DOI:
10.1039/D1FO03178C
(Paper)
Food Funct., 2022,
13, 91-101
Neuroprotective and anxiolytic potential of green rooibos (Aspalathus linearis) polyphenolic extract
Received
22nd September 2021
, Accepted 26th November 2021
First published on 26th November 2021
Abstract
South African rooibos (Aspalathus linearis) tea is globally consumed for its health benefits and caffeine free nature, but no information is available on the neuroprotective capacity of (unfermented) green rooibos. Our aim was to investigate the cytoprotective activity of green rooibos in neuronal cells, including probing antioxidant and enzyme inhibitory properties that could explain observed effects in these cells. We also investigated the anxiolytic potential of green rooibos using zebrafish larval models. Green rooibos extract (Green oxithin™) was assessed for its neuroprotective potential in Neuro-2a cells treated with different concentrations of the extract (12.5–25–50–100 μg mL−1) and different concentrations of hydrogen peroxide (250 or 125 μM) as oxidizing agent. Cell viability (MTT) and redox status (intracellular ROS) were also quantified in these cells. Antioxidant properties of the extract were quantified using cell-free systems (DPPH, ORAC and xanthine/xanthine oxidase), and potential neuroprotection evaluated in terms of its potential to inhibit key enzymes of the CNS (monoamine oxidase A (MOA-A), acetylcholinesterase (AChE) and tyrosinase (TYR)). Results demonstrated that green rooibos extract exerted significant cytoprotective properties in Neuro-2a cells, particularly when exposed to lethal 250 μM hydrogen peroxide, increasing cell survival by more than 100%. This may be ascribed (at least partially) to its capacity to limit intracellular ROS accumulation in these cells. Data from cell-free systems confirmed that green rooibos was able to scavenge free radicals (synthetic and physiological) in a dose dependent manner with a similar profile activity to vitamins C and E. Green rooibos also acted as a moderate MAO-A inhibitor, but had no significant effect on AChE or TYR. Finally, zebrafish larvae treated with lower doses of green rooibos demonstrated a significant anxiolytic effect in the light–dark anxiety model. Using the PTZ excitotoxicity model, green rooibos was shown to rescue GABA receptor signalling, which together with its demonstrated inhibition of MAO-A, may account for the anxiolytic outcome. Current data confirms that green rooibos could be considered a “functional brain food” and may be a good option as starting ingredient in the development of new nutraceuticals.
Introduction
Polyphenols are a class of secondary metabolites from plants characterized by aromatic rings with hydroxyl groups.1,2 These compounds are generally classified in the following subgroups according to structural criteria: phenolic acids, flavonoids, anthocyanins, catechins, cumarins, lignans and tannins, among others. Although they work as modulators of plant physiology, they have gained popularity in human health research, as they possess bioactive and pharmacological properties3,4 which may have substantial benefit in the preventative medicine niche, specifically in terms of non-communicable diseases of lifestyle,5 but also in the context of cancer.6 From a nutritional perspective, they behave as non-nutrient bioactive compounds with antioxidant and other modulating properties in a plethora of tissues and organs.7
The consumption of polyphenolic compounds in our diet has been recently associated with a reduction in the development of certain disorders such as cancer or cardiovascular, metabolic and neurodegenerative diseases.7–10 Oxidative stress seems to be involved in the pathogenesis of many chronic diseases and polyphenols may act as antioxidants and as compounds with other relevant bioactivities.11,12
Polyphenols are mainly found in certain groups of plant foods such as fruits and vegetables, virgin olive oil, cocoa, edible flowers, medicinal plants and herbal teas.13
Herbal teas and infusions are very popular worldwide helping to maintain hydration without increasing caloric intake.14,15 In fact, tea (Camellia sinensis) is the most popular beverage all over the world after water.16,17 Other herbal teas that are popularly drunk worldwide (as researched as result of their popularity) are chamomile, rooibos, mate, hibiscus, mint, etc.15
Many herbal teas are normally ingested as part of the food habits but certain users drink these beverages – such as the teas mentioned above – as infusions for medicinal purposes as well.18,19 Whether as a food or as a medicinal plant, herbal teas are interesting sources of bioactive molecules that can participate in many physiological pathways to maintain health or prevent certain diseases, such as cardiometabolic or cardiovascular diseases, as well as other chronic conditions known to result from chronic systemic inflammation.3 In this sense, polyphenols from teas are particularly known to act as antioxidants modulating other physiological processes; they have been demonstrated to be involved in the prevention of chronic and inflammatory disorders such as systemic inflammation or neurodegenerative and metabolic disorders.7,20
Although tea in its different variations is very popular, its use is discouraged in patients with insomnia or anxiety due to its caffeine content.21 Rooibos tea is an exception here, as it is free of caffeine.
In terms of phytochemicals, rooibos contains numerous polyphenols, including dihydrochalcones, flavanones, flavone, flavonols, flavan-3-ols, lignans and hydroxycinnamic derivatives.22–24 The particular chalcone aspalathin (Fig. 1) is one of the main phenolic compounds and is often used for quality control or product standardisation purposes. Rooibos tea is commercially available in forms: the most commonly known, fermented version, and an unfermented (green) version. For fermented rooibos, the needle-shaped plant leaves are bruised and oxidized in open air to allow development of its characteristic organoleptic properties.25 The fermentation process however substantially changes the polyphenol composition of the extract, as previously elucidated.26 In brief, the main differences between unfermented and fermented rooibos is that unfermented rooibos contains higher dihydrochalcone levels – upon fermentation, the dihydrochalcone aspalathin is converted to eriodictyol-glucopyranoside isomers, Unfermented rooibos also contains somewhat higher levels of flavonols.
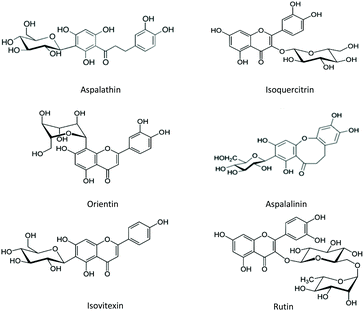 |
| Fig. 1 Examples of flavonoids detected in rooibos (Aspalathus linearis). | |
Traditionally, rooibos tea is considered as an antispasmodic but many other activities such as cardioprotective, antioxidant, anti-inflammatory and antidiabetic properties have been demonstrated.5,25,27–29 Of particular relevance to the current study, despite the fact that anxiolytic and calming effects are arguably the oldest anecdotal properties ascribed to rooibos,27 the potential neurological effects of rooibos have not been elucidated and no relevant data is available for unfermented (green) rooibos.
The main objectives of the current study were therefore to characterise antioxidant and neuroprotection potential of green rooibos using cell free systems, neuronal cell culture and zebrafish larval models.
Results
Antioxidant activity in cell-free systems
The green rooibos extract was tested for its capacity to inhibit DPPH activity or quench peroxyl radicals (ORAC). In addition, its capacity for superoxide radical scavenging was generated using an enzymatic system (xanthine/xanthine oxidase).
Green rooibos was capable of neutralizing both synthetic (DPPH) and physiological (superoxide) radicals (Fig. 2) and compared favourably to the potency of the assay reference standards. ORAC value for the extract was 8.1 μmol TE per mg.
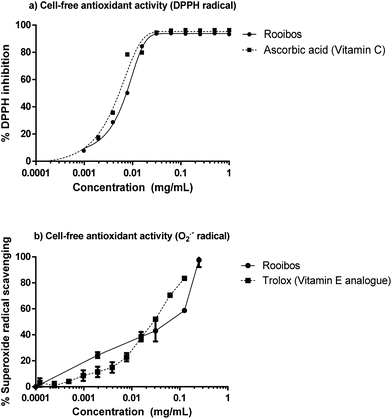 |
| Fig. 2 Antioxidant activity of green rooibos extract in cell-free systems, when compared with the ability of other relevant antioxidants: vitamin C (a) and vitamin E (b) to scavenge DPPH or superoxide radicals respectively. | |
Cytoprotective activity of green rooibos in Neuro-2a cells
Fig. 3 shows the cytotoxicity profile of unfermented (green) rooibos extract after 24 h treatment in neuronal cells. Lower doses did not have any inhibitory effect on mitochondrial reductive capacity, but at the highest concentration tested, mitochondrial viability was dramatically reduced.
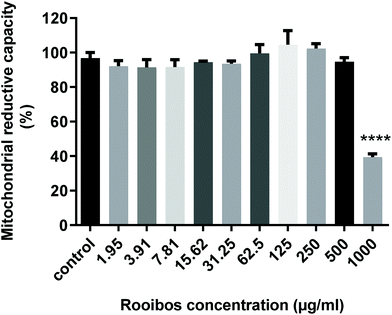 |
| Fig. 3 Cytotoxicity profile of green rooibos extract in the MTT assay expressed as % mitochondrial capacity. **** p < 0.0001 versus control cells (ANOVA and Dunnett's test). | |
The 3-(4,5-dimethylthiazol-2-yl)-2,5-diphenyltetrazolium bromide (MTT) assay data suggests a dose-dependent but non-significant initial increasing mitochondrial activity, which peaks at a dose of 125 μg ml−1, after which decreases in mitochondrial capacity becomes evident, although only significant at excessive dose. Since the dose range yielding somewhat increased mitochondrial capacity corresponded with the doses shown to have significant effect in the antioxidant assays, four different concentrations of green rooibos extract ranging from 12.5–100 μg ml−1 were evaluated for their cytoprotective potential against oxidative stress induced by two different concentrations of hydrogen peroxide (250 and 125 μM).
Fig. 4 shows that increasing concentrations of unfermented rooibos extract exert cytoprotection against an oxidative insult induced by hydrogen peroxide using the MTT assay of mitochondrial activity. The statistical significance can only be observed when cells were pre-treated with 50 and 100 μg mL−1 for 24 h and hydrogen peroxide was applied at a concentration of 250 μM, generating a lethal oxidative stress (Fig. 4a). However, when hydrogen peroxide was applied at sub-lethal doses (125 μM), significant protective effects were not detected in the MTT assay (Fig. 4b).
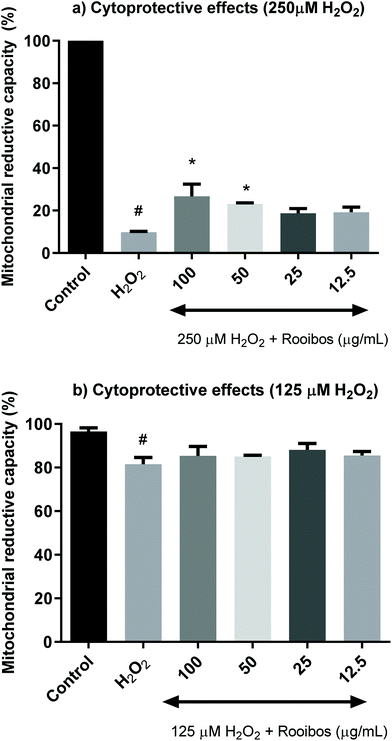 |
| Fig. 4 Cytoprotective activity of green rooibos extract in Neuro-2a cells exposed to a lethal oxidative insult with 250 μM H2O2 (a) or exposed to a sub-lethal treatment with 125 μM H2O2 (b) for 24 h; rooibos significantly increased mitochondrial reductive capacity from 9.7% in cells treated with 250 μM H2O2 up to 26.7% or 23.1% with 100 and 50 μg mL−1 of extract pre-treatments for 24 h (#p < 0.0001 versus control, * p < 0.05 versus hydrogen peroxide, ANOVA and Dunnett's multicomparison test). When generating oxidative stress at 125 μM, mitochondrial reductive capacity was not modified significantly (from 81.5% in cells exposed to hydrogen peroxide to 85.3 and 85.1% in cells pre-treated with 100 and 50 μg mL−1 of rooibos extract for 24 h). | |
The protective effects of green rooibos extract were also tested in Neuro-2a cells in terms of intracellular ROS production (Fig. 5). The cells were co-treated with increasing concentrations of extract (12.5–25–50–100 μg mL−1) and 250 or 125 μM H2O2 for a period of 90 min. Results demonstrated that green rooibos significantly decreased ROS production in the cells, thus effectively modulating intracellular oxidative stress profile.
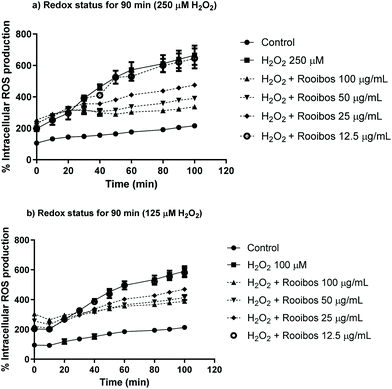 |
| Fig. 5 Redox status modulation by green rooibos extract expressed as % of intracellular ROS production in Neuro-2a cells exposed to a lethal oxidative insult with 250 μM H2O2 (a) or exposed to a sub-lethal treatment with 125 μM H2O2 (b) for 90 min. Asterisks showing significance are not included in the figure in order to avoid misinterpretations and clarify the figure; at 70–80–90 min three extract concentrations (100–50–25 μg mL−1) significantly prevented intracelullar ROS production compared to cells treated alone with 250 or 125 μM H2O2 (**** p < 0.0001, Two-way ANOVA and Tukey's multiple comparisons test). | |
In order to probe pathways as alternative to direct anti-oxidant activity, which may also affect neuronal cell survival, the effect of green rooibos extract on selected neuroenzymes was assessed. In this context, green rooibos extract exhibited dose-dependent effect as MAO-A inhibitor, but this activity was not superior to the reference selective inhibitor clorgyline (Fig. 6). No inhibition of two other enzymes involved in neuroprotection, AChE and TYR, was detected (data not shown).
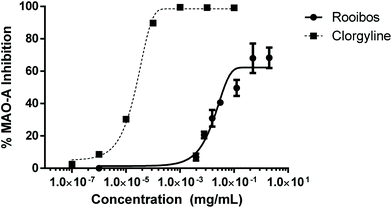 |
| Fig. 6 Enzyme inhibition of neuroenzyme monoamine oxidase -A (MAO-A) by green rooibos extract, in comparison to to the reference inhibitor clorgyline. | |
Neuroprotective effect of rooibos in zebrafish larval models
Analysis of total distance moved by zebrafish larvae in the light–dark anxiety assay demonstrated an ANOVA main effect of rooibos dose on total distance moved under both the light and dark conditions (P < 0.0001, Fig. 7), with especially lower concentrations of rooibos tested, decreasing activity to levels similar as those seen in diazepam-treated larvae after 1 h of exposure, while higher doses only produced significant effects after 24 h of exposure.
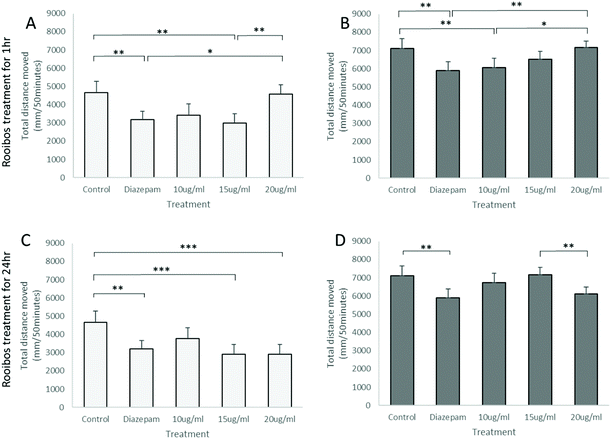 |
| Fig. 7 Activity of zebrafish larvae in the light–dark anxiety assay. Cumulative distances moved during the 5 light (A) and 5 dark (B) cycles after 1 h or 24 h (C and D) exposure to green rooibos exposure are presented, using 4uM diazepam treatment immediately before tracking as positive treatment control. Statistics: Factorial ANOVA showed ANOVA main effect of treatment dose (P < 0.0001) for both treatment protocols and both lighting conditions. Bonferroni post hoc: *, P < 0.05; **, P < 0.01; ***, P < 0.001, n = 12 per group. | |
These activity profiles suggestive or an anxiolytic effect of low dose green rooibos corresponded lower total body ROS (H2O2) levels after 1 h of exposure to rooibos (Fig. 8), but not after 24 h, where exposure to higher levels of green rooibos was not associated with a benefit in terms of ROS levels.
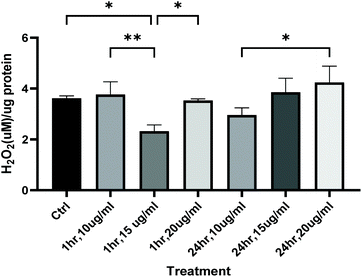 |
| Fig. 8 Whole body reactive oxygen species (H2O2) levels measured in zebrafish larvae at 116hpf, after either 1 h or 24 h exposure (by immersion) to green rooibos extract doses 10, 15 and 20 ug ml−1 in E3. Statistics: One-way ANOVA indicated main effect of treatment dose (P < 0.001), Bonferroni post hoc: *, P < 0.05, **, p < 0.01. | |
As ROS levels thus could not fully explain the anxiolytic response observed, the potential mechanism of rooibos was also probed in terms of its effect on GABA receptor signalling. In the PTZ assay, PTZ was shown to result in significantly increased activity, which was prevented by co-treatment with diazepam (Fig. 9), validating the model. None of the green rooibos doses assessed showed significant therapeutic effect after 1 h of exposure. However, after 24 h pre-treatment, both lower doses of green rooibos was able to prevent the PTZ-associated hyperactivity, but not the high dose, which was associated with an activity profile similar to that of PTZ.
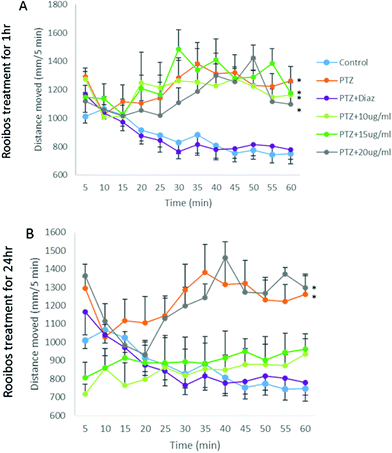 |
| Fig. 9 Activity tracks after (A) 1 h and (B) 24 h exposure to green rooibos extract, illustrating PTZ-induced hyperactivity, that are prevented by co-administration of 4uM diazepam, as well as lower doses of green rooibos after 24 h treatment. Statistics: repeated measures ANOVA showed main effect of treatment dose (P < 0.05) after 24 h treatment. Bonferroni post hoc: n = 12; *, P < 0.05 – total distance moved similar for groups with * and significantly higher than all other groups. | |
Discussion
Current data significantly adds to the literature on rooibos, by elucidating its potential for neuroprotection via two different pathways; firstly, we demonstrate free radical scavenging effect in neuronal cells and secondly, the anxiolytic potential of green rooibos, via its inhibition of the neuroenzyme monoamine oxidase A, as well as upregulation of signalling via GABA receptor.
In terms of antioxidant properties, the green rooibos extract assessed presented with a similar antioxidant activity to vitamin C or E in the tested cell-free models. These results are in line with previous reports demonstrating antioxidant properties of green rooibos using similar (DPPH and/or superoxide scavenging) and different (earlier) methodologies, such as the ABTS˙+ radical cation decolorization assay, the β-carotene bleaching assay and the Rancimat assessment for oxidation stability.30–34 In addition, anti-inflammatory, antidiabetic and cardioprotective properties has been reported for green rooibos25,27,35–39 – interestingly, the aetiology of chronic inflammatory disease, diabetes and cardiovascular disease all have increased oxidative stress as major role player,40 which suggests that these beneficial effects reported may at least in part, be as a result of the antioxidant capacity of green rooibos.
The potent antioxidant capacity demonstrated in the DPPH and ROS activity inhibition assays were also evident in the neuronal cell culture model, where doses of up to 500 μg ml−1 of green rooibos did not significantly affect mitochondrial reductive capacity – an indirect measure of mitochondrial and cellular viability. Although the highest dose employed exhibited decreased mitochondrial activity, this result should be interpreted in context of the dose used. To place this result into context of rooibos consumption, it is important to consider that for the current model and extract, a dose of 25 μg ml−1 extract roughly equates to the antioxidants contained in one cup of rooibos tea. This calculation is however made under the assumption of 100% absorption – as is the norm for this type of calculation. However, it is well known that many of the flavonoids contained in rooibos are poorly absorbed from the gut.41,42 Thus, the highest dose employed here – which was calculated to be equivalent to 40 cups of tea – may be significantly stronger than this calculation suggests. This fully transparent data indicates that green rooibos consumption in physiologically feasible amounts carries no risk of cytotoxicity.
In terms of cytoprotection from a lethal dose of H2O2, green rooibos showed a remarkable dose-dependent capacity to rescue more than 25% of cells from the apoptotic cell death known to be induced by H2O2, when compared to a survival rate of less than 10% in untreated H2O2-exposed cells. Interestingly, in cells exposed to a weaker H2O2 challenge, this protective effect was not evident. Two factors may account for this result. Firstly, in vitro exposure of cortical neurons to H2O2 illustrated increased intracellular calcium after exposure to high, but not low doses of H2O2.43 Secondly, neuro-2a cells have been reported to have relatively high intracellular glutathione levels (compared to PC12 cells;44) and low expression of voltage-gated sodium channels (20-fold lower than cerebellar granule neurons;45) – both characteristics reported to afford neuro-2a cells relatively greater resistance to H2O2-induced oxidative stress and neurotoxicity. Thus, taken together, this result suggests that the milder dose of H2O2 was either insufficient to challenge the cells beyond their endogenous antioxidant capacity, or that the relatively smaller decrease in mitochondrial activity may have resulted via a pathway unrelated to oxidative stress levels, or both.
Furthermore, although rooibos is anecdotally claimed to have a calming effect, in vivo data in support of this claim is currently relatively sparse. We found only one very recent study reporting behaviour suggestive of decreased anxiety in a rodent model, after supply of rooibos as only fluid in housing cages for 3 months.46 In the current study, anxiety behaviour in zebrafish in the light–dark assay was significantly lower after exposure to green rooibos, suggesting dose-specific anxiolytic potential of rooibos and confirming anecdotal claims. Of interest, while lower doses seemed to elicit decreases in activity similar to that of diazepam, this effect was lost after 24 h exposure, when only the highest dose seemed effective. This may suggest that in the 24 h exposure protocol, the psychoactives in rooibos had broken down, so that the high dose treatment had reached the lower “effective” dose, while the lower doses had broken down to ineffective doses. This warrants further analysis of different fractions of rooibos, in order to pinpoint specific actives, so that stability testing may be performed to optimise supplementation strategy. To our knowledge, the current study is the first illustrating potential anxiolytic effects for green rooibos, and at relavely acute time points, which further supports a direct psychoactive effect.
In terms of mechanisms involved, the green rooibos extract employed in the current study was rich in polyphenols (41%); thus its significant dose-dependent inhibition of intracellular ROS production is not surprising. Significantly, when the green rooibos extract is compared with other beverages such as juices obtained from blueberry, cranberry or cherry, its ORAC value was much higher (approx. 8.1 μmol TE per mg for green rooibos extract vs. 0.85, 0.64, 0.60 μmol TE per mg for blueberry, cranberry and cherry respectively).47
Although the cytoprotective properties of green rooibos likely result from the specific mechanisms – such as its large free radical scavenging capacity – that we report here, the decreased ROS levels observed in zebrafish could not fully explain the anxiolytic effect of rooibos in vivo – clearly, other mechanisms also came into play.
Indeed, current data suggest that additional mechanisms may contribute to the beneficial effects elucidated. For example, MAO-A inhibitors have previously been suggested as neuroprotective agents with important applications in neurology and psychiatry.48–53 The inhibition of the MAO-A enzyme by the green rooibos extract could be due to the presence of flavonoids such as quercetin and/or quercitin derivatives, for which MAO-A-inhibiting properties have been reported.52,54 In addition, in zebrafish, the stimulatory response of the GABAA receptor antagonist PTZ was prevented in the presence of green rooibos extract. Of interest, lower doses of rooibos were again more beneficial than higher doses, but required a 24 h exposure time before effects became evident. This data, suggesting a calming effect of green rooibos via potentiated GABAA receptor signalling, warrants further investigation.
Current results are in line with reports identifying fermented rooibos as a neuroprotective agent in animal models, where it was shown to decrease lipid peroxides and confering protection against ischemic brain injury in rats.55,56 No comparative data on neuroprotective effects of fermented vs. unfermented rooibos is available. In our opinion, such a comparison between differences in composition and differences in physiological effect, may contribute to a better understanding and highlight from a mechanistic point of view, the in vivo observed effects in relation with the central nervous system.
Experimental procedures
Reagents and chemicals
Foetal bovine serum (FBS), phosphate-buffered saline (PBS) and gentamicin were purchased from Gibco (Invitrogen, Paisley, UK). 3-(4,5-Dimethyl-2-thiazolyl)-2,5-diphenyltetrazolium bromide (MTT), dimethyl sulphoxide (DMSO), Dulbecco's modified Eagle's medium (DMEM), hydrogen peroxide solution (30% w/w), 6-hydroxy-2,5,7,8-tetramethylchromane-2-carboxylic acid (TROLOX), 2,7-dichloro-dihydrofluorescein diacetate (DCFH-DA), monoamine oxidase A (human recombinant), acetylcholinesterase (from electric eel), tyrosinase (from mushroom) and lipoxidase (from soybean) were purchased from Sigma-Aldrich (St Louis, MO, USA). Chemical reagents for chemical assays were of analytical grade.
Green rooibos extract
A registered, commercially available, green rooibos extract (Green Oxithin ™) was kindly donated by Mr Roy van Brummelen (Van Brummelen Consultants CC, Pretoria, South Africa – local trademark owner). Briefly, a natural product extraction specialist company (Brenn-O-Kem (Pty) Ltd, Wolseley, South Africa) extracted unfermented Aspalathus linearis leaves in ethanol using a proprietary method. All solvents were subsequently removed using appropriate industrial methods and the product tested to confirm compliance to industry standards. The moisture content of the extract was 0.1% and total polyphenol content was 41.1% according to the Folin method.57
Antioxidant characterisation of rooibos in cell-free systems
DPPH antiradical assay.
In 96-well microplates, each well contained 150 μL of extract at different concentrations and 150 μL of DPPH (0.04 mg mL−1 methanol solution).58 Antioxidant activity was determined by measuring absorbance (Abs) at 515 nm after 30 min of dark incubation. Blank and control wells were also considered. The highest concentration of extract tested was 1 mg mL−1. Vitamin C (ascorbic acid) was used as positive standard. Background interferences from solvents and samples were deducted from the activities prior to calculating radical scavenging capacity (RSC) as follows: RSC (%) = [(Abscontrol − Abssample)/Abscontrol] × 100.
ORAC assay.
ORAC assay was carried out to measure the capacity of extracts to scavenge peroxyl radicals (Davalos et al., 2004).65 Samples and trolox were dissolved in PBS and methanol (50
:
50, v
:
v). Samples were incubated with fluorescein (0.07 μM) in 96-well plates for 10 min at 37 °C. Afterwards, 0.012 M AAPH (2,2′-azobis(2-methylpropionamidine) dihydrochloride) was added and fluorescence was measured for 98 min at 485 nm of excitation and 520 nm of emission. Results were expressed as μmol trolox equivalents (TE) per mg sample.
Superoxide radicals generated by xanthine/xanthine oxidase.
The effects of rooibos extract as superoxide radical scavenger was evaluated by measuring the formation of the NBT (nitrotetrazolium blue chloride)-radical superoxide complex.59 The reaction mixture is known to have poor stability and thus was prepared freshly every day. This mixture was composed of 90 μM xanthine, 16 mM Na2CO3, and 22.8 μM NBT in phosphate buffer (pH 7.0). 240 μL of the reaction mixture in each well with 30 μL of extract solution and xanthine oxidase (XO) was incubated in the dark for 2 min at 37 °C and absorbance read at 560 nm. Blank and control wells were also considered, and background interferences from solvents and samples were also deducted from the activities prior to calculating the RSC (%).
Cytoprotective and antioxidant activity of rooibos in Neuro-2a cells
Cell culture.
Rat neuroblastoma cells (Neuro-2a) were acquired from the ATCC (ATCC® CCL-131™) and were cultured in DMEM supplemented with 10% fetal bovine serum (FBS) and 1% gentamicin. Growth media was replaced every two or three days and cultures split once they reached approximately 80% confluence. Cells were seeded in flasks for stock maintenance and in 96-well plates for experiments. All cultures were maintained under standard cell culture conditions of 5% CO2 atmosphere at 37 °C. All cell culture experiments were conducted in triplicate and repeated three times.
Cellular protection after rooibos treatment.
Stock solution of Aspalathus linearis (Green OxithinTM) was prepared in dimethyl sulfoxide (DMSO) and vortexed for 5 min. This mixture was then diluted with pre-warmed media and a serial dilution was done to give final working concentrations of treatments (final concentration of DMSO was 0.1% or lower). N2a cells were treated with a range of concentrations from 0–1000 μg mL−1 and 0–800 μg mL−1 respectively, for 24h to determine tolerance of cells for the extract by MTT assay.
The MTT assay was used as a predictor of cell survival and mitochondrial activity.60 After treatments, cells were incubated with MTT solution (2 mg mL−1) for 3 h at 37 °C. Successively, the medium was replaced with DMSO in each well to dissolve formazan crystals. Finally, absorbance was measured at 550 nm. All experiments were performed in triplicate and results were expressed as a percentage of control (100%).
Doses of rooibos showing maximal cell viability in the MTT assay (12.5–100 μg mL−1) was used for subsequent assays in N2a cells – cells were pre-treated with 4 different concentrations of extract (12.5–25–50–100 μg mL−1) for 24 h prior to hydrogen peroxide exposure (125 or 250 μM) for 24 h. At the end of this protocol, cell survival was once again assessed using MTT.
Production of intracellular reactive oxygen species (ROS) in N2a cells.
DCFH-DA (2,7-dichloro-dihydrofluorescein diacetate) is a fluorescent reagent andwas used to detect the production of ROS at intracellular level. Briefly, cells were incubated in PBS-glucose +50 μl of DCFH-DA (0.01 M) at 37 °C and preserved from the light for 30 min. After this time, cells were twice washed with PBS-glucose and then different concentrations of rooibos extract and H2O2 (125 or 250 μM) were added. Generation of ROS was measured for 1 hour and 30 min (0′ 10′ 30′ 60′ 90′) in a microplate fluorescence reader (at λexcitation of 480 nm and λemission of 580 nm).
Enzyme inhibitory properties
Monoamine oxidase A (MAO-A) inhibition.
The inhibition of human recombinant MAO-A was evaluated using a previously described protocol.52 Each well contained 50 μl of rooibos extract in DMSO, 50 μl chromogenic solution (0.8 mM vanillic acid, 417 mM 4-aminoantipyrine and 4 U ml−1 horseradish peroxidase in potassium phosphate buffer pH 7.6.), 100 μl of 3 mM tyramine and 50 μl of 8 U ml−1 MAO-A. Control wells contained 50 μl of solvent. The absorbance was read at 490 nm every 5 min for 30 min. Clorgyline was used as reference substance.
Acetylcholinesterase (AChE) inhibition.
A modification of the Ellman's method was run using a 96-microplate reader.61 Each well contained 25 μl of 15 mM ATCI in Millipore water, 125 μl of 3 mM DTNB in buffer C (50 mM Tris-HCl, pH = 8, 0.1 M NaCl, 0.02 M MgCl2·6H2O), 50 μl buffer B (50 mM Tris-HCl, pH 8, 0.1% bovine serum) and 25 μl of rooibos extract in DMSO. Finally, 25 μl of the enzyme (0.22 U L−1) was added to start the reaction. Absorbance was read 13 times, every 13 s at 405 nm. Galantamine was used as reference substance.
Tyrosinase (TYR) inhibition.
This assay was carried out in 96-well microplates as previously described.62 10 μl of different concentrations of rooibos extract in DMSO, 40 μl of 5 mM L-DOPA, 80 μl phosphate buffer (pH 6.8) and 40 μl of tyrosinase (200 U mL−1) were mixed in each well. Controls contained 50 μl of solvent instead of extract. α-Kojic acid was used as reference substance.
Zebrafish larval behavioural changes effected by rooibos
Ethical considerations.
Zebrafish experiments were carried out on wild-type zebrafish larvae less than 5 days after fertilisation (<5 dpf). All protocols were ethically cleared by the Stellenbosch University Animal Research Ethics Committee (ref# ACU-2019-11820). Zebrafish eggs were obtained from the Zebrafish Research Unit in the division Clinical Pharmacology, Faculty of Medicine and Health Sciences, Stellenbosch University, within 1 hour of spawning using standardised methods. Eggs and larvae were maintained in E3 embryo medium at 28 °C and refreshed daily for the duration of the protocol.
Anxiety assay.
In order to evaluate the anxiolytic potential of green rooibos in vivo, the light–dark assay63 was employed. Zebrafish larvae were exposed for either 1 h or 24 hours to rooibos (10–25 μg ml−1) in 96-well plates. The activity of larvae was then tracked for a baseline period of 30 minutes, followed by repeated light–dark cycles (10 minutes bright light; 10 minutes dark; Fig. 10), in order to assess anxiety behaviour. Acute administration of 4 μM diazepam was used as internal validation of the assay.
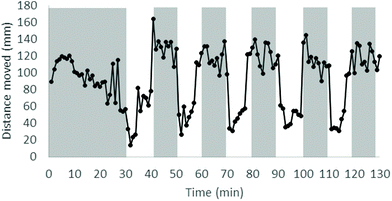 |
| Fig. 10 Protocol employed for the light–dark assay. Larvae were tracked for a baseline of 30 minutes to allow them to settle, after which they were exposed to 5 light (white panels) – dark (grey panels) cycles of 10 minutes each. The track illustrates activity of control zebrafish larvae at <5 dpf. | |
PTZ assay.
In order to probe potential mechanisms, the pentylenetetrazol (PTZ) assay64 was employed to assess the potential of rooibos to prevent excitotoxicity via modulation of GABA receptor activity. Briefly, larvae were exposed to green rooibos extract at a variety of doses (10–25 μg ml−1) for either 1 hour or 24 hours. (Controls, as well as PTZ and diazepam treatment controls, were maintained in E3 for the same amount of time, i.e. not exposed to rooibos extract.) Larvae were then pipetted into 96-well plates at one larva per well, in 150 μl E3. 100 μl of E3 (control), or 50 μl E3 plus 50 μl E3 containing PTZ (seizure-inducing challenge; final concentration 10 mM) or 50 μl E3 containing PTZ plus 50 μl E3 containing diazepam (DIAZ, positive treatment control; final concentration of 4 μM) was added to respective wells. Activity tracking was performed immediately after.
Activity tracking for all larvae was conducted at 116hpf, using Daniovision larval activity tracking equipment and Ethovision software (Noldus, Wageningen, Netherlands). A smoothing profile was applied, with minimum distance moved set at 0.2 mm. Activity data were sorted into 5-minute bins for visualisation, but total distance moved was used for group comparisons.
Hydrogen peroxide (ROS) assay.
Hydrogen peroxide (H2O2) concentrations in whole zebrafish larvae were determined using a commercial Hydrogen Peroxide colorimetric assay kit (Elabscience, E-BC-K102-S, USA) according to manufacturer's instructions with minor modifications. Briefly, after exposure of zebrafish larvae for either 1 h or 24 hours to rooibos (10–25 μg ml−1), 30 zebrafish larvae (<5 dpf) per sample were pooled and homogenized in 500 μl ice cold PBS (pH 7.4). Homogenates were centrifuged at 10000g at 4 °C for 10 minutes and supernatants were collected for the assay. 100 μl of the buffer solution 1 was added to 100 μl of reagent 2 along with 10 μl of H2O2 standard or 20 μl of sample in a 96 well plate and read at 405 nm on a microplate reader (Victor Nivo Multimode Plate Reader, PerkinElmer). The assay was performed in triplicate, on samples from three different experiments.
Statistical analysis
All cell culture experiments were performed in triplicate and repeated on three different days. Zebrafish assays were conducted using n = 12 per treatment group. Data are expressed as mean ± SEM (standard error of the mean). GraphPad Prism v.6 was used for data analyses, figures, non-linear regression and statistical analysis. Cell experiments were analysed using ANOVA followed by Dunnett's or Tukeýs multicomparison test. Zebrafish activity data was analysed using Statistica v.14, using repeated measures ANOVA and Bonferroni post hoc tests.
Conclusions
Unfermented (green) rooibos was demonstrated to be a rich source of phenolic compounds with antioxidant, neuroprotective and anxiolytic potential. On a practical note, our data highlights the importance of combining in vitro and in vivo models in the study of especially neuroactive components, to allow for a more integrated data interpretation and thus more comprehensive elucidation of potential mechanisms of action. Going forward, current data suggest that – in addition to its already established role in prevention of cardiovascular disease – unfermented rooibos extracts could potentially also be exploited as a functional brain food. Its use as an ingredient in pharmaceutical or nutraceutical products may have impact in the prevention of neurological disorders or cognitive decline of ageing.
Author contributions
VL and CS conceptualised and designed the study, as well as jointly providing funding. GC performed experiments in cell free systems and neuronal cells under supervision of VL. KPR and YP performed experiments in zebrafish under supervision of CS. All authors participated in data reduction and interpretation. CS wrote the first draft of the paper. All authors edited and approved the final manuscript.
Conflicts of interest
There are no conflicts to declare.
Acknowledgements
Universidad San Jorge and the South African National Research Foundation is acknowledged for financial support.
References
- A. F. Almeida, C. N. dos Santos and M. R. Ventura, Polyphenols, their metabolites and derivatives as drug leads, Curr. Pharm. Des., 2018, 24, 2188–2207 CrossRef CAS PubMed.
- A. B. Santhakumar, M. Battino and J. M. Alvarez-Suarez, Dietary polyphenols: structures, bioavailability and protective effects against atherosclerosis, Food Chem. Toxicol., 2018, 113, 49–65 CrossRef CAS.
- C. G. Fraga, K. D. Croft, D. O. Kennedy and F. A. Tomás-Barberán, The effects of polyphenols and other bioactives on human health, Food Funct., 2019, 10, 514–528 RSC.
- N. C. Veitch and R. J. Grayer, Flavonoids and their glycosides, including anthocyanins, Nat. Prod. Rep., 2011, 28, 1626–1695 RSC.
- L. Schloms, C. Smith, K. H. Storbeck, J. L. Marnewick, P. Swart and A. C. Swart, Rooibos influences glucocorticoid levels and steroid ratios in vivo and in vitro: a natural approach in the management of stress and metabolic disorders?, Mol. Nutr. Food Res., 2014, 58, 537–549 CrossRef CAS.
- O. R. Oyenihi, A. Krygsman, N. Verhoog, D. de Beer, M. J. Saayman, T. M. Mouton and A. Louw, Chemoprevention of LA7-Induced Mammary Tumor Growth by SM6Met, a Well-Characterized Cyclopia Extract, Front. Pharmacol., 2018, 20(9), 650 CrossRef PubMed.
- W. Koch, Dietary Polyphenols—Important Non-Nutrients in the Prevention of Chronic Noncommunicable Diseases. A Systematic Review, Nutrients, 2019, 11(5), 1039 CrossRef CAS.
- D. Gentile, M. Fornai, C. Pellegrini, R. Colucci, C. Blandizzi and L. Antonioli, Dietary flavonoids as a potential intervention to improve redox balance in obesity and related co-morbidities: a review, Nutr. Res. Rev., 2018, 31, 239–247 CrossRef CAS.
- N. Khan and H. Mukhtar, Tea Polyphenols in Promotion of Human Health, Nutrients, 2019, 11(1), 39 CrossRef CAS.
- K. A. Woodward, R. Draijer, D. H. J. Thijssen and D. A. Low, Polyphenols and Microvascular Function in Humans: A Systematic Review, Curr. Pharm. Des., 2018, 24, 203–226 CrossRef CAS PubMed.
- F. Ito, Y. Sono and T. Ito, Measurement and Clinical Significance of Lipid Peroxidation as a Biomarker of Oxidative Stress: Oxidative Stress in Diabetes, Atherosclerosis, and Chronic Inflammation, Antioxidants, 2019, 28(3), 72 CrossRef.
- E. Zaplatic, M. Bule, S. Z. A. Shah, M. S. Uddin and K. Niaz, Molecular mechanisms underlying protective role of quercetin in attenuating Alzheimer's disease, Life Sci., 2019, 224, 109–119 CrossRef CAS PubMed.
- O. C. Adebooye, A. M. Alashi and R. E. Aluko, A brief review on emerging trends in global polyphenol research, J. Food Biochem., 2018, 42, e12519 CrossRef.
- A. Chandrasekara and F. Shahidi, Bioactive compounds and their role in disease risk reduction, J. Tradit. Complement. Med., 2018, 8, 451–458 CrossRef.
- A. T. Valduga, I. L. Gonçalves, E. Magri and J. R. Delalibera Finzer, Chemistry, pharmacology and new trends in traditional functional and medicinal beverages, Food Res. Int., 2019, 120, 478–503 CrossRef CAS.
- M. Naveed, J. BiBi, A. A. Kamboh, I. Suheryani, I. Kakar, S. A. Fazlani, X. FangFang, S. A. kalhoro, L. Yunjuan, M. U. Kakar, M. E. Abd El-Hack, A. E. Noreldin, S. Zhixiang, C. LiXia and Z. XiaoHui, Pharmacological values and therapeutic properties of black tea (Camellia sinensis): A comprehensive overview, Biomed. Pharmacother., 2018, 100, 521–531 CrossRef CAS.
- N. Sanlier, İ. Atik and A. Atik, Dietary risk assessment of fluoride, lead, chromium, and cadmium through consumption of Tieguanyin tea and white tea, Trends Food Sci. Technol., 2018, 82, 82–88 CrossRef CAS.
- D. L. McKay and J. B. Blumberg, A review of the bioactivity and potential health benefits of peppermint tea (Mentha piperita L.), Phyther. Res., 2006, 20(8), 619–633 CrossRef CAS PubMed.
- D. L. McKay and J. B. Blumberg, A Review of the bioactivity and potential health benefits of chamomile tea (Matricaria recutita L.), Phyther. Res., 2006, 20(7), 519–530 CrossRef CAS PubMed.
- R. Bahramsoltani, F. Ebrahimi, M. H. Farzaei, A. Baratpourmoghaddam, P. Ahmadi, P. Rostamiasrabadi, A. H. R. Amirabadi and R. Rahimi, Dietary polyphenols for atherosclerosis: A comprehensive review and future perspectives, Crit. Rev. Food Sci. Nutr., 2019, 59, 114–132 CrossRef CAS PubMed.
- S. K. Bhatti, J. H. O'Keefe and C. J. Lavie, Coffee and tea: perks for health and longevity?, Curr. Opin. Clin. Nutr. Metab. Care, 2013, 16, 688–697 CrossRef CAS PubMed.
- F. Malongane, L. J. McGaw, H. Nyoni and F. N. Mudau, Metabolic profiling of four South African herbal teas using high resolution liquid chromatography-mass spectrometry and nuclear magnetic resonance, Food Chem., 2018, 257, 90–100 CrossRef CAS PubMed.
- M. A. Stander, B. E. Van Wyk, M. J. C. Taylor and H. S. Long, Analysis of Phenolic Compounds in Rooibos Tea (Aspalathus linearis) with a Comparison of Flavonoid-Based Compounds in Natural Populations of Plants from Different Regions, J. Agric. Food Chem., 2017, 65, 10270–10281 CrossRef CAS PubMed.
- N. A. Walters, A. de Villiers, E. Joubert and D. de Beer, Phenolic profiling of rooibos using off-line comprehensive normal phase countercurrent chromatography×reversed phase liquid chromatography, J. Chromatogr. A, 2017, 1490, 102–114 CrossRef CAS.
- C. Smith and A. Swart, Aspalathus linearis (Rooibos) – a functional food targeting cardiovascular disease, Food Funct., 2018, 9, 5041–5058 RSC.
- N. A. Walters, A. de Villiers, E. Joubert and D. de Beer, Improved HPLC method for rooibos phenolics targeting changes due to fermentation, J. Food Compos. Anal., 2017, 55, 20–29 CrossRef CAS.
- C. Smith and A. C. Swart, Rooibos (Aspalathus linearis) facilitates an anti-inflammatory state, modulating IL-6 and IL-10 while not inhibiting the acute glucocorticoid response to a mild novel stressor in vivo, J. Funct. Foods, 2016, 27, 42–54 CrossRef CAS.
- R. Johnson, D. De Beer, P. V. Dludla, D. Ferreira, C. J. F. Muller and E. Joubert, Aspalathin from Rooibos (Aspalathus linearis): A Bioactive C-glucosyl Dihydrochalcone with Potential to Target the Metabolic Syndrome, Planta Med., 2018, 84, 568–583 CrossRef CAS PubMed.
- M. Sasaki, N. Nishida and M. Shimada, A Beneficial Role of Rooibos in Diabetes Mellitus: A Systematic Review and Meta-Analysis, Molecules, 2018, 23(4), 839 CrossRef PubMed.
- L. Bramati, F. Aquilano and P. Pietta, Unfermented rooibos tea: quantitative characterization of flavonoids by HPLC-UV and determination of the total antioxidant activity, J. Agric. Food Chem., 2003, 51, 7472–7474 CrossRef CAS PubMed.
- E. Joubert, P. Winterton, T. J. Britz and D. Ferreira, : Superoxide anion radical and α,α-diphenyl-β-picrylhydrazyl radical scavenging capacity of rooibos (Aspalathus linearis) aqueous extracts, crude phenolic fractions, tannin and flavonoids, Food Res. Int., 2004, 37, 133–138 CrossRef CAS.
- E. Joubert, P. Winterton, T. J. Britz and W. C. A. Gelderblom, Antioxidant and pro-oxidant activities of aqueous extracts and crude polyphenolic fractions of rooibos (Aspalathus linearis), J. Agric. Food Chem., 2005, 53, 10260–10267 CrossRef CAS PubMed.
- H. Schulz, E. Joubert and W. Schütze, : Quantification of quality parameters for reliable evaluation of green rooibos (Aspalathus linearis), Eur. Food Res. Technol., 2003, 216, 539–543 CrossRef CAS.
- A. Von Gadow, E. Joubert and C. F. Hansmann, Comparison of the antioxidant activity of rooibos tea (Aspalathus linearis) with green, Production and quality aspects of rooibos tea and related products 143 oolong and black tea, J. Agric. Food Chem., 1997, 45, 632–638 CrossRef CAS.
- A. C. Hattingh, M. van de Venter and T. C. Koekemoer, The effects of rooibos (Aspalathus linearis) on 3T3-L1 preadipocytes after the induction of mitochondrial dysfunction, J. Funct. Foods, 2019, 55, 184–192 CrossRef CAS.
- R. Kamakura, M. J. Son, D. de Beer, E. Joubert, Y. Miura and K. Yagasaki, Antidiabetic effect of green rooibos (Aspalathus linearis) extract in cultured cells and type 2 diabetic model KK-A(y) mice, Cytotechnology, 2015, 67, 699–710 CrossRef CAS.
- S. E. Mazibuko, C. J. F. Muller, E. Joubert, D. De Beer, R. Johnson, A. R. Opoku and J. Louw, Amelioration of palmitate-induced insulin resistance in C2C12 muscle cells by rooibos (Aspalathus linearis), Phytomedicine, 2013, 20, 813–819 CrossRef CAS PubMed.
- N. Mikami, J. Tsujimura, A. Sato, A. Narasada, M. Shigeta, M. Kato, S. Hata and E. Hitomi, Green Rooibos Extract from Aspalathus linearis, and its Component, Aspalathin, Suppress Elevation of Blood Glucose Levels in Mice and Inhibit α-amylase and α-glucosidase Activities in vitro, Food Sci. Technol. Res., 2015, 21, 231–240 CrossRef CAS.
- W. G. Pantsi, J. L. Marnewick, A. J. Esterhuyse, F. Rautenbach and J. Van Rooyen, Rooibos (Aspalathus linearis) offers cardiac protection against ischaemia/reperfusion in the isolated perfused rat heart, Phytomedicine, 2011, 18, 1220–1228 CrossRef CAS PubMed.
- C. Smith, Natural antioxidants in prevention of accelerated ageing: a departure from conventional paradigms required, J. Physiol. Biochem., 2018, 74, 549–558 CrossRef CAS PubMed.
- T. Breiter, C. Laue, G. Kressel, S. Gröll, U. H. Engelhardt and A. Hahn, Bioavailability and antioxidant potential of rooibos flavonoids in humans following the consumption of different rooibos formulations, Food Chem., 2011, 128, 338–347 CrossRef CAS.
- G. L. Hostetler, R. A. Ralston and S. J. Schwartz, Flavones: Food Sources, Bioavailability, Metabolism, and Bioactivity, Adv. Nutr., 2017, 8, 423–435 CrossRef CAS.
- E. R. Whittemore, D. T. Loo, J. A. Watt and C. W. Cotmans, A detailed analysis of hydrogen peroxide-induced cell death in primary neuronal culture, Neuroscience, 1995, 67, 921–932 CrossRef CAS.
- F. H. Calderón, A. Bonnefont, F. J. Muñoz, V. Fernández, L. A. Videla and N. C. Inestrosa, PC12 and Neuro 2a cells have different susceptibilities to acetylcholinesterase-amyloid complexes amyloid25–35 fragment glutamate hydrogen peroxide, J. Neurosci. Res., 1999, 56, 620–631 CrossRef.
- K. T. LePage, R. W. Dickey, W. H. Gerwick, E. L. Jester and T. F. Murray, The neurotoxic lipopeptide kalkitoxin interacts with voltage-sensitive sodium channels in cerebellar granule neurons, Crit. Rev. Neurobiol., 2005, 17, 27–50 CrossRef CAS.
- J. Pyrzanowska, I. Joniec-Maciejak, K. Blecharz-Klin, A. Piechal, D. Mirowska-Guzel, I. Fecka and E. Widy-Tyszkiewicz, Long-term administration of Aspalathus linearis infusion affects spatial memory of adult Sprague-Dawley male rats as well as increases their striatal dopamine content, Neurosci. Lett., 2021, 747, 135680 CrossRef CAS PubMed.
- G. Cásedas, E. González-Burgos, C. Smith, V. López, M. P. Gómez-Serranillos, G. Cásedas, E. González-Burgos, C. Smith, V. López and M. P. Gómez-Serranillos, Food Chem. Toxicol., 2018, 118, 572–580 (
Food Chem. Toxicol.
, 2018
, 118
, 572–580
) CrossRef PubMed.
- G. Baker, D. Matveychuk, E. M. MacKenzie, A. Holt, Y. Wang and S. Kar, Attenuation of the effects of oxidative stress by the MAO-inhibiting antidepressant and carbonyl scavenger phenelzine, Chem.-Biol. Interact., 2019, 304, 139–147 CrossRef CAS PubMed.
- S. Carradori, D. Secci and J. P. Petzer, MAO inhibitors and their wider applications: a patent review, Expert Opin. Ther. Pat., 2018, 28, 211–226 CrossRef CAS PubMed.
- J. P. M. Finberg, Update on the pharmacology of selective inhibitors of MAO-A and MAO-B: focus on modulation of CNS monoamine neurotransmitter release, Pharmacol. Ther., 2014, 143, 133–152 CrossRef CAS PubMed.
- J. P. M. Finberg and J. M. Rabey, Inhibitors of MAO-A and MAO-B in Psychiatry and Neurology, Front. Pharmacol., 2016, 7, 340 CAS.
- L. Saaby, H.
B. Rasmussen and A. K. Jäger, MAO-A inhibitory activity of quercetin from Calluna vulgaris (L.) Hull, J. Ethnopharmacol., 2009, 121, 178–181 CrossRef CAS.
- M. B. H. Youdim, Monoamine oxidase inhibitors, and iron chelators in depressive illness and neurodegenerative diseases, J. Neural Transm., 2018, 125, 1719–1733 CrossRef CAS PubMed.
- P. Dhiman, N. Malik, E. Sobarzo-Sánchez, E. Uriarte and A. Khatkar, Quercetin and Related Chromenone Derivatives as Monoamine Oxidase Inhibitors: Targeting Neurological and Mental Disorders, Molecules, 2019, 24(3), 418 CrossRef PubMed.
- O. Akinrinmade, S. Omoruyi, D. Dietrich and O. Ekpo, Long-term consumption of fermented rooibos herbal tea offers neuroprotection against ischemic brain injury in rats, Acta Neurobiol. Exp., 2017, 77, 94–105 Search PubMed.
- O. Inanami, T. Asanuma, N. Inukai, T. Jin, S. Shimokawa, N. Kasai, M. Nakano, F. Sato and M. Kuwabara, The suppression of age-related accumulation of lipid peroxides in rat brain by administration of Rooibos tea (Aspalathus linearis), Neurosci. Lett., 1995, 196, 85–88 CrossRef CAS.
- V. L. Singleton, R. Orthofer and R. M. Lamuela-Raventos, Analysis of total phenols and other oxidation substrates and antioxidants by means of folin-ciocalteu reagent, Methods Enzymol., 1999, 299, 152–178 CAS.
- V. López, S. Akerreta, E. Casanova, J. M. García-Mina, R. Y. Cavero and M. I. Calvo, In vitro antioxidant and anti-rhizopus activities of Lamiaceae herbal extracts, Plant Foods Hum. Nutr., 2007, 62, 151–155 CrossRef PubMed.
- J. L. Rodríguez-Chávez, E. Coballase-Urrutia, A. Nieto-Camacho and G. Delgado-Lamas, Antioxidant capacity of “Mexican arnica” Heterotheca inuloides Cass natural products and some derivatives: their anti-inflammatory evaluation and effect on C. elegans life span, Oxid. Med. Cell. Longevity, 2015, 843237 DOI:10.1155/2015/843237.
- T. Mosmann, Rapid colorimetric assay for cellular growth and survival: application to proliferation and cytotoxicity assays, J. Immunol. Methods, 1983, 65, 55–63 CrossRef CAS PubMed.
- I. K. Rhee, M. Van De Meent, K. Ingkaninan and R. Verpoorte, Screening for acetylcholinesterase inhibitors from Amaryllidaceae using silica gel thin-layer chromatography in combination with bioactivity staining, J. Chromatogr. A, 2001, 915, 217–223 CrossRef CAS.
- F. S. Senol, I. Orphan, G. Yilmaz, M. Cicek and B. Sener, Acetylcholinesterase, butyrylcholinesterase, and tyrosinase inhibition studies and antioxidant activities of 33 Scutellaria L. taxa from Turkey, Food Chem. Toxicol., 2010, 48(3), 781–788 CrossRef CAS PubMed.
- R. M. Basnet, D. Zizioli, S. Taweedet, D. Finazzi and M. Memo, Zebrafish Larvae as a Behavioral Model in Neuropharmacology, Biomedicines, 2019, 7(1), 23 CrossRef PubMed.
- S. C. Baraban, M. R. Taylor, P. A. Castro and H. Baier, Pentylenetetrazole induced changes in zebrafish behavior, neural activity and c-fos expression, Neuroscience, 2005, 131, 759–768 CrossRef CAS PubMed.
- A. Dávalos, C. Gómez-Cordovés and B. Bartolomé, Extending applicability of the oxygen radical absorbance capacity (ORAC-fluorescein) assay, J. Agric. Food Chem., 2004, 52(1), 48–54 CrossRef PubMed.
|
This journal is © The Royal Society of Chemistry 2022 |