ZnPc12 was detected in the following measurement. ZnPc4, ZnPc11, and ZnPc12 (5 μM) were added and incubated for 3 h. The BES–H2O2–Ac probe (5 μM) was added in cells for 1 h, and the cells were irradiated for 5 min. The fluorescence images were observed at 0 h. ZnPc4, ZnPc11, and ZnPc12 (5 μM) were added and incubated for 3 h. After irradiation for 5 min, the cells were incubated for another 11, 23, or 47 h. Then the BES–H2O2–Ac probe (5 μM) was added in cells for 1 h and the fluorescence images were observed at 12, 24, or 48 h using an inverted fluorescence microscope (the exposure time was 600 ms).
2.3 Safety and cytotoxicity detection of ZnPcs
Tumor cells were cultured in 96-well plates for 24 h in an incubator (5% CO2, 37 °C, for normoxia condition study). ZnPc1–ZnPc10 (5 μM) was added with or without irradiated by a 665 nm light for 5 min after 4 h. For another 20 h, 10% 3-[4,5-dimethylthiazol-2-yl]-2,5-diphenyl tetrazolium bromide (MTT) was added and incubated for 4 h. Then, the original Dulbecco's minimum essential medium (DMEM) was removed, and dimethylsulfoxide (DMSO) was added to detect the optical density (OD) value at 570 nm using a microplate reader. The cell survival percent of ZnPc4, ZnPc11, and ZnPc12 in the dark and light cytotoxicity were also detected by the above method.
2.4 Preparation and characterization of HFZP
Fe3O4 was synthesized according to previous reports with the following procedures. FeCl3·6H2O (0.41 g), histidine (0.25 g), and sodium acetate (1.80 g) were dissolved in ethylene glycol (40 mL) by stirring and ultrasonic dispersing until it was clear. The above solution was then transferred into a Teflon-lined stainless-steel autoclave and reacted at 200 °C for 20 h. After cooling to room temperature, Fe3O4 was obtained and washed with ethanol five times and vacuum dried below 40 °C. Fe3O4 (5 μL, 12 mg mL−1) and ZnPc12 (5 μL, 2 mg mL−1) were mixed in water (5 mL) and ultrasonically dispersed for 15 min. ZnPc@Fe3O4 was obtained and then collected by centrifugation (the payload of ZnPc on Fe3O4 was 0.148 mg mg−1). After that, ZnPc@Fe3O4 and PEG (5 μL, 50 mg mL−1) were mixed in water (5 mL) and ultrasonically dispersed for 15 min. The result of HFZP was collected by centrifugation. The loading efficiency of ZnPc12 on HFZP was then calculated (the payload of ZnPc on HFZP was 0.037 mg mg−1).
2.5 H2O2, O2 and 1O2 measurements
Tumor cells were incubated in 48-well plates for 24 h under normoxia conditions (5% CO2, 37 °C). ZnPc12 and HFZP were added using an N2 saturated DMEM based cell culture medium and placed in a tri-gas incubator (5% CO2, 5% O2, 90% N2, 37 °C, for hypoxia condition study). Other HFZP groups were added magnets for providing the magnetic field under the plates. The cells were incubated for another 3 h. After that, the cells were irradiated for 5 min and continued to be incubated for 23 h. Then BES–H2O2–Ac, Tris (4,7-diphenyl-1,10-phenanthroline)ruthenium(II) dichloride (Ru(dpp)3Cl2), and singlet oxygen sensor green (SOSG) probe (5 μM) were added to the cells. After 1 h, the cells were washed with PBS. The cells were irradiated for 5 min, and an inverted fluorescence microscope detected the fluorescence intensity of different drugs (the exposure times of the above probes were 800, 500, and 600 ms, respectively).
2.6 Antitumor activity in vitro
Tumor cells were cultured in 96-well plates for 24 h under normoxic conditions (5% CO2, 37 °C). ZnPc12 (2.5 μM), Fe3O4 (34.05 mg L−1), and HFZP (ZnPc: 2.5 μM, Fe3O4: 34.05 mg L−1, PEG: 97.12 mg L−1) were added using an N2 saturated DMEM based cell culture medium and placed in a tri-gas incubator (5% CO2, 5% O2, 90% N2, 37 °C, for hypoxia condition study). Other HFZP groups were added magnets for providing the magnetic field under the plates. The cells were irradiated by a 665 nm light for 5 min after 4 h. For another 20 h, 10% MTT was added and incubated for 4 h. Then, the original DMEM was removed, and DMSO was added and the OD value was detected using a microplate reader.
2.7 Apoptosis detection in vitro
Tumor cells were cultured in 6-well plates for 24 h under normoxia conditions (5% CO2, 37 °C). ZnPc12, Fe3O4, and HFZP were added using an N2 saturated DMEM based cell culture medium and placed in a tri-gas incubator (5% CO2, 5% O2, 90% N2, 37 °C, for hypoxia condition study). Other HFZP groups were added magnets for providing the magnetic field under the plates. After irradiation for 5 min for incubating for another 24 h, DMEM culture solution was sucked out into the centrifuge tube, and the cells were washed with PBS and digested for appropriate time by tyrisin. The above DMEM was added to blow adherent cells and the cells were transferred to the centrifuge tube. Then the above cells were centrifuged at 1000 g for 5 min. The supernatant was removed and the cells were resuspended by PBS. After centrifugation again, the cells were collected and Annexin V-FITC binding solution was added to resuspend gently. After that, Annexin V-FITC (2.5 μL) and propidium iodide (PI, 5 μL) were added for blending. The cells were incubated at room temperature for 20 min in the dark and then detected using a flow cytometer (FCM).
2.8 Subcellular localization
HeLa cells were seeded in the confocal dish and incubated for 24 h. The original DMEM culture solution was removed and DMEM containing the drug (HFZP: 2 μM, calculated as ZnPc12) was added for another 4 h. Then the original medium was removed and the cells were washed with PBS. Lyso-tracker blue (75 nM) was added to the cells and cultured for 30 min. After that, the probe was taken out and the cells were washed with PBS. A confocal laser scanning microscope (CLSM) was used to obtain the fluorescence of Lyso-tracker blue and ZnPc12.
2.9
In vivo fluorescence imaging
4T1 cells (1 × 107 mL−1) were subcutaneously injected (200 μL) in female BALB/c nude mice to establish bilateral tumor-bearing. When the tumor size reached approximately 100 mm3, saline and HFZP solution (2.5 mg kg−1, calculated as ZnPc12) were intravenously injected via the tail. The magnetic field was applied to the right-side tumor for 1 h. After 6 h, the mice were sacrificed to obtain the main organs, including heart, liver, spleen, lungs, kidneys, and tumor. The fluorescence distribution in vivo was measured by animal living imaging instrument (ALII) (Ex = 405 nm, Em = 580–720 nm).
2.10 MRI measurements
[Fe] was a typical T2 contrast agent and had a high relaxation rate (r). The relaxation rates of HFZP were detected to compare the contrast imaging effect. The concentration of [Fe] in HFZP was 0.019, 0.038, 0.076, 0.152, and 0.304 mM. The above samples were added to 1.5 mL centrifuge tubes and fixed with agarose, scanning using a low-field resonance imaging scanner at room temperature. The relaxation rate calculation formula is as follows:
where T0 is the relaxation time of the agarose aqueous solution, T is the relaxation time of the test sample, [Fe] is the concentration HFZP, and r is the relaxation rate.
In this experimental section, the measurement conditions (T2) were listed as follows: coil was selected 1#, TR was set to 2000, TE was set to 50 and average was set to 6. Then, T2-MRI was measured in tumor-bearing mice before and after injecting HFZP (2.5 mg kg−1). 1 h after administration, the magnetic field was added near one tumor position for 1 h. 6 h after injection, the mice were anesthetized for scanning.
2.11 Antitumor activity in vivo
The mice were randomly divided into 6 groups, and different treatments were given as follows: (1) control group: saline without light; (2) ZnPc12 + L group: 2.5 mg kg−1 with light; (3) Fe3O4 group: 16.89 mg kg−1 with light; (4) HFZP + M group: 2.5 mg kg−1 (calculated as ZnPc12) with the magnetic field and without light; (5) HFZP + L group: 2.5 mg kg−1 (calculated as ZnPc12) without a magnetic field and with light; and (6) HFZP + M + L group: 2.5 mg kg−1 (calculated as ZnPc12) with the magnetic field and with light. The samples were administered tail vein injection on days 1, 2, 3, 4, 5, 6, 7, 8, 10, and 13. After injection for 1 h, the HFZP + M and HFZP + M + L groups were added magnets for providing the magnetic field in the tumor for 1 h. After injection for 6 h, ZnPc12, HFZP + L, and HFZP + M + L groups were irradiated by a 665 nm LED light for 30 min. The weight and tumor volume were recorded every day. After treatment for 14 days, the mice were sacrificed to obtain the main organs and tumor sections for H&E staining analysis.
2.12 Immunofluorescence detection
The mice were treated with the above antitumor activity in vivo conditions. Then pimonidazole hypoxyprobe-1 (60 mg kg−1) was administrated in mice by intraperitoneal injection. 90 minutes later, the tumor was taken out and embedded with an optimal cutting temperature compound (OCT) embedding medium for the frozen section. The obtained slices were placed in precooled acetone at 4 °C and fixed for 15 min. Then the slices were taken out and washed with PBS (1×) 3 times, and the slices were gently shaken for 10 min each time. After that, the slices were placed in PBS (1×) containing 0.2% TX-100 for 15 min and washed with PBS (1×) 3 times. The tissues were painted with a histochemical pen, and the sealing solution (goat serum) was added to the tissue in the circle of the histochemical pen for incubating for 1 h in the dark. The obtained sections were incubated with the mouse IgG1 primary antibody (1
:
150, v-v) and CD 31 antibody (1
:
200, v-v) overnight at 4 °C. After the slices were removed and restored to room temperature, they were cleaned with PBS (1×) 3 times and gently shaken for 10 min each time. The secondary antibody rabbit IgG (1
:
150, v-v) was added for incubating for 1 h in the dark, and the fluorescence images were observed using CLSM.
2.13 Fe2+ release detection of Fe3O4
The measurement of the standard curve: o-phenanthroline was a color agent that could combine Fe2+ to form the red complex. According to the Lambert–beer law (A = εbc), the concentration of Fe2+ could be calculated. FeCl3 (1 mg mL−1) was respectively taken out in 0, 10, 20, 30, 40, and 50 μL and added in the aqueous solution (3 mL), and then 0.15% o-phenanthroline (300 μL) and 10% hydroxylamine hydrochloride (100 μL) were added to the mixture solution. After reacting for 2 min, different Fe2+ concentrations corresponding to the UV-Vis spectrum were detected and the standard curve was calculated.
The measurement of Fe2+ release: the samples were divided into 8 groups, and different treatments were given as follows: (1) 0 h – H2O2: 3 mL aqueous solution (pH = 4.5) + 15 μL Fe3O4 (10 mg mL−1), the above solution was mixed and centrifuged immediately. The supernatant was collected for detection. (2) 0 h + H2O2: 3 mL aqueous solution (pH = 4.5) + 15 μL Fe3O4 (10 mg mL−1) + 30 μL H2O2 (100 mM), the above solution was mixed and centrifuged immediately. The supernatant was collected for detection. (3–8) 24 h, 48 h, 72 h, 96 h, 120 h and 144 h + H2O2: 3 mL aqueous solution (pH = 4.5) + 15 μL Fe3O4 (10 mg mL−1) + 30 μL H2O2 (100 mM), the above solution was mixed and centrifuged every day respectively at the same time. The supernatant was collected for detection. After that, 0.15% o-phenanthroline (300 μL) and 10% hydroxylamine hydrochloride (100 μL) were added to the above supernatant. After reacting for 2 min, the maximum absorbance at 510 nm was measured using the UV-Vis spectrum.
3. Results and discussion
3.1. Oxidative burst effect by ZnPcs
The substitution group of ZnPc could strongly influence its properties. Therefore, ten ZnPcs (ZnPc1–ZnPc10) with various substitution groups were chosen for the study (the synthetic routes of the ZnPcs are given in the ESI,† and their structures are shown in Fig. S1–S5†). The BES–H2O2–Ac probe, which could interact with H2O2 specifically to generate the fluorescent product DFF with bright green fluorescence, was used to detect the H2O2 concentration enhancement post the PDT process of the above ZnPcs.32
The concentration of ZnPcs was chosen as 5 μM since there is no apparent dark cytotoxicity of these ZnPcs at this concentration (Fig. S6, ESI†). Post 665 nm light irradiation for 5 min and incubation in the dark for 24 h, the brightest DFF green fluorescence was detected in the cells treated with ZnPc4 (Fig. S7, ESI†). This phenomenon proves that ZnPc4 could effectively induce oxidative burst to generate H2O2 post the PDT process, and the benzoic acid group substitution could help this effect. Thus, ZnPc11 and ZnPc12 with more benzoic acid group substitution were synthesized, and their oxidative burst effect was compared (Fig. 2B). After PDT treatment, the DFF fluorescence intensity of ZnPc12-treated cells was the strongest, indicating that its oxidative burst induced H2O2 generation amount is higher than those of ZnPc4 and ZnPc11. Besides, ZnPc12 has satisfactory light-toxicity to the cancer cells under normoxic conditions (Fig. S8, ESI†). Thus, ZnPc12 was selected for the following study in this paper.
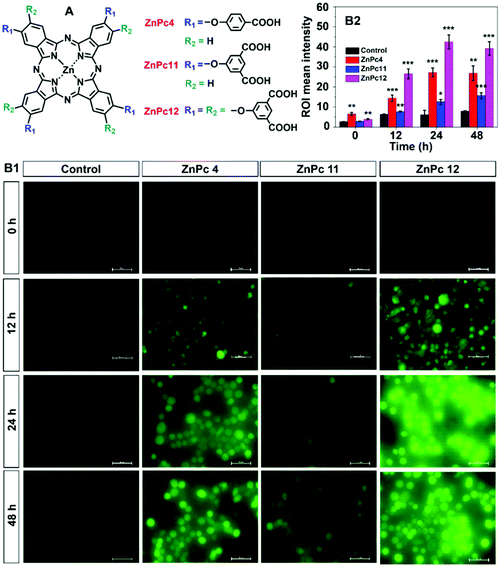 |
| Fig. 2 (A) The structures of ZnPc4, ZnPc11, and ZnPc12; (B1) DFF fluorescence signal and (B2) intensity comparison from ZnPc4, ZnPc11, or ZnPc12 treated cells post light irradiation and incubation for 0 h, 12 h, 24 h or 48 h in the dark (bar = 50 μm) (*P < 0.05, **P < 0.01, ***P < 0.001 ZnPcs treated groups versus control). | |
3.2. The mechanism of oxidative burst
H2O2 can be directly generated by the type I mechanism of the PDT.33 As shown in Fig. 2B1, trace H2O2 generated post light irradiation immediately, which is referred to as 0 h after irradiation, indicates that the type I PDT is not the primary source of H2O2 in the oxidative burst. Endogenous H2O2 of the cell can be generated by the mitochondrial respiratory chain and NADPH oxidase. To prove that the endogenous H2O2 generation is a critical source of the oxidative burst, the mitochondrial respiratory chain complex II inhibitor, thenoyltrifluoroacetate (TTFA),34 and an NADPH oxidase inhibitor, apocynin,35 were used. As shown in Fig. 3A, adding TTFA or apocynin decreased DFF's green fluorescence intensity sharply, indicating that mitochondrial respiratory chain and NADPH oxidase dysfunction inhibits the oxidative burst of ZnPc12. The results indicated that in addition to the PDT type I mechanism, the mitochondrial respiratory chain and NADPH oxidase influence by the PDT process of ZnPc12 participated in the endogenous H2O2 production to induce the H2O2 burst.
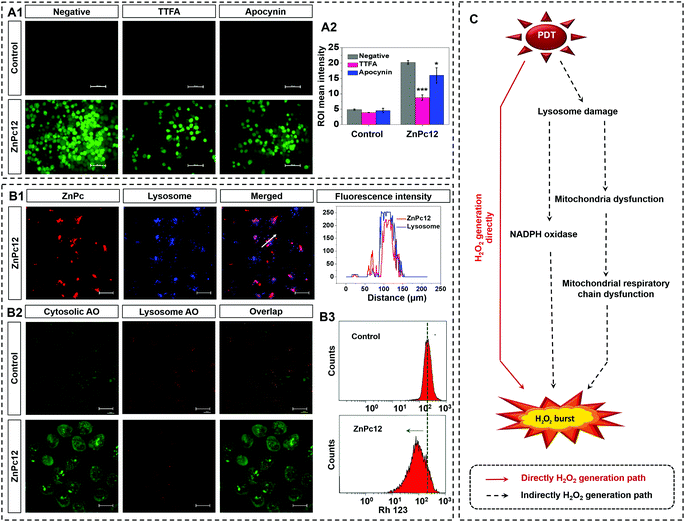 |
| Fig. 3 (A) Fluorescence images and intensity comparison of oxidative burst by TTFA and apocynin inhibitor pretreatment (bar = 100 μm). (B1) Subcellular localization was detected by ZnPc12 using a lysosome probe (bar = 20 μm). Lysosome membrane permeability and mitochondrial membrane potential were measured by AO (B2) and Rh 123 staining (B3) (bar = 20 μm). (C) The mechanism of ZnPc12 induced oxidative burst post the PDT process. | |
ZnPc12 was localized in the lysosome (Fig. 3B1). How does it influence the mitochondrial respiratory chain and NADPH oxidase located at the mitochondria and membrane, respectively? Therefore, the PDT damage process caused by light started from the lysosome. Endogenous H2O2 in tumor cells was mainly produced in mitochondria.36,37 ROS generation in the lysosome changed the lysosomal membrane permeabilization (LMP), which was verified by the acridine orange (AO) release experiment (Fig. 3B2). LMP induced protease was released into the cytoplasm to influence the NADPH oxidase and mitochondrion membrane permeabilization (MMP), which was verified by Rhodamine-123 (Rh 123) experiment (Fig. 3B3). MMP could result in mitochondrial dysfunction to influence the respiratory chain.38,39
In summary, there are direct and indirect H2O2 sources in the oxidative burst. The direct H2O2 source is from the type I mechanism of the PDT. After the injury by the ROS generated by PDT, the dysfunction of the mitochondrial respiratory chain and the NADPH oxidase caused indirect H2O2 production is the indirect H2O2 sources in the oxidative burst (Fig. 3C).
3.3. Fabrication and properties of HFZP
The histidine modified ultra-small Fe3O4 aggregator is prepared by a modified hydrothermal method, and the histidine modification is proved by the XPS spectra (Fig. S10, ESI†). After loading ZnPc12, no noticeable morphology changes were detected for Fe3O4 (Fig. 4A1 and A2). The payload of ZnPc12 on Fe3O4 was 0.148 mg mg−1. Furthermore, PEG was encapsulated on the surface of Fe3O4@ZnPc12 to obtain HFZP and the payload of ZnPc12 on HFZP was 0.037 mg mg−1. After being encapsulated by PEG, a transparent layer appeared around the nanoparticles (Fig. 4A3), and its average size was 183.6 nm (Fig. S11, ESI†). More importantly, dynamic light scattering (DLS) patterns in different physiological solutions (including saline, PBS, and DMEM) were also detected and there was no obvious HFZP aggregation (Fig. S12, ESI†). After loading ZnPc12 on the surface of positively charged Fe3O4, the zeta potential was decreased to −16.93 mV. The negatively charged PEG encapsulation made the zeta potential of HFZP to be −25.9 mV (Fig. S13, ESI†). It is indicated that ZnPc12 and PEG were effectively loaded onto Fe3O4. HFZP can be well dispersed in the aqueous solution, while HFZP moves to the magnet's position after adding the magnet, proving that HFZP has satisfactory magnetism (Fig. S14, ESI†). In the solution of Fe3O4 or HFZP solution in the presence of H2O2, massive O2 was generated, proving the catalase-mimic function of Fe3O4 (Fig. 4B). The ultrathin section of the cells treated with HFZP was observed by TEM to prove that HFZP can be effectively taken up by cancer cells (Fig. S15, ESI†). HFZP was located at the lysosome, which was proved by co-treating the cancer cells by HFZP and Lyso-tracker blue (Fig. S16, ESI†).
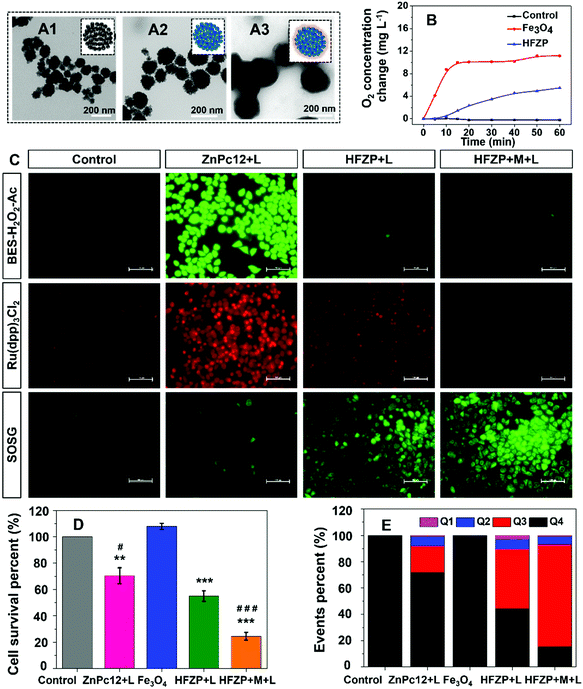 |
| Fig. 4 TEM image of Fe3O4 (A1), Fe3O4@ZnPc (A2) and HFZP (A3). (B) O2 generation comparison in aqueous, Fe3O4 and HFZP solution for 60 min (H2O2: 20 mM). (C) Comparison of fluorescence images of H2O2, O2 and 1O2 was observed by BES–H2O2–Ac, Ru(dpp)3Cl2, and SOSG probes (bar = 100 μm). (D) The light cytotoxicity comparison of Control, ZnPc12 + L, Fe3O4, HFZP + L and HFZP + M + L (**P < 0.01, ***P < 0.001 drugs treated versus control; #P < 0.05, ###P < 0.001 ZnPc12 + L and HFZP + L versus HFZP + L). (E) Cell apoptosis quantitative analysis of control, ZnPc12 + L, Fe3O4, HFZP + L and HFZP + M + L by FCM. | |
The following in vitro experiments were carried out under hypoxic conditions in the tri-gas incubator (5% CO2, 5% O2, 90% N2). The detected H2O2, O2 and 1O2 were used as BES–H2O2–Ac, Ru(dpp)3Cl2, and SOSG probes, respectively. As shown in Fig. 4C, BES–H2O2–AC is a kind of fluorescent probe specific for H2O2.40 Compared to the cells treated with ZnPc12, the H2O2 concentration in the cells treated with HFZP was decreased, indicating the decomposition of H2O2 catalyzed by HFZP in these cells. Ru(dpp)3Cl2 was widely used as a probe for luminescence detection and oxygen quantitative determination. The fluorescence intensity of Ru(dpp)3Cl2 (absorbance λmax = 455 nm, emission λmax = 613 nm) was significantly reduced by O2 due to quenching and it is an effective oxygen probe based on strength or decay time measurements.41 In contrast, the intracellular O2 concentration in the cells treated with HFZP was higher than that in ZnPc12 treated cells, indicating effective O2 generation from H2O2 decomposition. Moreover, compared with the cells treated with HFZP but without a magnetic field, the cells treated with HFZP with a magnet (HFZP + M) showed more obvious H2O2 decomposition and O2 generation since the magnetic field could promote HFZP uptake by cancer cells (Fig. 4C). SOSG was used as an indicator to evaluate 1O2 production in different groups.42 Compared to the cells treated with ZnPc12, the 1O2 concentration in the cells treated with HFZP was increased. The results showed that HFZP could produce 1O2 effectively and had better activity in a complicated physiological environment. The in vitro antitumor activity of HFZP was researched by MTT assay. Compared to ZnPc12, the light-toxicity of HFZP was enhanced under hypoxic conditions. Moreover, under the magnetic field, the PDT activity of HFZP was further improved (Fig. 4D). Besides, the cell apoptosis of the drugs was also detected by FCM. The apoptotic ratio of HFZP + M was 83.61%, superior to those of the other groups (Fig. 4E and Fig S17, ESI†).
3.4
In vivo bio-distribution and MRI imaging of HFZP
The in vivo HFZP distribution was detected using a bilateral tumor-bearing mice model. One side of the tumor has put a magnet to provide the magnetic field (HFZP + M side) to evaluate the magnetic field assisted targeting ability of HFZP, and another side has not put the magnet (HFZP side). HFZP could effectively accumulate at the tumor tissue. Moreover, the HFZP + M side fluorescence intensity was more robust than the HFZP side in bearing bilateral tumor mice, indicating the magnetic field assisted targeting function of HFZP (Fig. 5A).
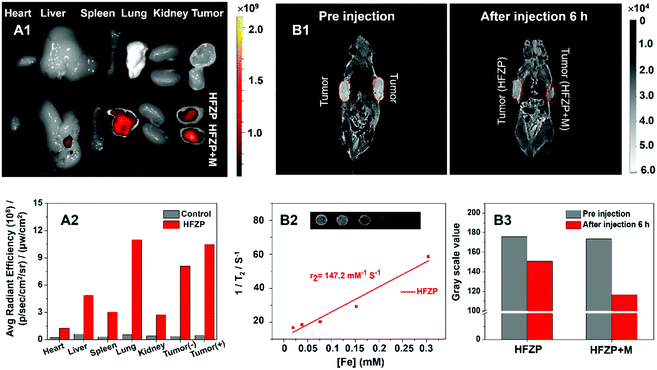 |
| Fig. 5 (A) In vivo fluorescence images and intensity of main organs and tumors pre injection and after injection HFZP without or with a magnet. T2-weighted MRI images (B1) and gray value (B3) of bilateral tumor bearing mice by HFZP and HFZP + M post tail vein injection at 0 and 6 h. (B2) Linearity curve of r2 (1/T2) versus [Fe] of corresponding relaxivity. The inset shows T2-weighted MRI images by different [Fe] concentrations in HFZP. | |
T
2-MRI is a susceptible technology to detect cancer. To create a T2-weighted image, magnetization is allowed to decay before measuring the MRI signal by changing the echo time. T2-Weighted image weighting is appropriate for detecting water-rich pathological tissue, such as tumors, edema, and inflammation. Fe-containing nanomaterials can be used as T2 contrast agents. Therefore, with the increasing concentration of HFZP, the T2-weighted MRI signal intensity was gradually decreased, which demonstrated the targeting abilities of HFZP.43 The inset panel in Fig. 5B2 showed an increase in the T2-MRI signal strength of HFZP with an increase in the Fe concentration, proving that HFZP is an ideal T2-MRI regent. The relaxation rate (r) of HFZP was 147.2 mM−1 S−1. As shown in Fig. 5B1 and B3, before injection, there was no magnetic material [Fe] and so there was a strong MRI signal in the tumor. However, the MRI signal decreased because of the magnetization effect of [Fe] and [Fe] was contained within the HFZP. Therefore, 6 h post-injection of HFZP, the T2-MRI signals showed a sharp decrease in the tumor, which also indicates the targeting ability of HFZP. With the assistance of the magnetic field, the tumor tissue's T2-MRI signal was further decreased, indicating the magnetic field assisted targeting ability of HFZP.
3.5 The depth of tumor penetration and tumor suppression functions
The blood supply of the solid tumor is insufficient, resulting in the tumor cells close to the blood vessels being normoxic, and most tumor cells far away from the blood vessels being hypoxic.44,45
The CD 31 antibody was used to label the blood vessels in tumor tissue. The hypoxic probe (pimonidazole hypoxyprobe-1) was applied to observe the O2 concentration at the tumor tissue post-treatment by various drugs. The bright green fluorescence of pimonidazole hypoxyprobe-1 can be effectively enhanced under hypoxic conditions but quenched by O2 under well-oxygenated conditions. As shown in Fig. 6A, in the solid tumor of the mice without any treatment, the green fluorescence signal intensity of the normoxic zone (around the blood vessel) was weak, and the fluorescence signal intensity of the hypoxic zone (far away from the blood vessel) was vigorous. In the solid tumor of ZnPc12 treatment mice, the green fluorescence signal intensity was enhanced overall even around blood vessels, demonstrating that O2 was consumed during the PDT process of ZnPc12. In contrast, the hypoxia was efficiently relieved overall in the solid tumor zones, both near and far away from the blood vessel, of the HFZP group. These data proved that in the tumor normoxic zone, the PDT effect of ZnPc12 in HFZP induces cancer cell oxidative burst to generate massive endogenous H2O2. Then, Fe3O4 in HFZP catalyzes H2O2 decomposition to generate sufficient O2 for PDT in the nearby hypoxia zone.
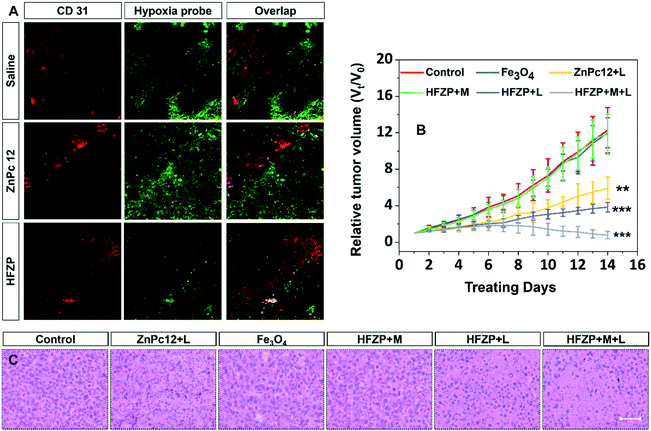 |
| Fig. 6 (A) Immunofluorescence staining images of tumor tissue from control, ZnPc12, and HFZP groups using the CD 31 antibody and hypoxia probe labeling. (B) Relative tumor volume (Vt/V0) during 14 days therapy of various drugs treated mice (**P < 0.01, ***P < 0.001 drugs treated versus control). (C) H&E staining of tumor tissue sections from the mice post different strategy treatments (bar = 50 μm). | |
Then in vivo biological safety and tumor suppression activity of ZnPc12, Fe3O4, and HFZP under the different conditions were studied further. As shown in Fig. S18A (ESI†), all mice treated with various drugs under different conditions did not show noticeable weight loss. After treatment for 14 days, no histopathological damage was detected in the main organs from the H&E staining of these tissues’ sections. These results indicated that ZnPc12, Fe3O4, and HFZP had excellent biocompatibility in vivo (Fig. S18B, ESI†).
Compared with the control group, Fe3O4 and HFZP + M groups did not show apparent tumor suppression. In contrast, the tumor growth of ZnPc12 + L, HFZP + L, and HFZP + M + L groups was significantly inhibited. The in vivo PDT activity of HFZP was superior to that of ZnPc12, and the magnetic field assisted tumor-targeting ability further enhances the PDT outcome of HFZP (Fig. 6B). The H&E staining results of tumor sections also prove these conclusions, and the HFZP + M + L group induced the most severe nuclear damage in all groups (Fig. 6C). The above data proved that HFZP has practical magnetic field-assisted tumor targeting ability and satisfactory in vivo PDT-induced tumor suppression activity.
It could also be observed that HFZP disaggregated into ultra-small particles with an average size of 8.77 nm (Fig. S19A1 and A2, ESI†) in the presence of H2O2. Furthermore, most HFZP nanoparticles were disaggregated in the cells treated with HFZP for 24 h post-light irradiation (Fig. S19B, ESI†). Such a change was mainly due to the H2O2 burst-induced HFZP nanoparticle disaggregation.
Furthermore, we added the release capacity of Fe2+ from the HFZP solution in the absence or presence of H2O2. Fe2+ release from HFZP under acidic conditions (pH = 4.5) was detected by o-phenanthroline colorimetry.46 The standard curve of Fe concentration was measured, and the linear equation was fitted y = 0.0568x + 0.0162 (Fig. S19C and D, ESI†). As shown in Fig. S19E (ESI†), Fe concentrations in supernatants were calculated to be 0.04, 0.37, 2.92, 3.81, 3.75, 3.95, 4.09, and 3.91 μg mL−1 by the standard curve at the different times, respectively. It was indicated that Fe2+ release from HFZP gradually increased with the prolonging incubation times in an aqueous acid solution in the presence of H2O2. The maximum release content of 74.09% was reached. We proposed that the disaggregation and dissolution of HFZP are conducive to its metabolism.
Just as the references mentioned, the clearance of Fe3O4-based materials in our body is always through the liver.47 In order to observe more intuitively the effects of HFZP on the liver of mice, H&E staining of main organs was performed. It could be seen that the liver was expected and no lesion performance occurs, which further demonstrates the safety of HFZP to the liver.48,49
4. Conclusions
Catalyzing endogenous H2O2 decomposition to generate O2 can relieve tumor hypoxia and enhance PDT activity. However, the endogenous H2O2 content is inadequate. Moreover, the pericellular O2 tension strongly influences the endogenous H2O2 production, significantly decreasing under hypoxic conditions. Here, we found that the PDT process of ZnPc12 induced cancer cell oxidative burst to generate massive H2O2, which can be an alternative H2O2 supplier to relieve tumor hypoxia. Then, HFZP consisting of ZnPc12, Fe3O4, and PEG was fabricated. In the tumor normoxia zone, cancer cell oxidative burst by ZnPc12 provides massive H2O2. Fe3O4 catalyzes H2O2 decomposition to generate sufficient O2 for PDT in the hypoxia zone nearby since O2 is a small hydrophobic molecule that can be transported to hypoxic areas through a simple diffusion pathway along a concentration gradient. Thus, we proposed that the oxidative burst is a continuous H2O2 self-supplier, which could be utilized for hypoxia relief in research areas including but not limited to cancer PDT treatment.
Author contributions
Xianqing Shi: design, fabrication, property identification of nanomedicine, and writing. Li Gui: in vitro and in vivo bioactivity research, ROS detection, and cell and animal imaging. Lin Zhou: design and revision. Shaohua Wei: revision and providing funds.
Conflicts of interest
There are no conflicts to declare.
Acknowledgements
X. S. and L. G. contributed equally to the work. This research was supported financially by the National Natural Science Foundation of China (21671105), the Priority Academic Program Development of Jiangsu Higher Education Institutions, the Foundation of Jiangsu Collaborative Innovation Centre of Biomedical Functional Materials (161090H001), and the Postgraduate innovation Project of Jiangsu Province (181200002470).
References
- J. Ji, X. Li, T. Wu and F. Feng, Chem. Sci., 2018, 9, 5816–5821 RSC.
- J. Karges, U. Basu, O. Blacque, H. Chao and G. Gasser, Angew. Chem., Int. Ed., 2019, 58, 14334–14340 CrossRef CAS PubMed.
- G. Z. Li, S. M. Yuan, D. S. Deng, T. Ou, Y. Q. Li, R. Sun, Q. F. Lei, X. S. Wang, W. W. Shen, Y. Y. Cheng, Z. Liu and S. Wu, Adv. Funct. Mater., 2019, 29, 1901932 CrossRef.
- N. Tian, W. Z. Sun, X. S. Guo, J. Lu, C. Li, Y. J. Hou, X. S. Wang and Q. X. Zhou, Chem. Commun., 2019, 55, 2676–2679 RSC.
- X. Li, B. D. Zheng, X. H. Peng, S. Z. Li, J. W. Ying, Y. Zhao, J. D. Huang and J. Yoon, Coord. Chem. Rev., 2019, 379, 147–160 CrossRef CAS.
- X. Li, J. F. Lovell, J. Yoon and X. Chen, Nat. Rev. Clin. Oncol., 2020, 17, 657–674 CrossRef PubMed.
- X. Li, N. Kwon, T. Guo, Z. Liu and J. Yoon, Angew. Chem., Int. Ed., 2018, 57, 11522–11531 CrossRef CAS PubMed.
- S. Kuang, L. L. Sun, X. R. Zhang, X. X. Liao, T. W. Rees, L. L. Zeng, Y. Chen, X. T. Zhang, L. N. Ji and H. Chao, Angew. Chem., Int. Ed., 2020, 59, 20679–20703 Search PubMed.
- X. H. Wang, X. Y. Wang, S. X. Jin, N. Muhammad and Z. J. Guo, Chem. Rev., 2019, 119, 1138–1192 CrossRef CAS PubMed.
- X. Yang, Y. Yang, F. Gao, J. J. Wei, C. G. Qian and M. J. Sun, Nano Lett., 2019, 19, 4334–4342 CrossRef CAS PubMed.
- J. H. Liang, Y. Zheng, X. W. Wu, C. P. Tan, L. N. Ji and Z. W. Mao, Adv. Sci., 2020, 7, 1901992 CrossRef CAS PubMed.
- Y. Ding, Z. Y. Song, Q. Liu, S. H. Wei, L. Zhou, J. H. Zhou and J. Shen, Dalton Trans., 2017, 46, 11875–11883 RSC.
- S. Dong, Y. Chen, L. Yu, K. Lin and X. Wang, Adv. Funct. Mater., 2019, 30, 1907071 CrossRef.
- Z. Wang, B. Liu, Q. Sun, S. Dong, Y. Kuang, Y. Dong, F. He, S. Gai and P. Yang, ACS Appl. Mater. Interfaces, 2020, 12, 17254–17267 CrossRef CAS PubMed.
- S. Gao, P. Zheng, Z. Li, X. Feng, W. Yan, S. Chen, W. Guo, D. Liu, X. Yang, S. Wang, X. J. Liang and J. Zhang, Biomaterials, 2018, 178, 83–94 CrossRef CAS PubMed.
- D. W. Zheng, B. Li, C. X. Li, J. X. Fan, Q. Lei, C. Li, Z. Xu and X. Z. Zhang, ACS Nano, 2016, 10, 8715–8722 CrossRef CAS PubMed.
- J. Fandrey, S. Frede and W. Jelkmann, Biochem. J., 1994, 303, 507–510 CrossRef CAS PubMed.
- M. Lyu, D. Zhu, X. Kong, Y. Yang, S. Ding, Y. Zhou, H. Quan, Y. Duo and Z. Bao, Adv. Healthcare Mater., 2020, 9, 1901819 CrossRef PubMed.
- H. Hu, L. Yu, X. Qian, Y. Chen, B. Chen and Y. Li, Adv. Sci., 2020, 8, 2000494 CrossRef PubMed.
- S. Wang, G. Yu, Z. Wang, O. Jacobson, L. S. Lin, W. Yang, H. Deng, Z. He, Y. Liu, Z. Y. Chen and X. Chen, Angew. Chem., Int. Ed., 2019, 58, 14758–14763 CrossRef CAS PubMed.
- Y. Li, R. Jia, H. Lin, X. Sun and F. Qu, Adv. Funct. Mater., 2020, 31, 2008420 CrossRef.
- J. Wu and X. Ge, Biotechnol. Bioeng., 2004, 85, 714–721 CrossRef CAS PubMed.
- G. P. Bolwell, D. R. Davies, C. Gerrish, C.-K. Auh and T. M. Murphy, Plant Physiol., 1998, 116, 1379–1385 CrossRef CAS PubMed.
- P. Yang, S. Huang and A. Xu, Amphioxus Immunity, 2016, 153–165, DOI:10.1016/b978-0-12-849903-0.00008-7.
- J. M. Slauch, Mol. Microbiol., 2011, 80, 580–583 CrossRef CAS PubMed.
- J. B. Bliska and D. S. Black, Infect. Immun., 1995, 63, 681–685 CrossRef CAS PubMed.
- T. Wang, J. Hu, H. Luo, H. Li, J. Zhou, L. Zhou and S. Wei, Small, 2018, 14, 1802337 CrossRef PubMed.
- Z. Yu, Q. Sun, W. Pan, N. Li and B. Tang, ACS Nano, 2015, 9, 11064–11074 CrossRef CAS PubMed.
- G. L. Semenza, Mol. Aspects Med., 2016, 47-48, 15–23 CrossRef CAS PubMed.
- B. Li, T. Gong, N. Xu, F. Cui, B. Yuan, Q. Yuan, H. Sun, L. Wang and J. Liu, Small, 2020, 16, 2003969 CrossRef CAS PubMed.
- Y. Yao, D. Zhao, N. Li, F. Shen, J. O. Machuki, D. Yang, J. Li, D. Tang, Y. Yu, J. Tian, H. Dong and F. Gao, Anal. Chem., 2019, 91, 7850–7857 CrossRef CAS PubMed.
- X. Jiang, H. Wang, R. Yuan and Y. Chai, Anal. Chem., 2018, 90, 8462–8469 CrossRef CAS PubMed.
- Y. Y. Wang, Y. C. Liu, H. W. Sun and D. S. Guo, Coord. Chem. Rev., 2019, 395, 46–62 CrossRef CAS.
- T. Nishikawa, D. Edelstein, X. L. Du, S.-I. Yamagishi, T. Matsumura, Y. Kaneda, M. A. Yorek, D. Beebe, P. J. Oates, H.-P. Hammes, I. Giardino and M. Brownlee, Nature, 2000, 404, 787–790 CrossRef CAS PubMed.
- L. Y. Hou, F. Q. Sun, R. X. Huang, W. Sun, D. Zhang and Q. S. Wang, Redox Biol., 2019, 22, 101134 CrossRef CAS PubMed.
- J. Sun, K. Du, J. J. Diao, X. T. Cai, F. D. Feng and S. Wang, Angew. Chem., Int. Ed., 2020, 59, 12122–12128 CrossRef CAS PubMed.
- G. Q. Yang, Z. J. Liu, R. L. Zhang, X. H. Tian, J. Chen, G. M. Han, B. H. Liu, X. Y. Han, Y. Fu, Z. J. Hu and Z. P. Zhang, Angew. Chem., Int. Ed., 2020, 59, 16154–16160 CrossRef CAS PubMed.
- C. Marques, C. S. Oliveira, S. Alves, S. R. Chaves, O. P. Coutinho, M. Corte-Real and A. Preto, Cell Death Dis., 2013, 4, 507 CrossRef PubMed.
- K. Xu, H. Yao, J. Hu, J. Zhou, L. Zhou and S. Wei, Free Radical Biol. Med., 2018, 124, 431–446 CrossRef CAS PubMed.
- H. Maeda, Y. Fukuyasu, S. Yoshida, M. Fukuda, K. Saeki, H. Matsuno, Y. Yamauchi, K. Yoshida, K. Hirata and K. Miyamoto, Angew. Chem., Int. Ed., 2004, 43, 2389–2391 CrossRef CAS PubMed.
- W. P. Li, C. H. Su, Y. C. Chang, Y. J. Lin and C. S. Yeh, ACS Nano, 2016, 10, 2017–2027 CrossRef CAS PubMed.
- B. Liu, S. Liang, Z. Wang, Q. Sun, F. He, S. Gai, P. Yang, Z. Cheng and J. Lin, Adv. Mater., 2021, 33, 2101223 CrossRef CAS PubMed.
- J. Tang, H. Zhou, J. Liu, J. Liu, W. Li, Y. Wang, F. Hu, Q. Huo, J. Li, Y. Liu and C. Chen, ACS Appl. Mater. Interfaces, 2017, 9, 23497–23507 CrossRef CAS PubMed.
- E. F. Garner and E. A. Beierle, Cancers, 2016, 8 DOI:10.3390/cancers8010005.
- J. A. Joyce and D. T. Fearon, Science, 2015, 348, 74–80 CrossRef CAS PubMed.
- D. Wang, J. Zhou, R. Chen, R. Shi, G. Xia, S. Zhou, Z. Liu, N. Zhang, H. Wang, Z. Guo and Q. Chen, Biomaterials, 2016, 107, 88–101 CrossRef CAS PubMed.
- J. Wang, Y. Chen, B. Chen, J. Ding, G. Xia, C. Gao, J. Cheng, N. Jin, Y. Zhou, X. Li, M. Tang and X. M. Wang, Int. J. Nanomed., 2010, 5, 861–866 CAS.
- X. Song, X. Luo, Q. Zhang, A. Zhu, L. Ji and C. Yan, J. Magn. Mater., 2015, 388, 116–122 CrossRef CAS.
- Q. L. Jiang, S. W. Zheng, R. Y. Hong, S. M. Deng, L. Guo, R. L. Hu, B. Gao, M. Huang, L. F. Cheng, G. H. Liu and Y. Q. Wang, Appl. Surf. Sci., 2014, 307, 224–233 CrossRef CAS.
Footnotes |
† Electronic supplementary information (ESI) available. See DOI: 10.1039/d1ma00439e |
‡ X. Q. Shi and L. Gui contributed equally to the work. |
|
This journal is © The Royal Society of Chemistry 2021 |
Click here to see how this site uses Cookies. View our privacy policy here.