DOI:
10.1039/D0FO02754E
(Paper)
Food Funct., 2021,
12, 97-106
Wheat germ glycoprotein regionally modulates immunosuppressed mouse intestinal immunity function from early life to adulthood
Received
21st October 2020
, Accepted 23rd November 2020
First published on 25th November 2020
Abstract
Wheat germ glycoprotein (WGP) is widely used due to its nutritional benefits and biological activity. This study evaluated the effects of WGP on intestinal-immunosuppressed mice from early life to adulthood and detected the underlying mechanism. The results revealed that WGP demonstrated no clinical side effects on the body index, serum total IgA level, protein expression and the morphology of intestine in newborn mice. In the phase of life, compared with the cyclophosphamide-treated group (CG), WGP clearly promoted the secretion of sIgA and effectively regulated the cytokine gene (IL-2, IFN-γ, TNF-α, IL-4, IL-6, IL-5, IL-17, and TGF-β1) expression in the intestine. Furthermore, WGP promoted the expression of CD40L and CD40, phosphorylation of IKKα/β and transcription of NF-κB-p65. The data as reported in this present analysis suggest that WGP can improve the intestinal immunity of newborn mice to adulthood via the CD40L-CD40-IKKα/β-NF-κB p65 signaling pathway.
1. Introduction
Exposure to chronic metabolic diseases early in life leads to an increased risk of sustaining this condition well into adulthood.1,2 Some studies point out that frequent use of antibiotics early in life increases the risk of immune diseases in children; for instance, eczema, asthma, and allergies.3 In recent years, numerous observational studies have noted the importance of early-life environment in determining the trajectory of late-stage diseases.4–7 Consequently, it is necessary to protect the body early in life as much as possible. Immunosuppression is an unnatural immune state that compromises the immune response towards antigens and increases a person's susceptibility to a disease.8 The intestine plays a unique role in the innate immune system, not only as a principal place for digesting and absorbing nutrients but also an essential site for resisting microbial infection.9 The immunity of the intestinal tract subsequently emerges as a biological barrier consisting of intestinal mucosal cells, intestinal immune cells and healthy intestinal microbiota.10
Polysaccharides play an important role in people's daily diet, contributing to good health.11 Dietary fibers can improve the intestinal health of their hosts by producing short-chain fatty acids12 and manipulating intestinal microbiota to generate “healthy” compositions.13 Some reports have reported that polysaccharides can regulate immunity by improving intestinal function in early life. Y. Nakamura et al. discovered that dietary fructooligosaccharides improved the immunoglobulin A response and polymeric immunoglobulin receptor expression in the intestines of newborn mice.14 In their study, Juffrie M. reported that fructooligosaccharides are beneficial as they can curtail the duration of diarrhea experienced by children. This is achieved by ensuring that the intestine flora retains a good balance.15
Cyclophosphamide is an alkylating agent mostly used in chemotherapy for the treatment of autoimmune diseases, cancer, and blood and marrow transplantation. The toxic effects of cyclophosphamide originate from damaging the structure of DNA and immune cells, interfering with the proliferation and differentiation of T and B cells, decreasing the number of normal T and B cells as well as inhibiting the cellular and humoral immune responses. It had been demonstrated that cyclophosphamide induced the damage of intestinal mucosa, and inhibited and disrupted the gut immunity. In this study, cyclophosphamide was selected to induce the immunosuppressed model according to the previous methods with some modifications, such as the study by Chen et al. They indicated that Cordyceps sinensis polysaccharides could ameliorate intestinal injury and enhance antioxidant activity in cyclophosphamide-induced intestinal immunosuppressed mice.16 Xie et al. investigated the effects of Dendrobium huoshanense polysaccharide on mice with cyclophosphamide-induced intestinal injury and explored its potential mechanisms.17 Zhao et al. also chose cyclophosphamide to build the immunosuppressed mouse model to investigate the effects of polysaccharides from bee-collected pollen of Chinese wolfberry on the immune response and gut microbiota composition in cyclophosphamide-treated mice.18
Wheat germ is the constituent part of wheat (2%–3%), and it has been demonstrated to wield a significant impact on human health via its antioxidant, anti-cancer, and anti-hyperlipidemic properties. It is considered to be very nutritious due to its nutrients such as unsaturated fatty acids, proteins, and dietary fibers.19–21 A wheat germ glycoprotein (WGP), which is the main component of wheat germ, has been investigated in our previous studies. These analyses illuminated its structure, immunomodulatory activity and simulated gastrointestinal digestion.22–25 Subsequently, this study sets out to analyze the effects of wheat germ glycoprotein on the intestinal immunity in mice from the stages of early life to adulthood.
2. Materials and methods
2.1 Material
Pregnant and specifically pathogen-free Balb/c mice used in this experiment were obtained from Sibeford (Beijing) Biotechnology Co. Ltd (Beijing, China, Certificate number: SCXK (Jing) 2019-0002). IL-2, IL-4, IL-6, IL-10, TNF-α, IFN-γ, IgA and sIgA ELISA kits were provided by Shanghai Enzyme-linked Biotechnology Co., Ltd (China). RIPA lysis buffer, protein marker and BCA protein assay kit were provided by Beijing Ding Guo Chang Sheng Biotechnology Co. Ltd (China). Antibodies against CD40, CD40L, IKKα/β, p-IKKα/β, NF-p65, and β-actin were provided by Santa Cruz Biotechnology (Calif., USA). All the other chemicals used in the experiment were of analytical purity grade. Wheat germ was purchased from the FADA Flour Group in Shandong, China. Wheat germ glycoprotein was obtained from the Laboratory of Food Nutrition and Safety (Tianjin University of Science & Technology, Tianjin, China).
2.2 Experimental design
Ten pregnant and specifically pathogen-free Balb/c mice at day 14 of pregnancy were provided by the Laboratory Animal Research Institute (SPF (Beijing) Biotechnology Co. Ltd) and placed in individually ventilated plastic cages at a temperature of 23 ± 1 °C. Humidity was set to 50%–70% with a 12 h light/dark cycle, and the specimens were given free access to water. Each group was randomly assigned 20 newborn mice. The experimental procedures employed in this study were approved by the Animal Ethics and Welfare Committee (IRMM-DWLL-2019096).
2.3 Animal grouping and immunodeficient mouse model establishment
The newborn mice were randomly divided into three groups as follows: (i) normal group (NG), (ii) cyclophosphamide (80 mg per kg BW) group (CG)-treated with sterile saline, and (iii) mice were induced with cyclophosphamide (80 mg per kg BW) and treated with the wheat germ glycoprotein (120 mg per kg BW per day) group (WCG). The dosage of WGP has been reported in our previous study and particularly the immunomodulating properties23 + cyclophosphamide (80 mg per kg BW) group (WCG), for the 20 mice per treatment group. Immunodeficient mice were induced using the previous methods with minor modifications.16–18,26 A clear description presented as follows: mice in the NG and CG groups were orally gavaged with gastric saline from postnatal day 7 to 21, while mice in the WCG group were orally gavaged with the 120 mg per kg BW per day WGP solution from postnatal day 7 to 21. The mice in each group were weaned at postnatal day 21. Simultaneously, mice in CG and WCG were intraperitoneally injected with cyclophosphamide (80 mg per kg bw) at postnatal day 22 for three consecutive days. Mice were sacrificed at postnatal day 21 (weaning) and day 42, respectively, and their blood, spleen, thymus and intestine were subsequently collected. The thymus and spleen indices were calculated (organ weight (mg)/body weight (g)). Fig. 1 illustrates the detailed experimental design of this mouse-based experiment.
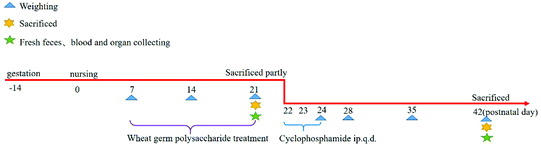 |
| Fig. 1 The schematic of the main experimental design. Parts of mice were sacrificed to collect blood, spleen and intestine at postnatal day 21. The rest of the mice were induced with immunodeficiency by cyclophosphamide and sacrificed at the end of this study. ip., intraperitoneal, q.d., one time per day. The two ends of the horizontal line represent the two compared groups, while the asterisk on the line represents significant difference between groups, n = 20, *P < 0.05, **P < 0.01 vs. NG, #P < 0.05, ##P < 0.01 vs. CG. | |
2.4 Measurement of serum IgA, and IL-2, IL-6, IL-4, TNF-α, IFN-γ, and sIgA in the small intestine
The blood of mice was collected at postnatal days 21 and 42 from the eyeball to prepare the serum. Subsequently, the collected serum was isolated for the IgA assay according to the manufacturer's instructions. Small intestines were flushed and rinsed with PBS (pH 7.4). Ten-milligram flushed-small intestine was completely homogenized with a homogenizer, and then centrifuged at 2000 rpm for 5 min at 4 °C. The supernatant was dispensed into a 1.5 mL EP tube and assayed by IL-2, IL-6, IL-4, IFN-γ, TNF-α and sIgA ELISA kits according to the manufacturer's instructions. It was then measured at a wavelength of 450 nm via a microplate reader.
2.5 RNA extraction and RT-qPCR
Total RNA was extracted from the small intestinal tissue with the TRIzol reagent (Invitrogen) following the manufacturer's instruction, and cDNA was obtained using an Invitrogen Kit according to the manufacturer's protocol. The purity of the RNA was determined using a Q5000 spectrophotometer (Quwell), and the ratio of absorbance at 260/280 nm in all samples was in the 1.8–2.0 range. Using total RNA as the template, an Invitrogen reverse transcription kit was employed to reverse-transcribed into cDNA. Then, qPCR was done using an ABI PRISM 7500 Real-Time PCR System. Data were analyzed using the 2−ΔΔCt method. For the purpose of retaining the housekeeping gene for normalization, GAPDH was employed. Primer sequences are summarized in Table 2.
2.6 Pathological observation of intestinal tissue
After the mice were sacrificed, their intestine tissues were removed quickly and fixed with 4% neutral buffered formalin for 24 h. Subsequently, fixed tissues were embedded with paraffin and processed by hematoxylin–eosin (H&E) staining. Following this, they were examined under light microscopy, as previously described.27
2.7 Western blot analysis for IKKα/β, p-IKKα/β, CD40L, CD40, and NF-κB p65
Small intestines (50 mg–100 mg) of mice were prepared and lysed with RIPA lysis buffer (500 μL–100 μL) for 30 min on ice and centrifuged at 12
000 rpm for 10 min at 4 °C. The supernatant was absorbed gently and transferred to a new centrifuge tube on ice. The concentration and purity of the protein were measured using the BCA protein assay kit and 10% SDS Tris-Glycine gels. Following that, gels were transferred onto PVDF membranes in a transfer buffer (25 mM Tris [pH 8.0] containing 192 mmol L−1 glycine and 20% methanol) using a Bio-Rad Trans-Blot Cell (Bio-Rad, Hercules, CA, USA) under a constant current of 300 mA. It was blocked for 1 h at room temperature in a PBS blocking buffer (containing 5% non-fat milk). This was followed by incubation with primary antibody (anti-IKKα/β, 1
:
1000; anti-p-IKKα/β, 1
:
1000; anti-CD40L, 1
:
1000; anti-CD40, 1
:
1000; anti-NF-κB p65, 1
:
1000; anti-β-actin, 1
:
1000) overnight at 4 °C. After being washed with PBS containing 0.05% Tween-20, the strips were incubated with secondary HRP-conjugated goat anti-mouse IgG (1
:
5000) for 1 h. The ECL detection system visualized the antigen–antibody complexes, and bands were quantified using the ImageJ software.
2.8 Statistical analysis
The result was presented as mean values ± standard deviations and analyzed by the SPSS16.0 software (SPSS, Chicago, IL, USA). The significance of differences between the two groups was evaluated using the Student's t-test, while the differences among multiple groups were analyzed using the one-way analysis of variance (ANOVA), followed by the Bonferroni post-hoc test. Findings of P < 0.05 and P < 0.01 were considered as a confirmation that a significant difference existed.
3. Results and discussion
3.1 Bodyweight and organ indexes of mice
At postnatal day 21, the body weights of the mice in all groups displayed a steady increase with no significant variations (Fig. 2A). Simultaneously, no significant spleen and thymus differences were detected in all groups (P > 0.05) (Fig. 2B). However, the bodyweight of mice in CG at postnatal day 24 revealed a significant decline compared to WCG. Nevertheless, no significant difference was observed among the groups at postnatal days 35 and 42, and this was attributed to the body weights of mice gradually increasing with age.
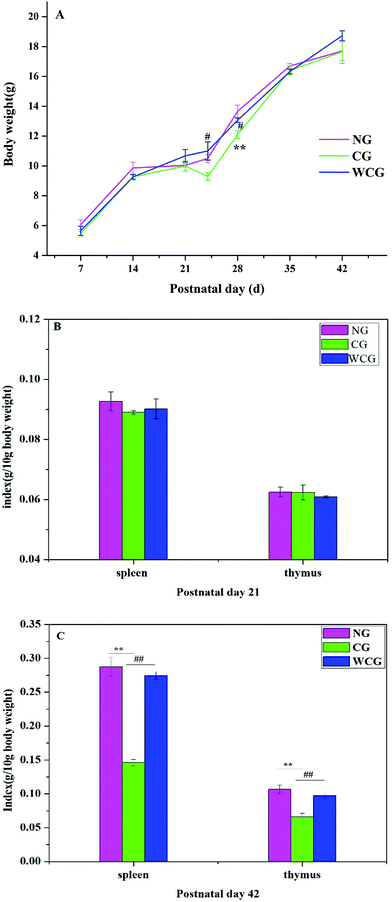 |
| Fig. 2 Changes in the body index in the three groups of female mice. The body index during the experiment included body weight (A), spleen and thymus index at postnatal day 21 (B), and spleen and thymus index at postnatal day 42 (C). Data are expressed as mean ± SD. The two ends of the horizontal line represent the two compared groups, while the asterisk on the line represents the significant difference between groups, *P < 0.05, **P < 0.01 vs. NG, #P < 0.05, ##P < 0.01 vs. CG. | |
At postnatal day 42, all spleens and thymus of the sacrificed mice were weighed. Spleens and thymus indices of mice in CG were significantly lower than those of the other groups (P < 0.01), and no significant difference emerged between NG and WCG (P > 0.05).
3.2 Effect on cytokines levels in the intestinal tissue
The cytokine analysis results are summarized in Table 1. At postnatal day 21, the level of the four cytokines (IL-2, IL-4, IL-6, IFNγ and TNFα) in the intestinal tissue in all groups revealed no significant difference (P > 0.05). At postnatal day 42, compared to the CG mice, the wheat germ glycoprotein treatment led to an increases in the following: 5.57% ± 0.84% IL-2; 11.37% ± 0.46% for IL-4; 6.16% ± 1.30% for IL-6; 1.69% ± 0.01% for IFNγ; and 0.82% ± 0.18% for TNFα. No significant difference was found between the mice of NG and WCG (P > 0.05), which confirmed that WGP could stimulate the secretion of the four cytokines. In the present study, moderate interleukins are necessary to regulate cells for activation and function purposes. IL-2 led to two outcomes: first, the clonal expansion of T cells and promoting the functions of T cells and B cells,28 and second, IL-4 can stimulate the survival of double-negative T cells.29 IFN-γ and IL-2 are Th1-associated cytokines as well as IL-4, while IL-6 is Th2-associated cytokines. It is important to note that the balance of Th1/Th2 plays an important role in regulating the balance of cellular immunity and humoral immunity.30 Xie et al. suggested that Dendrobium huoshanense polysaccharide could ameliorate the intestinal immunological barrier function in two ways: first, promoting Th1-type cytokines and pro-inflammatory cytokines, and second, increasing Th2-type cytokines and anti-inflammatory cytokines to maintain the intestinal immune homeostasis.31 Consequently, this outcome indicated that wheat germ glycoprotein can potentially regulate the balance of Th1/Th2 cells.
Table 1 Effect of WGP on the levels of cytokines in intestinal tissue
Day |
Group |
IL2 (pg mL−1) |
IL4 (pg mL−1) |
IL6 (pg mL−1) |
IFNγ (pg mL−1) |
TNFα (pg mL−1) |
*P < 0.05, **P < 0.01 vs. NG, #P < 0.05, ##P < 0.01 vs. CG. |
21 |
NG |
165.67 ± 1.43 |
98.06 ± 1.05 |
115.82 ± 0.71 |
730.29 ± 1.31 |
684.30 ± 0.99 |
CG |
164.64 ± 1.78 |
97.81 ± 0.85 |
115.21 ± 1.64 |
729.17± 1.15 |
682.93 ± 0.10 |
WCG |
163.03 ± 0.40 |
98.15 ± 0.71 |
115.09 ± 0.70 |
728.23 ± 0.43 |
683.93 ± 0.10 |
42 |
NG |
151.76 ± 0.69 |
115.11 ± 1.23 |
118.0 ± 1.23 |
737.29 ± 0.10 |
779.84 ± 0.58 |
CG |
144.80 ± 0.56** |
102.23 ± 0.86** |
109.64 ± 0.67** |
723.79 ± 1.01** |
770.93 ± 0.91** |
WCG |
152.87 ± 0.63## |
113.85 ± 0.48## |
116.39 ± 0.71# |
736.00 ± 1.11## |
777.21 ± 0.51## |
Table 2 Primer sequences used for RT-qPCR
Gene |
Direction |
Primer sequences (5′→3′) |
Products (bp) |
Annealing temperature (°C) |
GAPDH |
Forward |
GTATCGGACGCCTGGTTAC |
141 |
62 |
Reverse |
CTGTGCCGTTGAACTTGCC |
IL-5 |
Forward |
TGCAGTGTGAATGAGAGCCA |
170 |
60 |
Reverse |
GTAAACTGGGGGAGGCTTCT |
IL-17 |
Forward |
AGCGTGTCCAAACACTGAGG |
116 |
60 |
Reverse |
GGTTGAGGTAGTCTGAGGGC |
TGF-β1 |
Forward |
AAATCAACGGGATCAGCCCC |
260 |
60 |
Reverse |
GAAGTTGGCATGGTAGCCCT |
Reverse |
CTCTCCTCAACAGCCACTCG |
3.3 IgA in serum and sIgA in the intestinal determination
IgA is the most common type of immunoglobulin in the human body secreted by activated B cells and plays a key role in the mucosal immunity and the body's anti-infection tolerance and protection strategies.32 Secretory IgA (sIgA) is secreted by plasma cells, which can be directly transported by intestinal epithelial cells and actively secreted into intestinal mucosa to prevent toxins and pathogens from adhering to intestinal epithelium.33 In this study, the result of IgA is shown in Fig. 3. At postnatal day 21, the levels of IgA and sIgA in all groups reported no significant difference (P > 0.05, Fig. 3A). However, after the WGP treatment, the level of IgA did increase significantly between WCG and NG (P < 0.05). Furthermore, the level of sIgA in WCG mice was also significantly higher than that in CG (P < 0.01, Fig. 3B). Luo et al. noted that a small quantity of sIgA in the gut and IgA in the immunodeficiency group may be due to the decrease in B cells secreting antibodies locally and peripherally.10 Furthermore, these researchers indicated that after administering Dendrobium huoshanense polysaccharide (GXG), an up-regulated secretion of IgA into the intestinal lumen occurred. This outcome strongly suggested that wheat germ glycoprotein could regulate the expression of sIgA and IgA in immunodeficient mice.
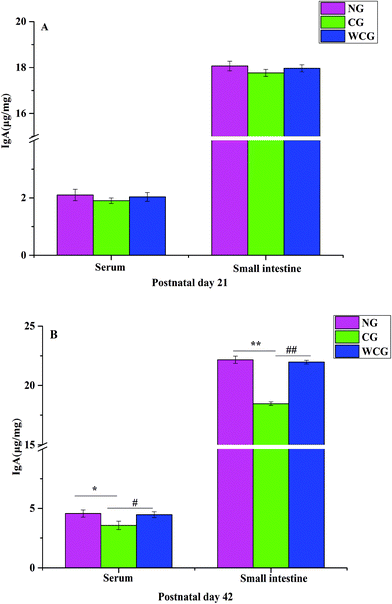 |
| Fig. 3 The concentration of IgA in blood (A) and sIgA in the intestine (B) of female mice. Data are expressed as mean ± SD. The two ends of the horizontal line represent the two compared groups, while the asterisk on the line represents the significant difference between groups, *P < 0.05, **P < 0.01 vs. NG, #P < 0.05, ##P < 0.01 vs. CG. | |
3.4 Effects of WGP on the levels of IL-5, IL-17 and TGF-β1 mRNA in small intestinal tissue
As shown in Fig. 4, no significant difference emerged in the mRNA levels of IL-5, IL-17 and TGF-β1 of mice among all groups at postnatal day 21. However, obvious enhancements appeared at postnatal day 42, and in the mice exposed to WGP, there was a significant increase in the levels of cytokines including IL-5, IL-17 and TGF-β1 compared with the mice of CG (P < 0.01). Moreover, the relative mRNA levels of IL-5, IL-17 and TGF-β1 in the mice of CG fell significantly compared with that in the mice of NG (P < 0.01). Th2 produces IL-5, which promotes the clearance of extracellular parasites.34 The RT-qPCR results indicated that WGP could promote the Th2-associated cytokine (IL-5) expression of mice at postnatal day 42. Intestinal mucosal immunity strikes a good balance between inflammatory and drug-resistant responses. This helps to, first, avoid overreaction to harmless luminal antigens, and second, to promote protective immune responses towards pathogens.35 T cells are known to be a potent source of TGF-β1, which is an immunosuppressive cytokine produced by multiple cell types. Moreover, one aspect of the effect of TGF-β1 consists of suppressing Th1 and Th2 differentiation, which makes diversion to IL-17 differentiation possible.36 In this study, WGP significantly increased the levels of IL-17 and TGF-β1 mRNA compared with the mice of CG.
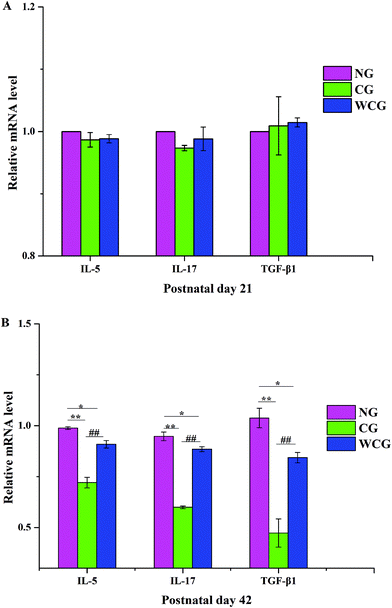 |
| Fig. 4 The mRNA levels of IL-5, IL-17 and TGF-β1 in female mice at postnatal day 21 and postnatal day 42, respectively. Data are expressed as mean ± SD, and the two ends of the horizontal line represent the two compared groups. The asterisk on the line represents the significant difference between groups, *P < 0.05, **P < 0.01 vs. NG, #P < 0.05, ##P < 0.01 vs. CG. | |
3.5 Histological analysis of the intestinal morphology using H&E
Natural polysaccharides in the diet are considered to be beneficial nutrients, which help the intestinal mucosal barrier function by promoting the development and functional maturation of intestinal mucosal epithelial cells37 and mucosal immune cells.38 Therefore, the simply structured intestines in the digestive tract play particularly important roles in immune resistance and nutritional metabolism.39,40 The intestinal physiological status can reflect the intestine's function. In the present study, the histological assay served to analyze the influences of wheat germ glycoprotein on the structural development of intestine from early in life to adulthood. The histological analysis of ileum and colon in mice at postnatal days 21 and 42 is displayed in Fig. 5. The ileum and colon in all groups were well-shaped at postnatal day 21. At postnatal day 42, the ileum and colon in CG revealed the loss of the regular villi structure compared with NG mice. Furthermore, there were no remarkable lesions in the mice treated with wheat germ glycoprotein compared with the NG mice (P > 0.05). These results mean that wheat germ glycoprotein can play an immunomodulatory function by affecting the intestine structure. Luo et al. have reported that when using H&E staining the count of infiltrating lymphocytes dropped significantly on the swollen side of the immunodeficiency group compared to the control group.41 In the study by Cheng et al. who employed H&E staining, when compared to the normal control group, they found that the ileal villi lesions and colonic crypt atrophy in the immunodeficient mice were significantly less (P < 0.05).42
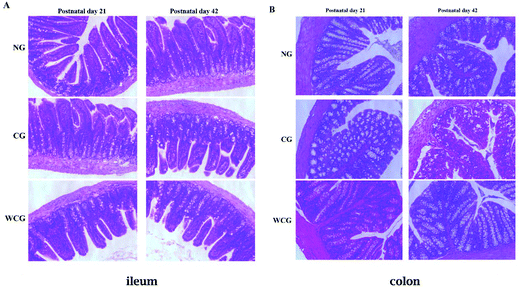 |
| Fig. 5 Intestinal tissue injury of mice at postnatal days 21 and 42. The H&E staining profiles of ileum (A) and colon (B) (20× magnification) in the mice of three groups were analyzed by a fluorescence microscope (Ti-U, Nikon, Japan). Scale bar = 100 μm. | |
3.6 Protein expression levels in the small intestine using western blot
CD40L is mainly expressed in activated CD4+T lymphocytes when binding to CD40 on the surface of B cells, and then activates the NF-κB signaling pathway. Consequently, the expression of CD40L and CD40 is a key point in solving the mechanism of wheat germ glycoprotein in regulating the intestinal immunity. The levels of CD40L, CD40, IKKα/β, pho-IKKα/β, and NF-κB p65 proteins in the small intestine were evaluated through the western-blotting assay. The results are illustrated in Fig. 6 where the changes in protein levels in the NG, CG, and WCG groups were not obvious at postnatal day 21 (Fig. 6A–D). At postnatal day 42 (Fig. 6E–H), compared with the normal group, the CD40L, CD40, IKKα/β, pho-IKKα/β, and NF-κB p65 protein expressions of mice in CG were much lower. However, CD40L, CD40, IKKα/β, pho-IKKα/β, and NF-κB p65 increased following wheat germ glycoprotein treatment. This suggests that WGP could enhance the intestinal immune function by promoting the levels of CD40L, CD40, IKKα/β, pho-IKKα/β, and NF-κB p65 proteins.
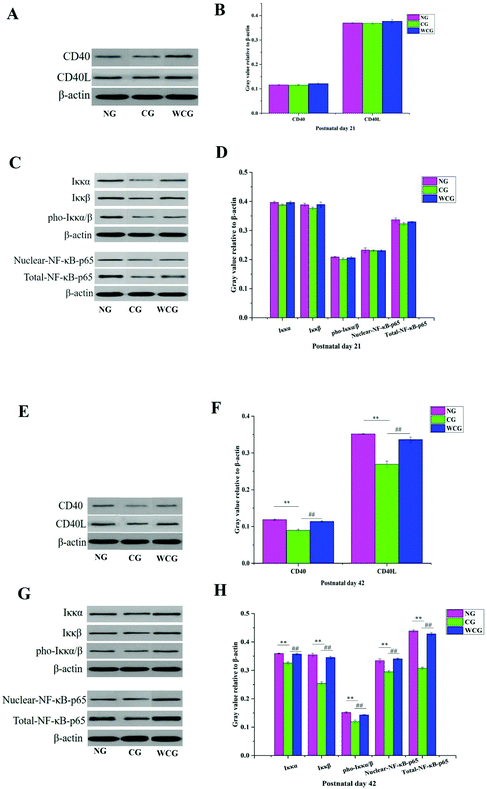 |
| Fig. 6 The relative expression levels of the immune-related protein in mice small intestine were determined by western blot. The representative gene expression of CD40 and CD40L proteins at postnatal day 21 (A); quantitative analysis of CD40 and CD40L at postnatal day 21 (B); representative gene expression of IKKα/β, p-IKKα/β, NF-p65, and nuclear-NF-p65 protein at postnatal day 21 (C); quantitative analysis of IKKα/β, p-IKKα/β, NF-p65, and nuclear-NF-p65 at postnatal day 21 (D); representative gene expression of CD40 and CD40L protein at postnatal day 42 (E); quantitative analysis of CD40 and CD40L at postnatal day 42 (F); representative gene expression of IKKα/β, p-IKKα/β, NF-p65, and nuclear-NF-p65 proteins at postnatal day 42 (G); quantitative analysis of IKKα/β, p-IKKα/β, NF-p65, and nuclear-NF-p65 at postnatal day 42 (H); data are presented as mean ± SD. The two ends of the horizontal line represent the two compared groups, while the asterisk on the line represents the significant difference between groups, *P < 0.05, **P < 0.01 vs. NG, #P < 0.05, ##P < 0.01 vs. CG. | |
Previous studies have suggested that B-cell-activating with CD40 can induce the phosphorylation of IκBα bound to cytosolic NF-κB.43 IκB exists in the cytoplasm in a resting state and forms a complex with NF-κB in the form of inhibitory units. The activated IκB kinase complex (IKK) results in the phosphorylation of IκB. NF-κB was liberated due to the phosphorylation of IκBα degrading. This was in addition to the liberating NF-κB being translocated to the nucleus and activating target genes. In this study, the wheat germ glycoprotein treatment could promote the expression of IKKα/β and its phosphorylation, and accelerate the NF-κB p65 entry into the nucleus, thereby regulating the transcription of IgA genes. The idea that the signaling pathways of wheat germ glycoprotein regulate the intestinal immunity of newborn mice to adulthood is depicted in Fig. 7.
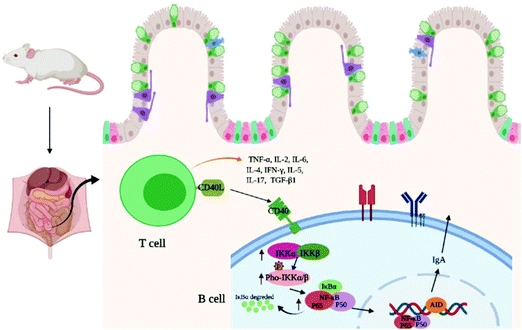 |
| Fig. 7 The speculated signaling pathways of wheat germ glycoprotein regulate the intestinal immunity function of newborn mice to adulthood. | |
4. Conclusions
This study investigated the effect of wheat germ glycoprotein on intestinal immunity in mice from early life to adulthood and further confirmed our previous speculation that wheat germ glycoprotein represented a type of immunostimulatory glycoprotein. This study's results confirmed that wheat germ glycoprotein could effectively regulate the cytokines gene expression including IL-2, IFN-γ, TNF-α, IL-4, IL-6, IL-5, IL-17, and TGF-β1. It can also up-regulate the expression of IgA and sIgA, and promote IKKα/β, p-IKKα/β, CD40L, CD40 and NF-κB p65 protein expressions. Finally, our study discovered that wheat germ glycoprotein-treated mice in early life could effectively improve their intestinal immunity to adulthood.
Conflicts of interest
The authors declare no conflict of interest.
Acknowledgements
Project funded by China Postdoctoral Science Foundation [2019M651051]; the fund of the Beijing Laboratory of Food Quality and Safety, School of Food and Chemical Engineering, Beijing Technology and Business University [FQS-201906].
References
- L. Y. Zhou, X. H. Xiao, Q. Zhang, J. Zheng, M. Li, X. J. Wang, M. Q. Deng, X. Zhai and J. Y. Liu, Gut microbiota might be a crucial factor in deciphering the metabolic benefits of perinatal genistein consumption in dams and adult female offspring, Food Funct., 2019, 10, 4505–4521 RSC.
- V. E. Ruiz, T. Battaglia, Z. D. Kurtz, L. Bijnens, A. Ou, I. Engstrand, X. Zheng, T. Iizumi, B. J. Mullins, C. L. Muller, K. Cadwell, R. Bonneau, G. I. Perez-Perez and M. J. Blaser, A single early-in-life macrolide course has lasting effects on murine microbial network topology and immunity, Nat. Commun., 2017, 8, 518 CrossRef.
- X. Luo, Z. Pan, S. Luo, Q. Liu, S. Huang, G. Yang, F. Nong, Y. Fu, X. Deng and L. Zhou, Effects of ceftriaxone-induced intestinal dysbacteriosis on regulatory T cells validated by anaphylactic mice, Int. Immunopharmacol., 2018, 60, 221–227 CrossRef CAS.
- K. Y. Yam, E. F. G. Naninck, M. R. Abbink, S. E. la Fleur, L. Schipper, J. C. van den Beukel, A. Grefhorst, A. Oosting, E. M. van der Beek, P. J. Lucassen and A. Korosi, Exposure to chronic early-life stress lastingly alters the adipose tissue, the leptin system and changes the vulnerability to western-style diet later in life in mice, Psychoneuroendocrinology, 2017, 77, 186–195 CrossRef CAS.
- M. C. Arrieta, L. T. Stiemsma, N. Amenyogbe, E. M. Brown and B. Finlay, The intestinal microbiome in early life: health and disease, Front. Immunol., 2014, 5, 427 Search PubMed.
- V. E. Ruiz, T. Battaglia, Z. D. Kurtz, L. Bijnens, A. Ou, I. Engstrand, X. Zheng, T. Iizumi, B. J. Mullins, C. L. Muller, K. Cadwell, R. Bonneau, G. I. Perez-Perez and M. J. Blaser, A single early-in-life macrolide course has lasting effects on murine microbial network topology and immunity, Nat. Commun., 2017, 8, 518 CrossRef.
- S. Schaffert and P. Khatri, Early life immunity in the era of systems biology: understanding development and disease, Genome Med., 2018, 10, 88 CrossRef.
- J. Xie, Q. Yu, S. Nie, S. Fan and M. Xie, Effects of Lactobacillus plantarum NCU116 on Intestine Mucosal Immunity in Immunosuppressed Mice, J. Agric. Food Chem., 2015, 63, 10914 CrossRef CAS.
- Y. Li, Y. Yang, Q. Ji, J. Song, L. Wang, B. Liu, J. Wang and C. Li, The function of Apostichopus japonicas catalase in sea cucumber intestinal immunity, Aquaculture, 2020, 521, 735103 CrossRef CAS.
- X. Luo, Y. Zheng, R. Wen, X. Deng, L. Zhou and H. Liao, Effects of ceftriaxone induced intestinal dysbacteriosis on lymphocytes in different tissues in mice, Immunobiology, 2016, 221, 994–1000 CrossRef CAS.
- A. E. Quiros-Sauceda, H. Palafox-Carlos, S. G. Sayago-Ayerdi, J. F. Ayala-Zavala, L. A. Bello-Perez, E. Alvarez-Parrilla, L. A. de la Rosa, A. F. Gonzalez-Cordova and G. A. Gonzalez-Aguilar, Dietary fiber and phenolic compounds as functional ingredients: interaction and possible effect after ingestion, Food Funct., 2014, 5, 1063–1072 RSC.
- T. M. Cantu-Jungles, T. R. Cipriani, M. Iacomini, B. R. Hamaker and L. M. C. Cordeiro, A pectic polysaccharide from peach palm fruits (Bactris gasipaes) and its fermentation profile by the human gut microbiota in vitro, Bioact. Carbohydr. Diet. Fibre, 2017, 9, 1–6 CrossRef CAS.
- X. Xu, P. Xu, C. Ma, J. Tang and X. Zhang, Gut microbiota, host health, and polysaccharides, Biotechnol. Adv., 2013, 31, 318–337 CrossRef CAS.
- Y. Nakamura, S. Nosaka, M. Suzuki, S. Nagafuchi, T. Takahashi, T. Yajima, N. Takenouchi-Ohkubo, T. Iwase and I. Moro, Dietary fructooligosaccharides up-regulate immunoglobulin A response and polymeric immunoglobulin receptor expression in intestines of infant mice, Clin. Exp. Immunol., 2004, 137, 52–58 CrossRef CAS.
- M. Juffrie, Fructooligosaccharide and diarrhea, Biosci. Microflora, 2002, 21, 31–34 CrossRef CAS.
- S. Chen, J. Wang, Q. Fang, N. Dong and S. Nie, Polysaccharide from natural Cordyceps sinensis ameliorated intestinal injury and enhanced antioxidant activity in immunosuppressed mice, Food Hydrocolloids, 2019, 89, 661–667 CrossRef CAS.
- S. Z. Xie, B. Liu, H. Y. Ye, Q. M. Li, L. H. Pan, X. Q. Zha, J. Liu, J. Duan and J. P. Luo, Dendrobium huoshanense polysaccharide regionally regulates intestinal mucosal barrier function and intestinal microbiota in mice, Carbohydr. Polym., 2019, 206, 149–162 CrossRef CAS.
- Y. Zhao, Y. Yan, W. Zhou, D. Chen, K. Huang, S. Yu, J. Mi, L. Lu, X. Zeng and Y. Cao, Effects of polysaccharides from bee collected pollen of Chinese wolfberry on immune response and gut microbiota composition in cyclophosphamide-treated mice, J. Funct. Foods, 2020, 72, 104057 CrossRef CAS.
- Li.-Ya Niu, S.-T. Jiang, Li.-J. Pan and M. Pang, Characterization of Wheat Germ Oil in Terms of Volatile Compounds, Lipid Composition, Thermal Behavior, and Structure, Int. J. Food Prop., 2013, 16, 1740–1749 CrossRef CAS.
- S. Ru, Z. Zhang, X. Hu, Q. Xing and W. Zhuo, Effect of wheat germ flour addition on wheat flour, dough and Chinese steamed bread properties, J. Cereal Sci., 2015, 64, 153–158 CrossRef.
- K. X. Zhu, X. P. Wang and X. N. Guo, Isolation and characterization of zinc-chelating peptides from wheat germ protein hydrolysates, J. Cereal Sci., 2015, 12, 23–32 CAS.
- L. Y. Yun, T. Wu, R. Liu, K. Li and M. Zhang, Structural Variation and Microrheological Properties of a Homogeneous Polysaccharide from Wheat Germ, J. Agric. Food Chem., 2018, 66, 2977–2987 CrossRef CAS.
- L. Y. Yun, T. Wu, Q. Li and M. Zhang, Dietary supplementation with purified wheat germ glycoprotein improve immunostimulatory activity in cyclophosphamide induced Balb/c mice, Int. J. Biol. Macromol., 2018, 118, 1267–1275 CrossRef CAS.
- L. Y. Yun, S. Wang, T. Wu, Q. Li and M. Zhang, Structural characterization of a novel glycoprotein in wheat germ and its physicochemical properties, Int. J. Biol. Macromol., 2018, 117, 1058–1065 CrossRef CAS.
- L. Y. Yun, D. Li, L. Yang and M. Zhang, Hot water extraction and artificial simulated gastrointestinal digestion of wheat germ polysaccharide, Int. J. Biol. Macromol., 2019, 123, 174–181 CrossRef CAS.
- C. Tang, J. Sun, B. Zhou, C. Jin, J. Liu, J. Kan, C. Qian and N. Zhang, Effects of polysaccharides from purple sweet potatoes on immune response and gut microbiota composition in normal and cyclophosphamide treated mice, Food Funct., 2018, 9, 937–950 RSC.
- M. Xue, Y. Liu, R. Lyu, N. Ge, M. Liu, Y. Ma and H. Liang, Protective effect of aplysin on liver tissue and the gut microbiota in alcohol-fed rats, PLoS One, 2017, 12, e0178684 CrossRef.
- F. S. Laroux, K. P. Pavlick, R. E. Wolf and M. B. Grisham, Dysregulation of intestinal mucosal immunity: implications in inflammatory bowel disease, News Physiol. Sci., 2001, 16, 272 CAS.
- H. Kato and A. Perl, Mechanistic Target of Rapamycin Complex 1 Expands Th17 and IL-4 + CD4-CD8- Double-Negative T Cells and Contracts Regulatory T Cells in Systemic Lupus Erythematosus, J. Immunol., 2014, 192, 4134–4144 CrossRef CAS.
- S. Z. Xie, B. Liu, D.-D. Zhang, X. Zha, L.-H. Pan and J. Luo, Intestinal immunomodulating activity and structural characterization of a new polysaccharide from stems of Dendrobium officinale, Food Funct., 2016, 7, 2789–2799 RSC.
- S. Z. Xie, B. Liu, H. Y. Ye, Q. M. Li, L. H. Pan, X. Q. Zha, J. Liu, J. Duan and J. P. Luo, Dendrobium huoshanense polysaccharide regionally regulates intestinal mucosal barrier function and intestinal microbiota in mice, Carbohydr. Polym., 2019, 206, 149–162 CrossRef CAS.
- R. Zhao, N. Cheng, P. A. Nakata, L. Zhao and Q. Hu, Consumption of polysaccharides from Auricularia auricular modulates the intestinal microbiota in mice, Food Res. Int., 2019, 123, 383–392 CrossRef CAS.
- N. Xiong and S. Hu, Regulation of intestinal IgA responses, Cell. Mol. Life Sci., 2015, 72, 2645–2655 CrossRef CAS.
- R. Martin, A. J. Nauta, K. Ben Amor, L. M. Knippels, J. Knol and J. Garssen, Early life: gut microbiota and immune development in infancy, Benefic. Microbes, 2010, 1, 367–382 CrossRef CAS.
- A. Perez-Lopez, J. Behnsen, S.-P. Nuccio and M. Raffatellu, Mucosal immunity to pathogenic intestinal bacteria, Nat. Rev. Immunol., 2016, 16, 135–148 CrossRef CAS.
- M. Veldhoen, R. J. Hocking, C. J. Atkins, R. M. Locksley and B. Stockinger, TGFβ in the context of an inflammatory cytokine milieu supports de novo differentiation of IL-17-producing T cells, Immunity, 2006, 24, 179–189 CrossRef CAS.
- T. Zuo, L. Cao, C. Xue and Q.-J. Tang, Dietary squid ink polysaccharide induces goblet cells to protect small intestine from chemotherapy induced injury, Food Funct., 2015, 6, 981–986 RSC.
- A. L. Maldonado-Contreras and B. A. Mccormick, Intestinal epithelial cells and their role in innate mucosal immunity, Cell Tissue Res., 2011, 343, 5–12 CrossRef CAS.
- S. Wu, G. Wang, E. R. Angert, W. Wang, W. Li, H. Zou, S. Wu, G. Wang, E. R. Angert and W. Wang, Composition, Diversity, and Origin of the Bacterial Community in Grass Carp Intestine, PLoS One, 2012, 7, e30440 CrossRef CAS.
- J. Zhu, W. Dai, Q. Qiu, C. Dong, J. Zhang and J. Xiong, Contrasting Ecological Processes and Functional Compositions Between Intestinal Bacterial Community in Healthy and Diseased Shrimp, Microb. Ecol., 2016, 72, 975–985 CrossRef.
- X. Luo, Z. Pan, S. Luo, Q. Liu, S. Huang, G. Yang, F. Nong, Y. Fu, X. Deng and L. Zhou, Effects of ceftriaxone-induced intestinal dysbacteriosis on regulatory T cells validated by anaphylactic mice, Int. Immunopharmacol., 2018, 60, 221–227 CrossRef CAS.
- R. Cheng, J. Guo, F. Pu, C. Wan, L. Shi, H. Li, Y. Yang, C. Huang, M. Li and F. He, Loading ceftriaxone, vancomycin, and Bifidobacteria bifidum TMC3115 to neonatal mice could differently and consequently affect intestinal microbiota and immunity in adulthood, Sci. Rep., 2019, 9, 3254 CrossRef.
- U. Siebenlist, K. Brown and E. Claudio, Control of lymphocyte development by nuclear factor-κB, Nat. Rev. Immunol., 2005, 5, 435–445 CrossRef CAS.
|
This journal is © The Royal Society of Chemistry 2021 |