DOI:
10.1039/D0MA00841A
(Paper)
Mater. Adv., 2021,
2, 1402-1412
Dual-emissive Ln3+/Mn4+ Co-doped double perovskite phosphor via site-beneficial occupation†
Received
28th October 2020
, Accepted 14th January 2021
First published on 14th January 2021
Abstract
The non-contact temperature detectors based on fluorescence intensity ratio (FIR) technology have been widely studied. In the past few decades, researchers have been working on optical temperature measurement via FIR technology based on the two thermally-coupled energy levels (TCLs) of rare-earth ions. However, the FIR method based on TCLs has inherent limitations, which hinder further improvement of relative sensitivity (Sr). In order to further improve the temperature measurement performance, we have designed a dual-activator luminescence system of La2LiSbO6 (LLSO) perovskite co-doped with rare-earth (Tb3+, Dy3+) ions and transition metal (Mn4+) ions according to the site-beneficial occupation principle. LLSO provides suitable occupation sites for Tb3+/Dy3+ ions and Mn4+ ions. The experimental results show that these doped ions can enter the matrix successfully and emit luminescence simultaneously. The emission peaks of Tb3+/Dy3+ ions and Mn4+ ions are well separated, which provides a good signal identification ability for temperature detection. In addition, Tb3+/Dy3+ ions and Mn4+ ions have different sensitivities to the environment due to their different external electronic configurations, which leads to different thermal quenching responses of their fluorescence emission intensity. The decline rate of Mn4+ ions is much faster than that of Tb3+/Dy3+ ions, which is very useful for temperature measurement based on FIR technology. Therefore, Tb3+/Dy3+ ions can be used as reference signals, whereas Mn4+ ions are suitable detection signals. We have investigated the temperature detection performance at different luminescent positions of luminescent ions. The results show that the fluorescence intensity ratio between Tb3+/Dy3+ ions and Mn4+ ions in the materials exhibits excellent temperature sensing performance in the temperature range of 303–523 K. The maximum relative sensitivity and absolute sensitivity of Tb3+ and Mn4+ co-doped LLSO phosphors are 0.946% K−1 and 0.00193 K−1, respectively; the maximum relative sensitivity and absolute sensitivity of Dy3+ and Mn4+ co-doped LLSO phosphors are 0.796% K−1 and 0.00832 K−1, respectively, which are much higher than those of some optical thermometric materials reported previously. The self-reference optical temperature measurement method based on double luminescent centers proposed in this paper can provide a new viewpoint for the development of high-performance thermometers.
1. Introduction
So far, luminescent materials constituting of luminescent ions and a host have been widely applied in white light-emitting diodes (W-LEDs), three-dimensional displays, medical equipment, temperature sensing and other fields.1–9 In recent years, the use of fluorescent materials as optical thermometers has attracted considerable attention of researchers because of their advantages of non-contact, real-time measurement, high spatial resolution and high accuracy.10–15 The contactless fluorescent temperature indicators overcome the shortcomings of traditional thermometers and are implemented far and wide in substances with small size or in harsh environment.16,17
Generally speaking, the matrix materials for optical temperature measurement include nanorods, nanoparticles, core–shell particles, glass ceramics containing fluorine nanocrystals and oxide blocks.18–22 This means that the phosphor can be resistant to oxidation and high temperature, and not react in harsh environment and remain stable. Trivalent lanthanide ions and some transition group ions are used as luminescent centers or activators in optical thermometry. Fluorescence temperature measurement is realized by monitoring the luminescent signal related to temperature, such as fluorescence intensity, peak position, bandwidth, fluorescence lifetime, and fluorescence intensity ratio (FIR).23–30 The temperature detection method based on FIR has the advantages of self-reference and is not affected by external factors except temperature; it has superiorities over other temperature measurement methods and has been widely applied. Generally speaking, most optical thermometers using FIR technology are based on two thermally-coupled energy levels (TCLs) of rare-earth ions, such as Er3+(2H11/2 and 4S3/2); Nd3+(4F5/2 and 4F3/2); Dy3+(4I5/2 and 4F9/2); and Eu3+(5D1 and 5D0).10,14,31–35 For temperature sensors based on TCLs, FIR obeys the Boltzmann distribution law, so relative sensitivity (Sr) is proportional to the corresponding TCL energy gap. However, the range of TCLs is generally 200–2000 cm−1, which makes it difficult to further improve Sr. In order to find an efficient FIR method to improve the performance of temperature measurement, the emission of different activation centers must overcome the inherent limitations of the FIR method based on TCLs, and provide a possibility for efficient temperature measurement. One of the emitting centers is used as the temperature probe and the other as the reference center. The emission intensity of activators usually decreases with the increase of temperature, but the quenching degree of different activators varies with temperature, one of which is faster and the other is slower, resulting in a large difference in FIR values between the two activators at different temperatures, so as to improve the temperature measurement performance. Rare-earth (RE) ions are a kind of special optical probes because their 4f active electrons are shielded by external electron sublayers, which makes them less sensitive to the environment, but enough to observe changes.36 Transition metal (TM) ions have a 3dn electronic configuration and are very sensitive to the surrounding environment. Therefore, the emission of TM ions is more affected by temperature than that of RE ions. Therefore, the dependence of TM ions and trivalent RE ions on temperature is different. It is expected that FIR based on TM ions and trivalent RE ions will change greatly with temperature, which should have high temperature sensitivity. In recent years, as a transition metal activator, Mn4+ has attracted much attention due to its low cost, deep red emission and wide excitation band.37–40
As is known, it is also very important to choose a suitable matrix as the carrier of activators. Not all matrix-doped ions can emit light, but if the matrix provides suitable sites for the doped ions to replace, then they can emit fluorescence. For example, the luminescence and thermometric properties of NaLaMgWO6:Eu3+,Mn4+ phosphors were studied by Zhou et al.41 In the NaLaMgWO6 matrix, Eu3+ ions often replace La3+ sites. Similarly, Mn4+ ions are more likely to replace W6+ ion sites. Double perovskite compounds have attracted much attention due to their high stability and low price. La2LiSbO6 is a typical perovskite structure, which is composed of [LiO6], [SbO6] octahedron and [LaO8] dodecahedron. The crystal structure of the La2LiSbO6 compound was first reported by López et al. in 1990.42 Its structure is monoclinic GdFeO3 type perovskite. A large number of octahedral sites in La2LiSbO6 (LLSO) are favorable for Mn4+ ions to enter the structure and promote luminescence. In this work, novel LLSO:Tb3+,Mn4+ and LLSO:Dy3+,Mn4+ phosphors with strong efficient dual-activator luminescence are devised and synthesized by a high-temperature solid-state method. LLSO host provides favorable site occupation for Mn4+ and Tb3+/Dy3+, specifically Mn4+ occupies the Sb site and Tb3+/Dy3+ occupies the La site, and makes them emit fluorescence. The luminescence properties and temperature dependence of these phosphors were tested and analyzed in detail, which showed their application prospects in optical temperature sensors.
2. Experimental section
2.1 Synthesis of samples
LLSO:0.005Mn4+, LLSO:0.09Tb3+, 0.005Mn4+ and LLSO:0.09Dy3+,0.005Mn4+ (the unit is mol%) phosphors and LLSO host were successfully synthesized via a high-temperature solid-state method. La2O3 (A.R.), Li2CO3 (A.R.), Sb2O3 (A.R.), MnCO3 (A.R.), Dy2O3 (≥99.9%), Tb4O7 (99.99%) as raw materials were weighed according to the stoichiometric ratio, in which Li2CO3 was over 3%. The raw materials were mixed and ground thoroughly in an agate mortar for 30 min. After full grinding, the mixed powder was transferred into an alumina crucible and calcined in air at 1200 °C for 2 h. After cooling to room temperature, the intermediate was taken out and ground into powder, and then returned to the crucible for repeated calcination. After cooling again, the product was ground into powder and put into a sample tube for testing.
2.2 Measurement and characterization
X-ray powder diffraction (XRD) of the samples was performed using an X-ray diffractometer (Bruker D8) containing Cu Kα radiation (λ = 1.5418 Å) with the operating conditions of 40 kV and 15 mA, and the XRD patterns were collected in the range of 10°–80° with the scanning steps of 0.02. The photoluminescence excitation (PLE) and emission (PL) spectra of samples were measured using a Hitachi F-7000 fluorescence spectrometer at room temperature with a 150 W xenon lamp as the excitation source. The quantum yields and decay curves of the samples were measured by an Edinburgh FS5 spectrophotometer; temperature-dependent photoluminescence (PL) characteristics were monitored via this spectrophotometer combined with a heating device (TAP 02).
3. Results and discussion
3.1 Crystal structure
The crystal structures of Mn4+ doped LLSO phosphors, Tb3+/Mn4+ and Dy3+/Mn4+ co-doped LLSO phosphors as well as un-doped samples were identified from the XRD patterns, as shown in Fig. 1(A). The diffraction peaks of the LLSO host match well with the standard card of LLSO (PDF#81-0839), indicating that the host has been successfully prepared. The XRD diffraction peaks of single doped and co-doped samples are consistent with those of LLSO, which proves that the introduction of Mn4+, Tb3+/Mn4+ or Dy3+/Mn4+ do not produce any impurity phase and cannot change the crystal structure. This indicates that these dopants are successfully incorporated into the LLSO host. Fig. 1(B) presents the crystal structure of the LLSO matrix. It is clearly seen that [LiO6] and [SbO6] connect with each other alternately through a common site to form octahedral chains, which is conducive to the entry of Mn4+ ions into its structure and promotes the luminescence of Mn4+ ions. La3+ ions occupy the eight-coordination sites. Considering the probable mechanism of effective ionic radii and coordination number (CN) of Mn4+ ion (0.53 Å, CN = 6) and Sb5+ (0.60 Å, CN = 6), it is easier for the Mn4+ activator to enter the Sb5+ locus. Similarly, Tb3+/Dy3+ ions prefer to replace the La3+ locus, which can be proved by calculating the value of the radius difference percentage (Dr), which is reported by Davolos43 and needs to be within 30%. The Dr values can be calculated using the equation: | 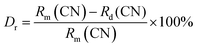 | (1) |
where Rm and Rd are the radii of matrix cations and doped cations, respectively. CN is the coordination number. The Dr values between matrix cations and doped cations are listed in Table 1. It can be clearly seen that Mn4+ ions will replace Sb5+ sites in the matrix, and Tb3+/Dy3+ ions can be doped into La3+ sites.
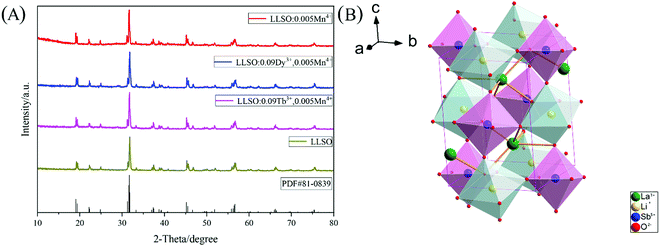 |
| Fig. 1 (A) XRD patterns of LLSO: 0.005Mn4+; LLSO: 0.09Tb3+, 0.005Mn4+; LLSO: 0.09Dy3+, 0.005Mn4+ and LLSO host. (B) Crystal structure schematic diagram of La2LiSbO6. | |
Table 1 The Dr values between matrix cations and doped cations
Matrix cations (CN) |
Doped cations (CN) |
R
m/Å |
R
d/Å |
D
r/% |
Li+(6) |
Mn4+(6) |
0.76 |
0.530 |
30.26 |
Sb5+(6) |
Mn4+(6) |
0.60 |
0.530 |
11.67 |
Li+(6) |
Tb3+(6) |
0.76 |
0.923 |
−21.45 |
La3+(8) |
Tb3+(8) |
1.160 |
1.040 |
10.34 |
Sb5+(6) |
Tb3+(6) |
0.60 |
0.923 |
−53.83 |
Li+(6) |
Dy3+(6) |
0.76 |
0.912 |
−20 |
La3+(8) |
Dy3+(8) |
1.160 |
1.027 |
11.47 |
Sb5+(6) |
Dy3+(6) |
0.60 |
0.912 |
−52 |
Fig. S1 (ESI†) shows the SEM images of each sample. It is not difficult to see that the samples exhibit irregular particle sizes in the range of 1–3 μm. In order to further confirm the structure of the synthesized samples, the standard data of LLSO were used as a reference, and Rietveld refinement of the existing samples was performed using GASA software. The final refinement mode is shown in Fig. 2, and the relevant refining parameters and crystallographic data are summarized in Table 2. The obtained final refinement parameters (Rwp, Rp and χ) show that the actual structure is in good agreement with the initial structure. The V values of the co-doped samples decreased because Tb3+(1.040 Å, CN = 8)/Dy3+(1.027 Å, CN = 8) and Mn4+(0.530 Å, CN = 6) replaced La3+(1.160 Å, CN = 8) and Sb5+(0.60 Å, CN = 6) sites, respectively, in the crystal lattice.
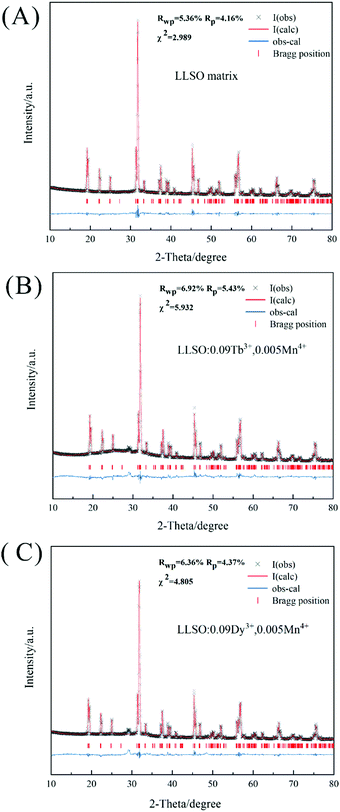 |
| Fig. 2 Rietveld refinement patterns for X-ray diffraction patterns of (A) LLSO matrix; (B) LLSO: 0.09Tb3+, 0.005Mn4+ and (C) LLSO: 0.09Dy3+, 0.005Mn4+. | |
Table 2 The relevant Rietveld refinement parameters and crystallographic data
Parameter |
LLSO matrix |
LLSO:0.09Tb3+, 0.005Mn4+ |
LLSO:0.09Dy3+, 0.005Mn4+ |
space group |
P21/n (14) |
P21/n (14) |
P21/n (14) |
a (Å) |
5.6195 |
5.6134 |
5.6137 |
b (Å) |
5.7236 |
5.7278 |
5.7259 |
c (Å) |
7.9670 |
7.9607 |
7.9605 |
α = γ (deg) |
90.000 |
90.000 |
90.000 |
β (deg) |
89.776 |
89.742 |
89.745 |
V (Å3) |
256.247 |
255.953 |
255.876 |
Units, Z |
2 |
2 |
2 |
R
p (%) |
4.16 |
5.43 |
4.37 |
R
wp (%) |
5.36 |
6.92 |
6.36 |
χ
2
|
2.989 |
5.932 |
4.805 |
3.2 Fluorescence properties and optical thermometry
Fig. 3 shows the PL and PLE spectra of Mn4+ single-doped LLSO and Dy3+/Tb3+, Mn4+ co-doped LLSO phosphors at room temperature. The excitation peaks in the range of 260–280 nm are due to the charge transfer of electrons from the oxygen ligands to the central metal atom. As shown in Fig. 3(A), the PL spectrum with 315 nm excitation wavelength presents an emission band centered at 713 nm, which originates from the spin-forbidden 2E → 4A2 transition of the Mn4+ ion. The PLE spectrum of Mn4+ monitored at 713 nm shows a wide hump in the range of 250–400 nm, three peaks centered at 276 nm, 319 nm and 354 nm were fitted by Gaussian peak splitting, and are due to the charge transfer (CT) from O2− to Mn4+, 4A2 → 4T1 and 4A2 → 2T2 spin-allowed transitions of Mn4+, respectively. The excitation peak at 480 nm corresponds to the Mn4+: 4A2 → 4T2 spin-forbidden transitions. Fig. 3[(B)a] illustrates the PLE spectrum of Tb3+ and Mn4+ ions co-doped LLSO phosphor, which was monitored at 544 nm. It consists of a broad band centered at 276 nm that is attributed to the charge transfer (CT) from O2− to Tb3+ and the peaks between 300–385 nm are attributed to 4f → 4f transitions of Tb3+. Under 276 nm excitation, the emission spectrum of LLSO:Tb3+,Mn4+ phosphor shows a series of sharp peaks at 492 nm, 544 nm, 591 nm and 624 nm as emission centers, which are 5D4 → 7FJ (J = 6, 5, 4, and 3) transitions. In addition, two weak emission peaks at 416 nm and 439 nm are due to 5D3 → 7F5 and 5D3 → 7F4 transitions, respectively. As depicted in Fig. 3[(B)b], the PLE spectrum of LLSO:Dy3+,Mn4+ phosphor, monitored at 577 nm emission wavelength displays several excitation bands centered at 350 nm, 365 nm, 388 nm and 427 nm attributed to 6H15/2 → 6P7/2, 6H15/2 → 6P5/2, 6H15/2 → 4I13/2, and 6H15/2 → 4G11/2 transitions, respectively. The PL spectrum under 274 nm excitation exhibits two characteristic emission peaks at 484 nm and 576 nm that are attributed to 4F9/2 → 6H15/2 and 4F9/2 → 6H13/2 transitions, respectively. In the PL and PLE spectra of the co-doped phosphors, the characteristic peaks of Mn4+ ions are clearly presented. We measured the quantum yields of the double-doped samples. The quantum yield of LLSO:0.09Tb3+,0.005Mn4+ phosphor was up to 45.95%, while the quantum yield of LLSO:0.09Dy3+,0.005Mn4+ phosphor was 23.42%.
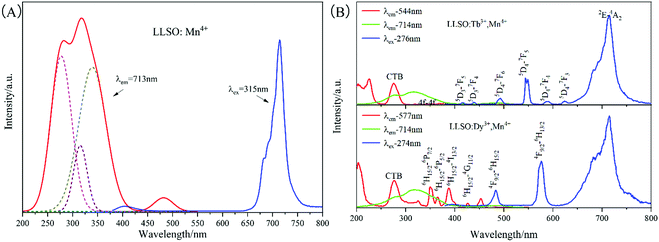 |
| Fig. 3 The PL and PLE spectra of (A) LLSO: 0.005Mn4+; (B) LLSO: 0.09Tb3+, 0.005Mn4+ and LLSO: 0.09Dy3+, 0.005Mn4+. | |
In order to further study whether there is energy transfer between Ln3+ and Mn4+, we analyzed the decay curves of samples with different concentrations, as shown in Fig. S2 (ESI†). It can be seen that with the increase of Tb3+ concentration, the lifetime of Mn4+ does not increase obviously, so there is no energy transfer from Tb3+ to Mn4+. However, with the increase of Dy3+ concentration, the lifetime of Mn4+ decreases, so there is no energy transfer from Dy3+ to Mn4+ as well. On the basis of the excitation and emission spectra received, we conjecture that the possible luminescence mechanism for Tb3+/Dy3+ ions and Mn4+ ions co-doped LLSO phosphors is shown in Fig. 4(A). Under the excitation of 276 nm or 274 nm, the electrons of Mn4+ immediately absorb energy, which is pumped from the ground state to the excited state, and then they relax to the low phonon energy 2Eg state through non-radiative relaxation. Ultimately, they return to the ground state and emit red light through radiative transitions. In the meantime, Tb3+ and Dy3+ ions are excited from the ground state to a higher excited state. Then, they are relaxed to the lower excited state through a nonradiative transition. Finally, they return to the ground state by releasing the observed photon radiation. CIE coordinates of these phosphors are shown in Fig. 4(B); the CIE coordinates of LLSO:0.005Mn4+ phosphor are (0.6481, 0.2653) in the dark red region, and the CIE coordinates of LLSO:0.09Tb3+/0.09Dy3+, 0.005Mn4+ phosphors are (0.4018, 0.4721) and (0.4319, 0.4052) in the yellow-green region and light yellow region, respectively.
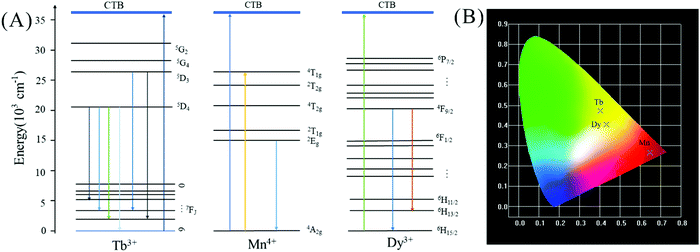 |
| Fig. 4 (A) The light-emitting mechanism of La2LiSbO6: Tb3+/Dy3+, Mn4+. (B) CIE(x, y) coordinate diagram of LLSO: 0.005Mn4+; LLSO: 0.09Tb3+, 0.005Mn4+ and LLSO: 0.09Dy3+, 0.005Mn4+. | |
According to the PLE spectra of Tb3+/Dy3+, Mn4+ co-doped LLSO phosphors, we probed the emission spectra at different excitation wavelengths, as shown in Fig. 5(A) and (B). As can be seen from Fig. 5(A), the emission spectra of the LLSO:Tb3+,Mn4+ phosphor excited at 276 nm show the appearance of characteristic peaks of Tb3+ and Mn4+ ions, and the luminescence intensity was high. Similarly, as shown in Fig. 5(B), the emission spectra of the LLSO:Dy3+,Mn4+ phosphor excited at 274 nm show the characteristic peaks of Dy3+ and Mn4+ ions, and the luminescence intensity was high. Therefore, we chose 276 nm and 274 nm as the excitation wavelengths for the Tb3+/Dy3+,Mn4+ co-doped phosphors to test the emission spectra at different temperatures.
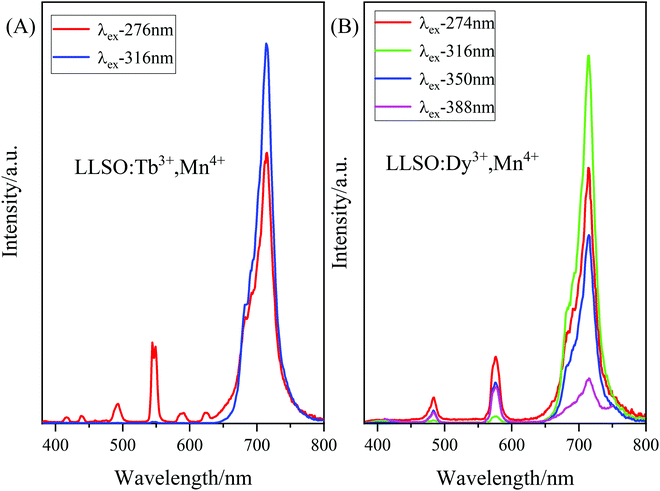 |
| Fig. 5 The PL spectra of (A) La2LiSbO6: 0.09Tb3+, 0.005Mn4+ and (B) LLSO: 0.09Dy3+, 0.005Mn4+ excited at different wavelength. | |
In order to further explore the temperature-dependent properties of phosphors, PL spectra of LLSO:0.09Tb3+/0.09Dy3+,0.005Mn4+ phosphors were monitored in the temperature range of 303–523 K, as shown in Fig. 6(A) and (C), respectively. With the increase of temperature, the luminescence intensity of Tb3+/Dy3+ and Mn4+ decreases, which is caused by heat quenching. The corresponding CIE colorimetric diagrams based on PL spectra at different temperatures are shown in Fig. S3 in the ESI.† As the temperature was increased from 303 to 523 K, a significant change in FIR (ITb/IMn) caused the transition of the chromaticity coordinates (0.3754, 0.4272) to (0.3494, 0.3898). Similarly, a significant change in FIR (IDy/IMn) caused the transition of the chromaticity coordinates (0.3826, 0.3715) to (0.3479, 0.3514). We integrated the intensities of the characteristic peaks of each ion by area integral, and their column graphs are shown in Fig. 6(B) and (D). Obviously, the luminescence intensity of Mn4+ ions decreases much faster, which is due to the unique 3d3 electron configuration of Mn4+ ions and is very sensitive to the external environment, resulting in stronger electron–phonon coupling and more intense heat quenching. Fig. 7 is the configuration coordinate diagram of Mn4+ in the LLSO host, which shows the possible thermal quenching mechanism of Mn4+. As shown in Fig. 7, Mn4+ ions are first excited from the 4A2 state to the B point of the 4T1g state, and relaxed to the bottom C point of the excited state after losing partial energy, which is due to the instability of Mn4+ ions at point B. Then it is relaxed to the E state through a nonradiative process, and then, by emitting red light (releasing photons) back to the ground state. However, with the increase in temperature, an increasing number of electrons in the 2E state gain energy and get thermally excited, which makes them more willing to reach the intersection point of 4A2 and 2E and then return to the ground state by radiation. The energy absorbed in this process is usually referred to as the activation energy of thermal quenching (ΔE). According to eqn (2), the ΔE values of Tb3+ ion and Mn4+ ion are 0.364 eV and 0.255 eV, respectively; the ΔE values of Dy3+ ion and Mn4+ ion are 0.330 eV and 0.256 eV, respectively. This is the reason why the thermal quenching of Mn4+ ion emission is faster than that of Tb3+/Dy3+ ions. This is very useful for temperature measurement based on FIR technology.
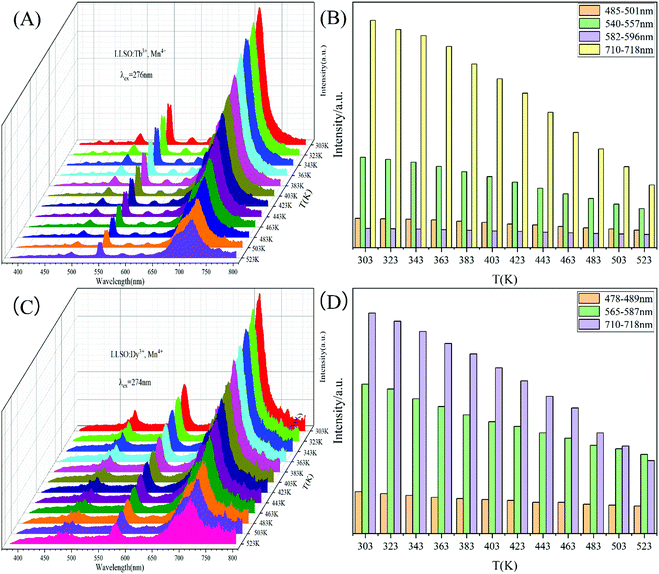 |
| Fig. 6 The temperature-dependent PL spectra of (A) La2LiSbO6: 0.09Tb3+, 0.005Mn4+ and (C) LLSO: 0.09Dy3+, 0.005Mn4+. (B), (D) The PL emission intensity of different band at various temperature. | |
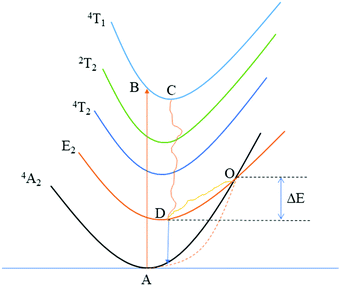 |
| Fig. 7 Configurational coordinate diagrams of Mn4+ ions. | |
In order to explore the sensitivity of the co-doped phosphors, eqn (2) can be used to express the temperature dependence of the emission intensity according to Boltzmann distribution and thermal quenching mechanism.44,45
| 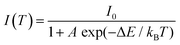 | (2) |
where
I0 is the emission intensity at the initial temperature (303 K in this work),
I(
T) refers to the emission intensity at temperature
T (unit is K),
A is a constant, Δ
E is the activation energy of heat quenching, and
kB corresponds to the Boltzmann constant (
kB = 8.629 × 10
5 eV K
−1). Considering a reasonable approximation, the temperature-dependent FIR of Tb
3+/Dy
3+ and Mn
4+ can be expressed as:
|  | (3) |
where
B and
C are constants,
E refers to the modified thermal quenching activation energy for the RE
3+/Mn
4+ dual-emitting system. The FIR values between Tb
3+ and Mn
4+ with different luminescence centers at different temperatures are shown in
Fig. 8, and the FIR values between Dy
3+ and Mn
4+ with different luminescence centers at different temperatures are shown in
Fig. 9. We use exponential fitting for these values, and the fitting relationship and fitting degree are presented in the figure. It can be seen from the figure that the exponential relationship between FIR and 1/
T fits very well. For temperature sensing, sensitivity is a very important parameter to evaluate the performance of the temperature sensor. Absolute sensitivity
Sa and relative sensitivity
Sr can be defined as:
|  | (4) |
|  | (5) |
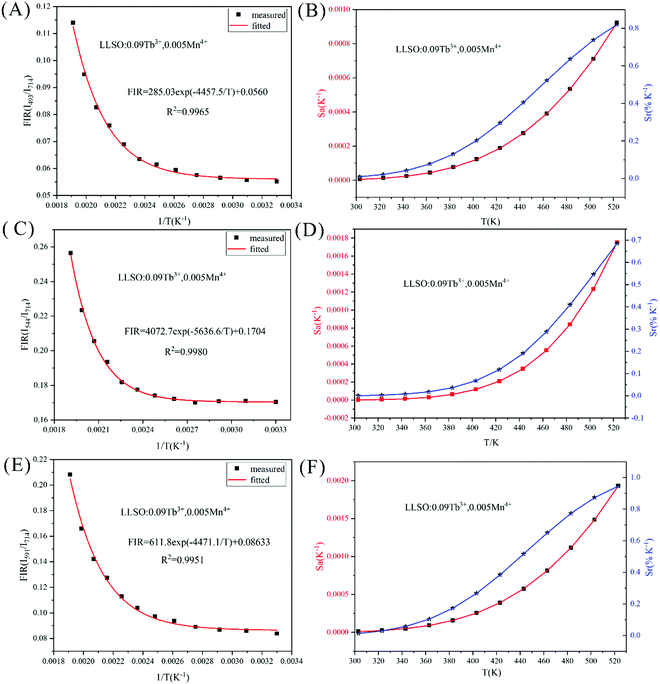 |
| Fig. 8 FIR values, Sr and Sa values of La2LiSbO6:0.09Tb3+,0.005Mn4+ at different temperatures. | |
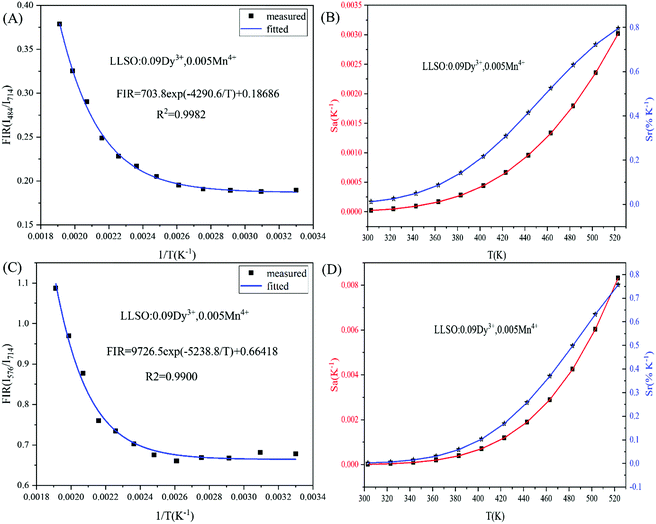 |
| Fig. 9 FIR values, Sr and Sa values of La2LiSbO6:0.09Dy3+,0.005Mn4+ at different temperatures. | |
The values of Sa and Sr are calculated and shown in Fig. 8. The maximum values of Sa and Sr of different luminescent centers are shown in Table 3. Similarly, FIR values between Dy3+ and Mn4+ ions and corresponding Sa and Sr values of different luminous centers at different temperatures are shown in Fig. 9, and their maximum Sa and Sr values are shown in Table 3. Obviously, with the increase of temperature, the relative temperature sensitivity and absolute temperature sensitivity are gradually improved, reaching the maximum at 523 K. The maximum Sa and Sr of Tb3+, Mn4+ co-doped LLSO phosphor appeared in the 591 nm/714 nm luminescence centers, which were 0.00193 K−1 and 0.946%K−1, respectively. The maximum Sa and Sr of Dy3+, Mn4+ co-doped LLSO phosphor appeared in the 576 nm/714 nm luminescence centers, which were 0.00302 K−1 and 0.796% K−1, respectively. The energy difference between Tb3+ (591 nm)/Dy3+ (576 nm) ions and Mn4+ (714 nm) ions is about 2900 cm−1 and 3400 cm−1, which is much larger than the traditional TCLs of rare-earth ions. This indicates that the Sr of the phosphors with double luminescent centers is higher than that of the phosphors based on TCLs, which is traceable. These Sr and Sa are comparable to many other typical co-doped systems listed in Table 4. In addition, temperature resolution (δT) is also an important index to reflect the temperature measurement performance of thermometers, which can be defined as:
|  | (6) |
where ∂Δ/
Δ represents the relative error and its value is about 0.5% in temperature measurement by FIR technology. Because of their excellent relative sensitivity, the minimum resolutions of LLSO:0.09Tb
3+,0.005Mn
4+ phosphors and LLSO:0.09Dy
3+,0.005Mn
4+ phosphors are 0.529 K and 0.628 K, respectively. The peak positions of Mn
4+ and Tb
3+/Dy
3+ are separated, which can provide a good signal discrimination performance. The results show that LLSO: 0.09Tb
3+/Dy
3+, 0.005Mn
4+ samples are suitable for optical thermometers.
Table 3 The maximum Sa and Sr values of La2LiSbO6:0.09Tb3+/Dy3+, 0.005Mn4+at different luminous centers
Doped ions |
Luminous centers (nm) |
Maximum Sa (K−1) |
Maximum Sr (% K−1) |
Tb3+/Mn4+ |
493/714 |
0.000924 |
0.820 |
Tb3+/Mn4+ |
544/714 |
0.00175 |
0.686 |
Tb3+/Mn4+ |
591/714 |
0.00193 |
0.946 |
Dy3+/Mn4+ |
484/714 |
0.00302 |
0.796 |
Dy3+/Mn4+ |
576/714 |
0.00832 |
0.757 |
Table 4 Temperature sensing performances of several typical temperature sensors
Hosts |
Doped ions |
Temperature range (K) |
Excitation wavelength (nm) |
S
r (%K−1) |
S
a (K−1) |
Ref. |
LaOBr |
Ce3+, Tb3+ |
293–433 |
350 |
0.42 |
— |
46
|
LiNbO3 |
Yb3+, Tm3+ |
323–773 |
980 |
0.7 |
0.0088 |
47
|
Sr3GdNa(PO4)3F |
Tb3+, Eu3+ |
303–483 |
275 |
0.16 |
0.00234 |
48
|
K3LuF6 |
Yb3+, Er3+ |
300–773 |
980 |
0.376 |
— |
49
|
Na3Sc2P3O12 |
Eu2+, Mn2+ |
293–473 |
340 |
1.556 |
— |
50
|
SrY2O4 |
Bi3+, Eu3+ |
313–563 |
330 |
0.86 |
0.0433 |
51
|
Ca2YZr2Al3O12 |
Bi3+, Eu3+ |
297–573 |
278 |
0.66 |
0.00826 |
52
|
BaLaMgNbO6 |
Dy3+, Mn4+ |
230–470 |
355 |
1.82 |
— |
53
|
Lu3Al5O12 |
Tb3+, Mn4+ |
270–420 |
355 |
2.8 |
0.043 |
54
|
Ca2LaNbO6 |
Eu3+, Mn4+ |
298–498 |
396 |
1.51 |
0.0399 |
55
|
NaLaMgWO6 |
Eu3+, Mn4+ |
303–523 |
320 |
0.86 |
0.0302 |
41
|
La2LiSbO6 |
Tb3+, Mn4+ |
303–523 |
276 |
0.946 |
0.00193 |
This work |
La2LiSbO6 |
Dy3+, Mn4+ |
303–523 |
274 |
0.796 |
0.00302 |
This work |
4. Conclusion
In all, we have successfully constructed a dual-luminescent system of Tb3+/Dy3+, Mn4+ co-doped antimonate by using a green synthesis method, which is based on the site-beneficial occupation principle. When Tb3+/Dy3+ ions and Mn4+ ions enter the matrix, they will occupy different cation sites, in which Mn4+ ions will replace Sb5+ sites in the matrix, and Tb3+/Dy3+ ions can be doped into La3+ sites. By analyzing their luminescence spectra, the emission of Tb3+/Dy3+ ions and Mn4+ ions can be simultaneously observed under excitation at 276 nm or 274 nm and the intensity is very ideal. On this basis, their possible luminescence mechanism is speculated.
Because of the different thermal quenching behavior between RE ions and TM ions, the emission intensity of Mn4+ is much faster than that of Tb3+ and Dy3+ ions with the increase in temperature. Therefore, Tb3+/Dy3+ ions can be used as reference signals, whereas Mn4+ ions are suitable detection signals. The fluorescence intensity ratio (FIR) of Tb3+/Dy3+ ions and Mn4+ ions are significantly affected by temperature. Therefore, the material exhibits excellent temperature measurement performance in the temperature range of 303–523 K. Based on FIR technology, the maximum relative sensitivity and absolute sensitivity of Tb3+ and Mn4+ co-doped LLSO phosphors are 0.946% K−1 (523 K) and 0.00193 K−1, respectively; the maximum relative sensitivity and absolute sensitivity of Dy3+ and Mn4+ co-doped LLSO phosphors are 0.796% K−1 and 0.00832 K−1, respectively, which are much higher than those of some optical thermometric materials reported previously. In addition, the emission peaks of Tb3+/Dy3+ ions and Mn4+ ions are well separated, which provides a good signal identification ability for temperature detection. This work hews out a new strategy to develop high-performance optical temperature sensing materials that have extensive application potentiality in non-contact temperature detection.
Conflicts of interest
There are no conflicts of interest to declare.
Acknowledgements
This work is financially supported by the National Natural Science Foundation of China (Grant No. 21401130); the Opening Research Fund of the State Key Laboratory of Rare Earth Resource Utilization, Changchun Institute of Applied Chemistry, Chinese Academy of Sciences (RERU2014005).
References
- D. Peng, B. Chen and F. Wang, Recent Advances in Doped Mechanoluminescent Phosphors, ChemPlusChem, 2015, 80, 1209–1215 CrossRef CAS.
- Q. Ju, D. Tu, Y. Liu, R. Li, H. Zhu, J. Chen, Z. Chen, M. Huang and X. Chen, Amine-Functionalized Lanthanide-Doped KGdF4 Nanocrystals as Potential Optical/Magnetic Multimodal BioProbes, J. Am. Chem. Soc., 2012, 134, 1323–1330 CrossRef CAS.
- M. Shang, S. Huang and J. Lin, Multicolor emissions and photoluminescence properties for Ca3Al4ZnO10: Ce3+/Eu3+/Tb3+/Mn2+ phosphors, J. Lumin., 2018, 204, 493–498 CrossRef CAS.
- M. Chen, Z. Xia and Q. Liu, Ethylenediamine-Assisted Hydrothermal Synthesis of NaCaSiO3OH: Controlled Morphology, Mechanism, and Luminescence Properties by Doping Eu3+/Tb3+, Inorg. Chem., 2016, 55, 11316–11322 CrossRef CAS.
- Q. Zeng, P. He, H. Liang, M. Gong and Q. Su, Luminescence of Eu3+-activated tetra-molybdate red phosphors and their application in near-UV InGaN-based LEDs, Mater. Chem. Phys., 2009, 118, 76–80 CrossRef CAS.
- L. Wang, R.-J. Xie, Y. Li, X. Wang, C.-G. Ma, D. Luo, T. Takeda, Y.-T. Tsai, R.-S. Liu and N. Hirosaki, Ca1−xLixAl1−xSi1+xN3:Eu2+ solid solutions as broadband, color-tunable and thermally robust red phosphors for superior color rendition white light-emitting diodes, Light: Sci. Appl., 2016, 5, e16155 CrossRef CAS.
- L. Feng, Z. Hao, X. Zhang, L. Zhang, G. Pan, Y. Luo, L. Zhang, H. Zhao and J. Zhang, Red emission generation through highly efficient energy transfer from Ce3+ to Mn2+ in CaO for warm white LEDs, Dalton Trans., 2016, 45, 1539–1545 RSC.
- C. Wang, Y. Jin, L. Yuan, H. Wu, G. Ju, Z. Li, D. Liu, Y. Lv, L. Chen and Y. Hu, A spatial/temporal dual-mode optical thermometry platform based on synergetic luminescence of Ti4+–Eu3+ embedded flexible 3D micro-rod arrays: High-sensitive temperature sensing and multi-dimensional high-level secure anti-counterfeiting, Chem. Eng. J., 2019, 374, 992–1004 CrossRef CAS.
- L. Li, X. Tang, Z. Wu, Y. Zheng, S. Jiang, X. Tang, G. Xiang and X. Zhou, Simultaneously tuning emission color and realizing optical thermometry via efficient Tb3+ → Eu3+ energy transfer in whitlockite-type phosphate multifunctional phosphors, J. Alloys Compd., 2019, 780, 266–275 CrossRef CAS.
- Z. Cao, S. Zhou, G. Jiang, Y. Chen, C. Duan and M. Yin, Temperature dependent luminescence of Dy3+ doped BaYF5 nanoparticles for optical thermometry, Curr. Appl. Phys., 2014, 14, 1067–1071 CrossRef.
- W. Dai, J. Hu, G. Liu, S. Xu, K. Huang, J. Zhou and M. Xu, Thermometer of stable SrAl2Si2O8: Ce3+, Tb3+ based on synergistic luminescence, J. Lumin., 2020, 217, 116807 CrossRef CAS.
- B. Dong, D. P. Liu, X. J. Wang, T. Yang, S. M. Miao and C. R. Li, Optical thermometry through infrared excited green upconversion emissions in Er3+–Yb3+ codoped Al2O3, Appl. Phys. Lett., 2007, 90, 181117 CrossRef.
- Y. Fu, L. Zhao, Y. Guo and H. Yu, A transparent and dual-functional oxyfluoride glass ceramics with color-tunable up-conversion luminescence and high thermosensitivity, J. Lumin., 2020, 217, 116807 CrossRef.
- H. Zhang, Z. Gao, G. Li, Y. Zhu, S. Liu, K. Li and Y. Liang, A ratiometric optical thermometer with multi-color emission and high sensitivity based
on double perovskite LaMg0.402Nb0.598O3: Pr3+ thermochromic phosphors, Chem. Eng. J., 2020, 380, 122491 CrossRef CAS.
- Z. Xing, P. Li, S. Wu, C. Liu, D. Dai, X. Li, L. Zhang, D. Wang, Z. Yang and Z. Wang, A perovskite-like LaSrGaO4: Mn2+, Nd3+, Y3+NIR luminescent material for fluorescent temperature sensor, J. Lumin., 2020, 225, 117352 CrossRef CAS.
- E. J. McLaurin, L. R. Bradshaw and D. R. Gamelin, Dual-Emitting Nanoscale Temperature Sensors, Chem. Mater., 2013, 25, 1283–1292 CrossRef CAS.
- X. Wang, Q. Liu, Y. Bu, C.-S. Liu, T. Liu and X. Yan, Optical temperature sensing of rare-earth ion doped phosphors, RSC Adv., 2015, 5, 86219–86236 RSC.
- J. Tang, P. Du, W. Li and L. Luo, Boosted thermometric performance in NaGdF4:Er3+/Yb3+ upconverting nanorods by Fe3+ ions doping for contactless nanothermometer based on thermally and non-thermally coupled levels, J. Lumin., 2020, 224, 117296 CrossRef CAS.
- P. Kaur, A. Khanna, M. N. Singh and A. K. Sinha, Structural and optical characterization of Eu and Dy doped CaWO4 nanoparticles for white light emission, J. Alloys Compd., 2020, 834, 154804 CrossRef CAS.
- J. Huang, L. Tang, N. Chen and G. Du, Broadening the Photoluminescence Excitation Spectral Bandwidth of YVO4:Eu3+ Nanoparticles via a Novel Core–Shell and Hybridization Approach, Materials, 2019, 12, 3830 CrossRef CAS.
- X. Miao, Z. Bai, G. Qiu, S. Tang, M. Guo, F. Cheng and M. Zhang, Preparation of transparent Mn-doped CaF2 glass-ceramics from silicon-manganese slag: Dependence of colour-controllable change on slag addition and crystallization behavior, J. Eur. Ceram. Soc., 2020, 40, 3249–3261 CrossRef CAS.
- T. Wang, S. Wang, H. Zhang, X. Zou and W. Hu, Tm3+–Dy3+–Eu3+ tri-doped transparent glass-ceramics containing NaY(MoO4)(2) crystal phase: Preparation, energy transfer, warm white light emitting, Opt. Mater., 2020, 104, UNSP 109851 CrossRef.
- B. R. Reddy, I. Kamma and P. Kommidi, Optical sensing techniques for temperature measurement, Appl. Opt., 2013, 52, B33–B39 CrossRef CAS.
- S. F. Collins, G. W. Baxter, S. A. Wade, T. Sun, K. T. V. Grattan, Z. Y. Zhang and A. W. Palmer, Comparison of fluorescence-based temperature sensor schemes: theoretical analysis and experimental validation, J. Appl. Phys., 1998, 84, 4649–4654 CrossRef CAS.
- V. Dubey, S. Agrawal and J. Kaur, Photoluminescence and thermoluminescence behavior of Gd doped Y2O3 phosphor, Optik, 2015, 126, 1–5 CrossRef CAS.
- M. Benabdesselam, F. Mady and S. Girard, Assessment of Ge-doped optical fibre as a TL-mode detector, J. Non-Cryst. Solids, 2013, 360, 9–12 CrossRef CAS.
- Z. Zhou, J. Zheng, R. Shi, N. Zhang, J. Chen, R. Zhang, H. Suo, E. M. Goldys and C. Guo,
Ab Initio Site Occupancy and Far-Red Emission of Mn4+ in Cubic-Phase La(MgTi)1/2O3 for Plant Cultivation, ACS Appl. Mater. Interfaces, 2017, 9, 6177–6185 CrossRef CAS.
- M. Sekulic, Z. Ristic, B. Milicevic, Z. Antic, V. Dordevic and M. D. Dramicanin, Li1.8Na0.2TiO3: Mn4+: the highly sensitive probe for the low-temperature lifetime-based luminescence thermometry, Opt. Commun., 2019, 452, 342–346 CrossRef CAS.
- I. E. Kolesnikov, A. A. Kalinichev, M. A. Kurochkin, E. Y. Kolesnikov and E. Lahderanta, Porphyrins as efficient ratiometric and lifetime-based contactless optical thermometers, Mater. Des., 2019, 184, 108188 CrossRef CAS.
- S. A. Wade, S. F. Collins and G. W. Baxter, Fluorescence intensity ratio technique for optical fiber point temperature sensing, J. Appl. Phys., 2003, 94, 4743 CrossRef CAS.
- D. He, C. Guo, S. Jiang, N. Zhang, C. Duan, M. Yin and T. Li, Optical temperature sensing properties of Yb3+–Er3+ co-doped NaLnTiO4 (Ln = Gd, Y) up-conversion phosphors, RSC Adv., 2015, 5, 1385–1390 RSC.
- D. Wawrzynczyk, A. Bednarkiewicz, M. Nyk, W. Strek and M. Samoc, Neodymium(III) doped fluoride nanoparticles as non-contact optical temperature sensors, Nanoscale, 2012, 4, 6959–6961 RSC.
- L. Zhao, Z. Cao, X. Wei, M. Yin and Y. Chen, Luminescence properties of Eu3+ doped YBO3 for temperature sensing, J. Rare Earths, 2017, 35, 356–360 CrossRef CAS.
- Z. Liang, F. Qin, Y. Zheng, Z. Zhang and W. Cao, Noncontact thermometry based on downconversion luminescence from Eu3+ doped LiNbO3 single crystal, Sens. Actuators, A, 2016, 238, 215–219 CrossRef CAS.
- Y. Ma, G. Xiang, J. Zhang, Z. Liu, P. Zhou, W. Liu, X. Tang, S. Jiang, X. Zhou, L. Li, Y. Luo and Y. Jin, Upconversion properties and temperature sensing behaviors in visible and near-infrared region based on fluorescence intensity ratio in LuVO4: Yb3+/Er3+, J. Alloys Compd., 2018, 769, 325–331 CrossRef CAS.
- K. Li, D. Zhu and H. Lian, Up-conversion luminescence and optical temperature sensing properties in novel KBaY(MoO4)(3): Yb3+, Er3+ materials for temperature sensors, J. Alloys Compd., 2020, 816, 152554 CrossRef CAS.
- H. Zhang, H. Zhang, B. Lei, N. Lai, X. Lin, H. Lu, Q. Lan and Y. Liu, High Temperature Solid-State Synthesis and Luminescent Properties of Sr2MgAl22O36:Mn4+, Rare Met. Mater. Eng., 2016, 45, 448–452 CrossRef.
- X. Huang, Q. Sun and B. Devakumar, Novel efficient deep-red-emitting Ca2LuTaO6:Mn4+ double-perovskite phosphors for plant growth LEDs, J. Lumin., 2020, 222, 117177 CrossRef CAS.
- M. Liu, P. Dang, Y. Wei, S. Liang, G. Liu, A. Geng, H. Lian and J. Lin, Comparative analysis on the photoluminescence properties of Cs2BF6:Mn4+ (B = Ge, Si, Zr, Ti) red phosphors for WLEDs, J. Am. Ceram. Soc., 2020, 103, 1197–1208 CrossRef CAS.
- Y. Song, N. Guo, J. Li, R. Ouyang, Y. Miao and B. Shao, Photoluminescence and temperature sensing of lanthanide Eu3+ and transition metal Mn4+ dual-doped antimoniate phosphor through site-beneficial occupation, Ceram. Int., 2020, 46, 22164–22170 CrossRef CAS.
- H. Zhou, N. Guo, M. Zhu, J. Li, Y. Miao and B. Shao, Photoluminescence and ratiometric optical thermometry in Mn4+/Eu3+ dual-doped phosphor via site-favorable occupation, J. Lumin., 2020, 224, 117311 CrossRef CAS.
- M. L. López, M. L. Veiga, A. Jerez and C. Pico, Synthesis and crystal structure of La2LiSbO6, Mater. Rer. Bull., 1990, 25, 1271–1277 CrossRef.
- A. M. Pires and M. R. Davolos, Luminescence of europium(III) and manganese(II) in barium and zinc orthosilicate, Chem. Mater., 2001, 13, 21–27 CrossRef CAS.
- X. Wang, Z. Zhao, Q. Wu, Y. Li and Y. Wang, A Garnet-Based Ca2YZr2Al3O12: Eu3+ Red-Emitting Phosphor for n-UV Light Emitting Diodes and Field Emission Displays: Electronic Structure and Luminescence Properties, Inorg. Chem., 2016, 55, 11072–11077 CrossRef CAS.
- Y. Gao, F. Huang, H. Lin, J. Zhou, J. Xu and Y. Wang, A Novel Optical Thermometry Strategy Based on Diverse
Thermal Response from Two Intervalence Charge Transfer States, Adv. Funct. Mater., 2016, 26, 3139–3145 CrossRef CAS.
- X. Zhang, Y. Huang and M. Gong, Dual-emitting Ce3+, Tb3+ co-doped LaOBr phosphor: luminescence, energy transfer and ratiometric temperature sensing, Chem. Eng. J., 2017, 307, 291–299 CrossRef CAS.
- L. Xing, R. Ao, Y. Liu and W. Yang, Optical thermometry based on the non-thermally coupled levels of Tm(III) in LiNbO3 crystals, Spectrochim. Acta, Part A, 2019, 222, 117159 CrossRef CAS.
- N. Guo, Y. Pan, W. Lv, R. Ouyang and B. Shao, Optical thermometric properties in Tb3+ and Eu3+ -coactivated dual-emissive fluorophosphate phosphors, Opt. Laser Technol., 2020, 123(105938) CAS.
- J. Cao, F. Hu, L. Chen, H. Guo, C. Duan and M. Yin, Wide-range thermometry based on green up-conversion luminescence of K3LuF6:Yb3+/Er3+ bulk oxyfluoride glass ceramics, J. Am. Ceram. Soc., 2017, 100, 2108–2115 CrossRef CAS.
- X. Zhang, Z. Zhu, Z. Guo, Z. Sun and Y. Chen, A ratiometric optical thermometer with high sensitivity and superior signal discriminability based on Na3Sc2P3O12: Eu2+, Mn2+ thermochromic phosphor, Chem. Eng. J., 2019, 356, 413–422 CrossRef CAS.
- R. Wei, J. Guo, K. Li, L. Yang, X. Tian, X. Li, F. Hu and H. Guo, Dual-emitting SrY2O4: Bi3+, Eu3+ phosphor for ratiometric temperature sensing, J. Lumin., 2019, 216, 116737 CrossRef CAS.
- Z. Zheng, J. Zhang, X. Liu, R. Wei, F. Hu and H. Guo, Luminescence and self-referenced optical temperature sensing performance in Ca2YZr2Al3O12: Bi3+,Eu3+ phosphors, Ceram. Int., 2020, 46, 6154–6159 CrossRef CAS.
- Y. Lin, L. Zhao, B. Jiang, J. Mao, F. Chi, P. Wang, C. Xie, X. Wei, Y. Chen and M. Yin, Temperature-dependent luminescence of BaLaMgNbO6: Mn4+, Dy3+ phosphor for dual-mode optical thermometry, Opt. Mater., 2019, 95, UNSP 109199 CrossRef.
- C. Xie, L. Zhao, B. Jiang, J. Mao, Y. Lin, P. Wang, X. Wei, M. Yin and Y. Chen, Dual-activator luminescence of LuAG: Mn4+/Tb3+ phosphor for optical thermometry, J. Am. Ceram. Soc., 2019, 102, 7500–7508 CrossRef CAS.
- P. Wang, J. Mao, L. Zhao, B. Jiang, C. Xie, Y. Lin, F. Chi, M. Yin and Y. Chen, Double perovskite A2LaNbO6: Mn4+, Eu3+ (A = Ba, Ca) phosphors: potential applications in optical temperature sensing, Dalton Trans., 2019, 48, 10062–10069 RSC.
Footnote |
† Electronic supplementary information (ESI) available. See DOI: 10.1039/d0ma00841a |
|
This journal is © The Royal Society of Chemistry 2021 |
Click here to see how this site uses Cookies. View our privacy policy here.