DOI:
10.1039/D0GC03818K
(Paper)
Green Chem., 2021,
23, 546-551
Photochemical metal-free aerobic oxidation of thiols to disulfides†
Received
11th November 2020
, Accepted 9th December 2020
First published on 9th December 2020
Abstract
Thiol oxidation to disulfides is an area of great importance in organic synthesis, both for synthetic and biological purposes. Herein, we report a mild, inexpensive and green photochemical approach for the synthesis of both symmetrical and non-symmetrical disulfides, using metal-free and environmentally friendly conditions. Utilizing phenylglyoxylic acid as the photoinitiator, common household bulbs as the light source and a simple inorganic salt as the additive, a versatile oxidation of thiols leading to products in excellent yields is described.
Introduction
Sulfur compounds, although usually associated with a foul smell, are considered non-toxic and can be found in a variety of natural products (Fig. 1). For example, sulforaphane is a key ingredient of the Brassicaceae family, that can be found in broccoli and cabbages that possesses anticancer activity, due to its isothiocyanate moiety.1 Among compounds containing sulfur, disulfides play a dominant role in biological systems, since they are fundamental factors in protein folding and oligomerization, as they stabilize the 3D structure and affect their biological function.2 Among the most common natural structures bearing a disulfide “bridge” is cystine, which is formed from the oxidation of cysteine, and insulin (Fig. 1). Disulfides are also important intermediates for many synthetic pathways and present various applications in agro-chemicals and pharmaceuticals.3,4 The latest global turmoil, regarding the pandemic caused by COVID-19, forced the scientific community to work intensively, in order to decode as fast as possible the structure and the function of the novel coronavirus. In this direction, researchers found that the spike protein of SARS-Cov-2019, which is the receptor-binding domain (RBD) to the ACE2 receptor of the host cell, consists of cysteine residues that form pairs with disulfide bonds.5 In fact, some of the latest studies confirm the importance of thiol–disulfide balance in the binding affinity of the spike protein to the ACE2 receptor.6 Furthermore, among the potential inhibitors of SARS-Cov-2019 protein are molecules that possess as a key unit a non-symmetrical disulfide.5e These findings render disulfides, as moieties of great importance and synthetic interest.
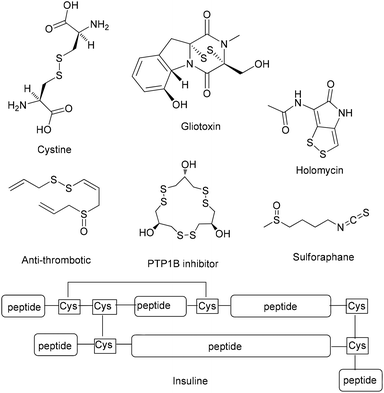 |
| Fig. 1 Sulfur compounds of biological importance. | |
Due to their importance, a plethora of synthetic methods to access disulfides has been reported, with most of them utilizing thiols as the starting materials. In many cases, the aerial oxidation of thiols can be catalyzed by metals or other frequently-used oxidation reagents, such as iodine, hydrogen peroxide, copper nanoparticles, bromine, N-bromo-derivatives, nitrogen-containing oxidants, diethyl azodicarboxylate, sulfuryl chloride, peroxymonosulfate, peroxydisufate, miscellaneous reagents and chromates (Scheme 1A).7
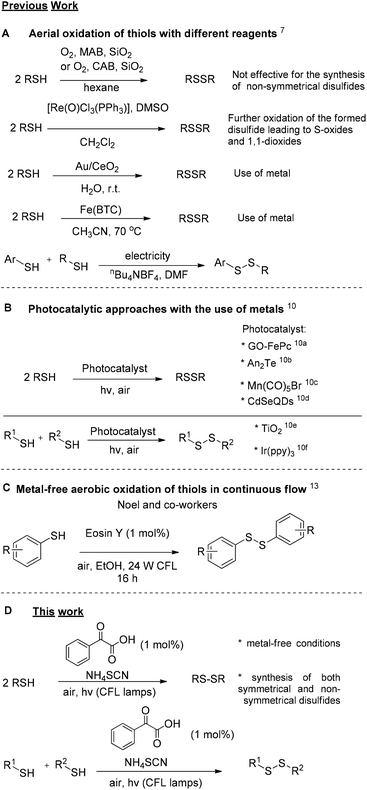 |
| Scheme 1 Approaches for the synthesis of disulfides from thiols. | |
An alternative approach for the oxidative coupling of thiols is the use of visible-light to achieve organic transformations. The increasing need for milder reactions conditions, cheaper and eco-friendlier oxidants led many researchers to discover new synthetic pathways based on photoredox catalysis, the use of visible light along with a photocatalyst to promote organic transformations.8,9 Basic feature in most photoredox reactions is the use of metal complexes as the photocatalyst. Photoredox catalysis also provided elegant approaches for the oxidation of thiols to disulfides (Scheme 1B).10 In all these cases, the authors postulate the importance of oxygen as the environmentally friendly and low-cost oxidant. A far cheaper approach and an alternative to photoredox catalysis is the introduction of photoorganocatalysis, the use of small organic molecules as photocatalysts.11,12 Exploring this promising field, Noel and co-workers introduced a continuous flow process, enabling a metal-free aerobic oxidation of thiols to disulfides, utilizing Eosin Y, an organic dye, as the photocatalyst and visible-light irradiation (Scheme 1C).13
Very recently, our group introduced in literature a photochemical protocol that is easy to operate employing cheap household bulbs as the source of irradiation.14 In an effort to expand this photochemical protocol, we envisaged the use of phenylglyoxylic acid as the photoinitiator for the oxidation of various thiols to disulfides (Scheme 1, bottom). This protocol is a green metal-free alternative to transition-metal photoredox catalysis.
Results and discussion
We initially investigated the oxidation of benzyl mercaptan (1a) (Scheme 2). A variety of commercially available photoinitiators (3a–l) can promote the reaction. Phenylglyoxylic acid (3a) outperformed all others and proved the suitable photoinitiator, providing the product in quantitative yield (Scheme 2). The progress of the reaction was also monitored by GS-MS and the oxidation of thiol was completed after 7 h. If the reaction was performed in the dark, or no catalyst was added, no reaction is taking place, constituting indispensable the use of both light and catalyst. Traces of product were also formed, when the reaction was performed under dark at 50 °C.
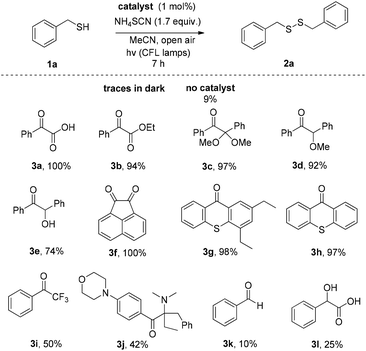 |
| Scheme 2 Initiator screening for the photochemical synthesis of disulfide 2a. | |
Then, our interest was shifted towards the study of the reaction conditions (Table 1).15 After optimization,15 acetonitrile was found to be an excellent solvent (Table 1, entries 1–7). It has to be highlighted the important role of ammonium thiocyanate, since its absence led to diminished yields (Table 1, entry 7). Other additives were also tested (Table 1, entries 8–12). It seems that the presence of a salt containing either a −SCN or −CN is necessary, along with a K+, Na+ or NH4+ counterion. Under argon atmosphere, the reaction yield decreased (Table 1, entry 13). Thus, a green and sustainable protocol for the synthesis of disulfides from thiols, is introduced, where phenylglyoxylic acid is used as the initiator and cheap household bulbs can be employed as the light source.
Table 1 Optimization of the reaction conditions for the oxidation of thiols to disulfides
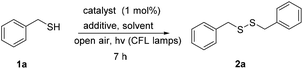
|
Entry |
Solvent |
Additive (1.7 equiv.) |
Yielda (%) |
Yield determined by GC-MS.
Under an Ar atmosphere.
|
1 |
CH2Cl2 |
NH4SCN |
19 |
2 |
THF |
NH4SCN |
41 |
3 |
Pet. ether |
NH4SCN |
15 |
4 |
AcOEt |
NH4SCN |
54 |
5 |
MeOH |
NH4SCN |
93 |
6 |
MeCN |
NH4SCN |
100 |
7 |
MeCN |
— |
44 |
8 |
MeCN |
KCl |
15 |
9 |
MeCN |
NH4Cl |
22 |
10 |
MeCN |
PhSCN |
30 |
11 |
MeCN |
KSCN |
95 |
12 |
MeCN |
Na2HPO4 |
5 |
13b |
MeCN |
NH4SCN |
24 |
Having established the optimum reaction conditions, we turned our attention in the exploration of the substrate scope of the reaction. First, a series of thiols were tested for the synthesis of symmetrical disulfides (Scheme 3). We realized that both aryl and alkyl thiols underwent disulfide formation in good to excellent yields. Various substitution patterns on the aromatic moiety were well tolerated (para-, ortho- and meta-substitution on electron withdrawing or donating groups), leading to products in mediocre to satisfactory yields (2a–o). The similar behaviour of different substrates under the optimized reaction conditions demonstrates the general applicability of this protocol, while this method allows us to encompass many different functional groups, such as –NO2, –COOH, protected –NH2, –OH, RCOO−, RO− or –Cl. Apart from the success in para-, meta- and ortho-substituted substrates, it has also been possible to obtain successful results with aliphatic thiols or even with stereochemically hindered substrates. It has to be highlighted that in some cases, simple aqueous wash was enough to deliver the product with enough purity, not requiring further purification.15
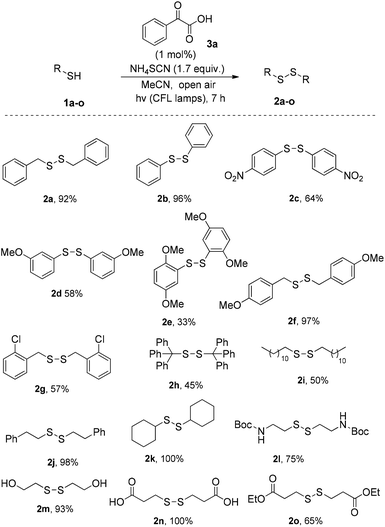 |
| Scheme 3 Substrate scope of the photochemical synthesis of symmetrical disulfides. | |
Encouraged from these results, we anticipated that our method would be suitable for the synthesis of non-symmetrical disulfides (Scheme 4). In an effort to control the chemoselectivity of the reaction, a mixture of 2 equiv. of a thiol bearing polar groups and 1 equiv. of a simple thiol was treated under the optimised reaction conditions. In this manner, the battle between the symmetrical and non-symmetrical products was reflected on the yields. We noticed that thiols that oxidised very well previously, do not present the same chemical behaviour as the half-part of a non-symmetrical disulfide. However, the fact that non-symmetrical disulfide formation is rarely described in literature and the metal-free conditions used, herein, constitute this green synthetic pathway very effective. Even low yields in some cases, can be admissible, in order to avoid the use of metals.
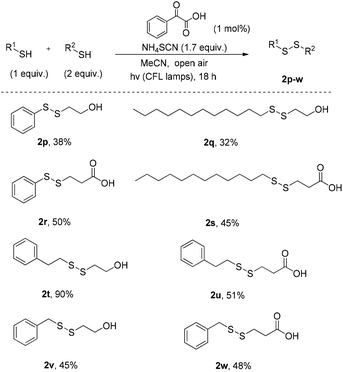 |
| Scheme 4 Substrate scope of the photochemical synthesis of non-symmetrical disulfides. | |
We then decided to decipher the reaction mechanism. Fluorescence quenching experiments with PhCOCO2H were initially performed.15 Interestingly, the fluorescence of PhCOCO2H did not decrease, when an increasing amount of thiol or NH4SCN alone, were added. However, when a 1
:
1 mixture of thiol
:
NH4SCN was added, the fluorescence was decreased.15 This confirms the important role of ammonium thiocyanate and postulates that either NH4SCN forms a salt with PhCOCO2H and its fluorescence is decreased by thiol or that the thiol forms a salt with NH4SCN, which decreases the fluorescence of PhCOCO2H or its salt. Then, UV-Vis were performed.15 The association of an electron rich molecule with an electron poor compound can lead to the formation of a new aggregate, called electron donor–acceptor (EDA) complex. In some cases, upon addition of the two components of the EDA complex, an increase in the UV absorbance of the mixture is observed. In our case, upon mixing the various reaction components, no remarkable increase in the UV absorbance was observed, rejecting the possibility of an EDA complex formation.
The reaction was also probed by the use of NMR spectroscopy. Phenylglyoxylic acid photodecomposed slowly to benzaldehyde, when irradiated alone in MeCN, obviously via a benzoyl radical.15 This photodecomposition was faster and more in quantity, when NH4SCN was present, which shows that the salt of PhCOCO2H with NH4SCN, photodecomposes faster to benzaldehyde than PhCOCOOH alone. Salt formation between thiol and NH4SCN was also evident. Finally, in the reaction mixture, the photodecomposition to benzaldehyde was also observed.15 We also performed a number of mechanistic experiments with a number of probes (Table 2). Obviously, this is a radical process (Table 2, entries 1 and 2). Interestingly, the use of CuCl2 (Table 2, entry 3) verified the fluorescence quenching experiments that a SET mechanism is contributing in a minor extend (if any) for the reaction outcome. Finally, oxygen species are taking part in the reaction (Table 2, entries 4–7).
Table 2 Mechanistic studies on the photochemical oxidation of benzyl mercaptan
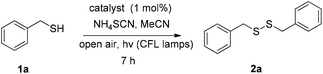
|
Entry |
Quencher (equiv.) |
Notes |
Yielda (%) |
Yield determined by GC-MS.
|
1 |
BHT (1.0) |
Radical scavenger |
0 |
2 |
TEMPO (1.0) |
Radical scavenger |
0 |
3 |
CuCl2 (1.0) |
Electron scavenger |
87 |
4 |
DABCO (1.0) |
Singlet oxygen scavenger |
30 |
5 |
NaN3 (1.0) |
Singlet oxygen scavenger |
10 |
6 |
Benzoquinone (1.0) |
Superoxide radical anion scavenger |
37 |
7 |
Ar atmosphere |
— |
24 |
In 2015, Cismesia and Yoon introduced the quantum yield measurement as a mechanistic tool.16 A closed photocatalytic system, which lacks chain propagation, exhibits a maximum theoretical quantum yield of Φ = 1. Open chain processes in principle can provide multiple equivalents of product from each photon absorbed, therefore the quantum yield of a chain reaction is Φ ≫ 1. We calculated the quantum yield of the reaction (Φ = 38), indicating a chain propagation mechanism.15
Based on the above experiments, a plausible reaction mechanism can be proposed (Scheme 5). Upon irradiation, phenylglyoxylic acid in MeCN is slowly photodecomposed to benzaldehyde via benzoyl radical I (Scheme 5, top left). If NH4SCN is present, salt formation with PhCOCO2H leads to a faster photodecomposition to benzaldehyde via benzoyl radical I (Scheme 5, top right). Then, one can envisage a number of potential pathways that can be followed (Scheme 5, bottom). There is a possibility that excited PhCOCO2H or its salt can lead to singlet oxygen generation via energy transfer, which is known in literature to interact with thiols and initiate disulfide formation (Scheme 5, bottom far right).10 However, PhCOCO2H is a poor singlet oxygen generator, according to our previous studies,14l and thus this pathway must not be very productive in this method. On the other hand, benzoyl radical I, generated either by excited PhCOCOOH or the salt of PhCOCOOH with NH4SCN, can initiate a hydrogen atom transfer (HAT) with the thiol, leading to thiyl radical II (Scheme 5, bottom right). Then, via reaction with another thiol and oxygen, disulfide is formed, along with HO2˙ (III). III can propagate the reaction via HAT with the thiol. This is probably the major pathway followed in our method, that can account for the reactivity without the additive as well. Similarly, thiyl radical II can react with the salt of thiol and NH4SCN, in the presence of oxygen, and via a less likely SET (single electron transfer) affords the product and superoxide anion IV (Scheme 5, bottom left). Superoxide anion can propagate the reaction via HAT with the thiol. This is probably a minor pathway in our method, since the use of an electron scavenger reduced the yield of the methodology only marginally. Finally, salt formation between thiol and NH4SCN leads to RS− (V) (Scheme 5, bottom far left). This reacts with II leading to VI.13 This is already postulated in literature that reacts with oxygen via a SET event, leading to product and superoxide radical IV, which as previously, can propagate the reaction.
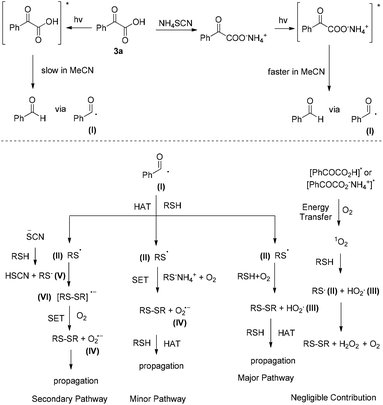 |
| Scheme 5 Proposed reaction mechanism for the photochemical aerobic oxidation of thiols to disulfides using phenylglyoxylic acid as the photoinitiator. | |
Conclusions
In conclusion, a simple, cheap and environmentally friendly photochemical protocol was developed and successfully applied to the oxidation of aryl and alkyl thiols to symmetrical and non-symmetrical disulfides. Bypassing the need for transition metal complexes or organic dyes, and thermal (photo)initiators, this method relies on a small organic molecule and cheap household bulbs. Phenylglyoxylic acid can be employed in a very low catalyst loading (1 mol%), leading to products in good to excellent yields. The mechanism of the reaction was also studied.
Experimental
General procedure for the synthesis of disulfides
In a glass vial containing phenylglyoxylic acid (0.8 mg, 0.005 mmol) and ammonium thiocyanate (65 mg, 0.85 mmol) in acetonitrile (2 mL), thiol (0.50 mmol) was added. The reaction mixture was irradiated on open air with 2 × 85 W household bulbs with vigorous stirring for 7 h. The desired product was isolated either after dilution with CH2Cl2 (5 mL), wash with 10% aq. NaHCO3 (2 × 5 mL) and the organic layer was dried over Na2SO4 and the solvent was removed in vacuo or after purification by column chromatography.
Author contributions
Conceptualization: C. G. K.; reaction optimization, substrate scope and compound characterization: N. S.; mechanistic studies: N. S. and C. G. K.; writing original draft: N. S. and C. G. K.; writing, reviewing and editing: C. G. K.; supervision and project administration: C. G. K.; funding acquisition: N. S. and C. G. K.
Conflicts of interest
The authors declare no conflicts.
Acknowledgements
The authors gratefully acknowledge the Hellenic Foundation for Research and Innovation (HFRI) for financial support through a grant, which is financed by 1st Call for H.F.R.I. Research Projects to Support Faculty Members & Researchers and the procurement of high-cost research equipment grant (grant number 655). N.S. would like to thank the Hellenic Foundation for Research and Innovation (HFRI) for financial support through a doctoral by the Hellenic Foundation for Research and Innovation (HFRI) under PhD Fellowship grant (Fellowship Number: 721). Also, COST Action C–H Activation in Organic Synthesis (CHAOS) CA15106 is acknowledged for helpful discussions.
Notes and references
- M. G. Kokotou, P. K. Revelou, C. Pappas and V. Constantinou-Kokotou, Food Chem., 2017, 237, 566–573 CrossRef CAS PubMed.
-
(a) E. Gross, C. S. Sevier, A. Vala, C. A. Kaiser and D. Fass, Nat. Struct. Mol. Biol., 2002, 9, 61–67 CrossRef CAS PubMed;
(b) J. R. Winther and C. Thorpe, Biochim. Biophys. Acta, 2014, 1840, 838–846 CrossRef CAS PubMed;
(c) M. Gongora-Bernitez, J. Tulla-Puche and F. Albericio, Chem. Rev., 2014, 114, 901–926 CrossRef PubMed;
(d)
S. Patai, The Chemistry of the Thiol Group, John Willey and Sons, 1974, pp. 113–119 Search PubMed.
- For a review, see:
(a) B. Mandal and B. Basu, RSC Adv., 2014, 4, 13854–13881 RSC. For some recent examples on the use of disulfides in synthesis, see:
(b) V. Pace, A. Pelosi, D. Antermite, O. Rosati, M. Curini and W. Holzer, Chem. Commun., 2016, 52, 2639–2642 RSC;
(c) L. Lelo, V. Pillari, N. Gajic, W. Holzer and V. Pace, Chem. Commun., 2020, 56, 12395–12398 RSC.
-
(a) E. Block, S. Ahmad, M. K. Jain, R. W. Crecely, R. Apitz-Castro and M. R. Cruz, J. Am. Chem. Soc., 1984, 106, 8295–8296 CrossRef CAS;
(b) J.-X. Gong, X. Shen, L.-G. Yao, H. Jiang, K. Krohn and Y.-W. Guo, Org. Lett., 2007, 9, 1715–1716 CrossRef CAS PubMed.
-
(a) I. M. Ibrahim, D. H. Abdelmalek, M. E. Elshahat and A. A. Elfiky, J. Infect., 2020, 80, 554–562 CrossRef CAS PubMed;
(b) J. Yu, S. Qiao, R. Guo and X. Wang, Nat. Commun., 2020, 11, 3070–3081 CrossRef CAS PubMed;
(c) J. Lan, J. Ge, J. Yu, S. Shan, H. Zhou, S. Fan, Q. Zhang, X. Shi, Q. Wang, L. Zhang and X. Wang, Nature, 2020, 581, 215–220 CrossRef CAS PubMed;
(d) D. Wrapp, N. Wang, K. S. Corbett, J. A. Goldsmith, C.-L. Hsieh, O. Abiona, B. S. Graham and J. S. McLellan, Science, 2020, 367, 1260–1263 CrossRef CAS PubMed;
(e) Z. Jin, X. Du, Y. Xu, Y. Deng, M. Liu, Y. Zhao, B. Zhang, X. Li, L. Zhang, C. Peng, Y. Duan, J. Yu, L. Wang, K. Yang, F. Liu, R. Jiang, X. Yang, T. You, X. Liu, X. Yang, F. Bai, H. Liu, X. Liu, L. W. Guddat, W. Xu, G. Xiao, C. Qin, Z. Shi, H. Jiang, Z. Rao and H. Yang, Nature, 2020, 582, 289–293 CrossRef CAS PubMed.
- S. Hati and S. Bhattacharyya, ACS Omega, 2020, 5(26), 16292–16298 CrossRef CAS PubMed.
- For a review, see:
(a) D. Witt, Synthesis, 2008, 2491–2509 CrossRef CAS. For a recent contribution, see:
(b) P. Huang, P. Wang, S. Tang, Z. Fu and A. Lei, Angew. Chem., Int. Ed., 2018, 57, 8115–8119 CrossRef CAS PubMed.
- For selected reviews, see:
(a) C. K. Prier, D. A. Rankic and D. W. C. MacMillan, Chem. Rev., 2013, 113, 5322–5363 CrossRef CAS PubMed;
(b) K. L. Scubi, T. R. Blum and T. P. Yoon, Chem. Rev., 2016, 116, 10035–10074 CrossRef PubMed;
(c) M. D. Kärkäs, J. A. Porco Jr. and C. R. J. Stephenson, Chem. Rev., 2016, 116, 9683–9747 CrossRef PubMed;
(d) D. Cambie, C. Bottecchia, N. J. W. Straathof, V. Hessel and T. Noel, Chem. Rev., 2016, 116, 10276–10341 CrossRef CAS PubMed;
(e) F. Strieth-Kalthoff, M. J. James, M. Teders, L. Pitzera and F. Glorius, Chem. Soc. Rev., 2018, 47, 7190–7202 RSC;
(f) L. Marzo, S. K. Pagire, O. Reiser and B. König, Angew. Chem., Int. Ed., 2018, 57, 10034–10072 CrossRef CAS PubMed.
- For selected examples, see:
(a) M. Neumann, S. Fuldner, B. Konig and K. Zeitler, Angew. Chem., Int. Ed., 2011, 50, 951–954 CrossRef CAS PubMed;
(b) F. Burg, M. Gicquel, S. Breitenlechner, A. Pöthig and T. Bach, Angew. Chem., Int. Ed., 2018, 57, 2953–2957 CrossRef CAS PubMed;
(c) E. Speckmeier, P. J. W. Fuchs and K. Zeitler, Chem. Sci., 2018, 9, 7096–7103 RSC;
(d) T. Patra, S. Mukherjee, J. Ma, F. Strieth-Kalthoff and F. Glorius, Angew. Chem., Int. Ed., 2019, 58, 10514–10520 CrossRef CAS PubMed;
(e) L. Capaldo, D. Merli, M. Fagnoni and D. Ravelli, ACS Catal., 2019, 9, 3054–3058 CrossRef CAS.
-
(a) P. Kumar, G. Singh, D. Tripathi and S. L. Jain, RSC Adv., 2014, 4, 50331–50337 RSC;
(b) M. Oba, K. Tanak, K. Nishiyama and W. Ando, J. Org. Chem., 2011, 76, 4173–4177 CrossRef CAS PubMed;
(c) K. Y. Desmond Tan, G. F. Teng and W. Y. Fan, Organometallics, 2011, 30, 4136–4143 CrossRef;
(d) X.-B. Li, Z.-J. Li, Y.-J. Gao, Q.-Y. Meng, S. Yu, R. G. Weiss, C.-H. Tung and L.-Z. Wu, Angew. Chem., Int. Ed., 2014, 53, 2085–2089 CrossRef CAS PubMed;
(e) C. Bottecchia, N. Erdmann, P. M. Tijssen, L. G. Milroy, L. Brunsveld, V. Hessel and T. Noël, ChemSusChem, 2016, 9, 1781–1785 CrossRef CAS PubMed;
(f) D. H. Dethe, A. Srivastava, B. D. Dherange and B. V. Kumar, Adv. Synth. Catal., 2018, 360, 3020–3025 CrossRef CAS.
- For selective reviews see:
(a) M. Fagnoni, D. Dondi, D. Ravelli and A. Albini, Chem. Rev., 2007, 107, 2722756 CrossRef PubMed;
(b) D. Ravelli, S. Protti and M. Fagnoni, Chem. Rev., 2016, 116, 9850–9913 CrossRef CAS PubMed;
(c) D. Ravelli, S. Protti and M. Fagnoni, Acc. Chem. Res., 2016, 49, 2232–2242 CrossRef CAS;
(d) N. A. Romero and D. A. Nicewicz, Chem. Rev., 2016, 116, 10075–11116 CrossRef CAS PubMed;
(e) I. K. Sideri, E. Voutyritsa and C. G. Kokotos, Org. Biomol. Chem., 2018, 16, 4596–4614 RSC;
(f) M. A. Theodoropoulou, N. F. Nikitas and C. G. Kokotos, Beilstein J. Org. Chem., 2020, 16, 833–857 CrossRef CAS PubMed.
- For selected example see:
(a) J. Grandjean and D. A. Nicewicz, Angew. Chem., Int. Ed., 2013, 52, 3967–3971 CrossRef CAS PubMed;
(b) I. Ghosh, T. Ghosh, J. I. Bardagi and B. König, Science, 2014, 346, 725–728 CrossRef CAS PubMed;
(c) T. M. Nguyen, N. Manohar and D. A. Nicewicz, Angew. Chem., Int. Ed., 2014, 53, 6198–6201 CrossRef CAS PubMed;
(d) E. Arceo, I. D. Jurberg, A. Álvarez-Fernández and P. Melchiorre, Nat. Chem., 2013, 5, 750–756 CrossRef CAS PubMed;
(e) E. Arceo, E. Montroni and P. Melchiorre, Angew. Chem., Int. Ed., 2014, 53, 12064–12068 CrossRef CAS PubMed;
(f) J. J. Murphy, D. Bastida, S. Paria, M. Fagnoni and P. Melchiorre, Nature, 2016, 532, 218–222 CrossRef CAS PubMed;
(g) L. Pitzer, F. Sandfort, F. Strieth-Kalthoff and F. Glorius, J. Am. Chem. Soc., 2017, 139, 13652–13655 CrossRef CAS PubMed.
- A. Talla, B. Driessen, N. J. W. Straathof, L. G. Milroy, L. Brunsveld, V. Hessel and T. Noel, Adv. Synth. Catal., 2015, 357, 2180–2186 CrossRef CAS.
- For PhCOCOOH-mediated processes, see:
(a) G. N. Papadopoulos, D. Limnios and C. G. Kokotos, Chem. – Eur. J., 2014, 20, 13811–13814 CrossRef CAS PubMed;
(b) G. N. Papadopoulos and C. G. Kokotos, Chem. – Eur. J., 2016, 22, 6964–6967 CrossRef CAS PubMed;
(c) G. N. Papadopoulos and C. G. Kokotos, J. Org. Chem., 2016, 81, 7023–7028 CrossRef CAS;
(d) D. Limnios and C. G. Kokotos, Adv. Synth. Catal., 2017, 359, 323–328 CrossRef CAS;
(e) N. Kaplaneris, A. Bisticha, G. N. Papadopoulos, D. Limnios and C. G. Kokotos, Green Chem., 2017, 19, 4451–4456 RSC;
(f) G. N. Papadopoulos, E. Voutyritsa, N. Kaplaneris and C. G. Kokotos, Chem. – Eur. J., 2018, 24, 1726–1731 CrossRef CAS PubMed;
(g) E. Voutyritsa and C. G. Kokotos, Angew. Chem., Int. Ed., 2020, 59, 1735–1741 CrossRef CAS PubMed;
(h) G. N. Papadopoulos, M. G. Kokotou, N. Spiliopoulou, N. F. Nikitas, E. Voutyritsa, D. I. Tzaras, N. Kaplaneris and C. G. Kokotos, ChemSusChem, 2020, 13, 5934–5944 CrossRef CAS PubMed;
(i) E. Voutyritsa, M. Garreau, M. G. Kokotou, I. Triandafillidi, J. Waser and C. G. Kokotos, Chem. – Eur. J., 2020, 26, 14453–14460 CrossRef CAS PubMed. For other photoinitiators, see:
(j) I. K. Sideri, E. Voutyritsa and C. G. Kokotos, ChemSusChem, 2019, 12, 4194–4201 CrossRef CAS;
(k) N. F. Nikitas, I. Triandafillidi and C. G. Kokotos, Green Chem., 2019, 21, 669–674 RSC;
(l) N. F. Nikitas, D. I. Tzaras, I. Triandafillidi and C. G. Kokotos, Green Chem., 2020, 22, 471–477 RSC;
(m) N. Spiliopoulou, N. F. Nikitas and C. G. Kokotos, Green Chem., 2020, 22, 3539–3545 RSC.
- For full reaction conditions, optimization and mechanistic studies, see ESI.†.
- M. A. Cismesia and T. P. Yoon, Chem. Sci., 2015, 6, 5426–5434 RSC.
Footnote |
† Electronic supplementary information (ESI) available: Experimental data, 1H and 13C NMR, UV-Vis, fluorescence quenching studies and other mechanistic studies. See DOI: 10.1039/d0gc03818k |
|
This journal is © The Royal Society of Chemistry 2021 |
Click here to see how this site uses Cookies. View our privacy policy here.