DOI:
10.1039/D0FO02629H
(Paper)
Food Funct., 2021,
12, 417-425
Demonstrating the involvement of an active efflux mechanism in the intestinal absorption of chlorogenic acid and quinic acid using a Caco-2 bidirectional permeability assay†
Received
7th October 2020
, Accepted 4th December 2020
First published on 7th December 2020
Abstract
Scope: Chlorogenic acid (5-caffeoylquinic acid), the most prominent polyphenolic compound in coffee, has been attributed multiple health-promoting effects such as anti-inflammatory, antidiabetic and antioxidative effects. These effects are dependent on the bioavailability of chlorogenic acid, which is determined by the pharmacokinetic properties: absorption, distribution, metabolism and excretion (ADME). In order to have a better understanding of the biological properties of chlorogenic acid and to optimize formulation and dosing of chlorogenic acid-containing food supplements, information on the absorption of chlorogenic acid and its microbial biotransformation products is of essence. Methods and results: In the present work, the intestinal absorption of chlorogenic acid and quinic acid, one of its most prominent intestinal biotransformation products, was studied by an in vitro permeability assay using a human Caco-2 cell line model. For both chlorogenic acid and quinic acid, the involvement of an active efflux mechanism was demonstrated, suggesting an overall low intestinal absorption. Conclusions: An overall low intestinal absorption for chlorogenic acid and quinic acid was reported given the involvement of an active efflux mechanism. These findings could aid in the development of optimal formulation and dosing strategies of chlorogenic acid in food supplements in order to obtain beneficial health effects.
1. Introduction
The term chlorogenic acids is the collective of naturally occurring phenolic compounds composed of an ester of quinic acid with one or more hydroxycinnamic acids such as caffeic acid and ferulic acid.1 The predominant species in the human diet is chlorogenic acid (5-caffeoylquinic acid), the ester of caffeic acid and quinic acid. It is the major polyphenolic compound found in coffee and is also present in mate tea, fruits and vegetables.2,3 Multiple in vivo animal studies and human clinical trials have attributed chlorogenic acid health-promoting properties such as anti-inflammatory,3 antioxidative,4 antidiabetic5 and antihypertensive effects.6 A meta-analysis including 25 case-control and 16 cohort studies7 and epidemiological data8 suggest an inverse association between coffee consumption and colorectal cancer incidence, but also beneficial effects such as prevention of coronary heart disease and some cancers were described.8,9 Quinic acid, one of the most prominent microbiotic biotransformation products of chlorogenic acid, is attributed indirect antioxidative effects as it is able to induce the antioxidant metabolism by enhancing the synthesis of tryptophan and nicotinamide in the gastrointestinal tract. Increased production of these compounds will lead to DNA repair enhancement and NF-κB inhibition.10,11 Furthermore, neuroprotective effects on aluminum chloride-induced dementia have been described for quinic acid when administered to rats.12
The health-promoting effects of chlorogenic acid are dependent on its bioavailability, which is determined by the pharmacokinetic properties: absorption, distribution, metabolism and excretion (ADME). In order to obtain a better understanding of the biological properties of chlorogenic acid, multiple studies have investigated the in vivo bioavailability in humans and rats after coffee consumption,9,13,14 oral ingestion of a green coffee extract15 or a chlorogenic acid supplemented diet.16 A study in ileostomy patients recovered 70% of the initial intake of chlorogenic acids in the ileal fluid after coffee consumption, meaning this fraction will reach the large intestine where it will be exposed to microbial biotransformation by the gut microbiome and colonic absorption.14 Furthermore, Stalmach et al.14 reported a higher recovery of microbial biotransformation products of chlorogenic acids in the urine of healthy human volunteers with an intact colon in comparison to the ileostomy patients. These findings substantiate an important role for the gut microbiota in the biotransformation of chlorogenic acids and the colon on absorption of its microbial metabolites.13,14 Accordingly, Farah et al.15 and Gonthier et al.16 studied the bioavailability of chlorogenic acid in humans and rats respectively, including the microbial biotransformation products in the formula of the apparent bioavailability. Farah et al. reported an apparent bioavailability of 33% ± 27% for chlorogenic acid after oral ingestion of a green coffee extract.15 Gonthier and colleagues stated that the bioavailability of chlorogenic acid is highly dependent on its microbial biotransformation as 57% (mol/mol) of the orally ingested dose of chlorogenic acid was recovered as microbial biotransformation products in the plasma. However, the influence of the hepatic metabolism on the detected biotransformation products is neglected in this study.16
In order to have a more profound understanding of the biological properties of chlorogenic acid and to optimize formulation and dosing strategies of chlorogenic acid-containing food supplements, detailed information on the absorption of chlorogenic acid and its microbial biotransformation products is imperative. In vivo bioavailability studies, however, do not provide the necessary information to make statements on the intestinal absorption of chlorogenic acid and its microbial biotransformation products. Firstly, chlorogenic acid is susceptible to microbial biotransformation by the gut microflora leading to multiple phenolic biotransformation products and quinic acid meaning that a low recovery of chlorogenic acid in plasma or urine cannot be necessarily linked to a low intestinal absorption. Furthermore, the measured analytes in the plasma may also result from hepatic biotransformation.17,18 Secondly, instant/brewing coffee and coffee extracts, frequently used in in vivo studies, contain multiple chlorogenic acids including caffeoylquinic acids, dicaffeoylquinic acids, feruloylquinic acids and p-coumaroylquinic acids.13–15 Biotransformation of these chlorogenic acids could lead to common microbial biotransformation products and misleading conclusions on the absorption of chlorogenic acid and/or its microbial biotransformation products.
In vitro permeability studies can provide information on the absorption of chlorogenic acid and microbial biotransformation products without influence of the other pharmacokinetic ADME properties. The human intestinal epithelial Caco-2 cell-line, derived from a colorectal adenocarcinoma, is widely used to mimic the intestinal barrier in vitro.19 Caco-2 cells have the ability to spontaneously differentiate towards a cell monolayer with the characteristic apical brush border with microvilli and adjacent cells with tight junctions.19 Multiple studies have shown a high correlation between the Caco-2 permeability results and the in vivo small intestinal and colonic absorption of orally ingested compounds, which makes them a suitable tool for evaluation and estimation of colonic absorption of chlorogenic acid and microbial biotransformation products.20–23 Additionally, Caco-2 cells lack the expression of cytochrome P450 enzymes, which should prevent further biotransformation of the tested compounds during the permeability assay.24
In the present work, we studied the intestinal absorption of chlorogenic acid in its native form and quinic acid, one of its most prominent intestinal biotransformation products, by an in vitro permeability assay using the Caco-2 cell line. Investigating the absorption of chlorogenic acid and quinic acid can aid in a more comprehensive evaluation of the health-promoting effects of chlorogenic acid and eventually a better formulation and/or dosage of chlorogenic acid-containing food supplements. To our knowledge, this is the first study to investigate the in vitro intestinal absorption of quinic acid by a Caco-2 assay.
2. Materials and methods
2.1. Chemicals and reagents
Chlorogenic acid (96.63% (w/w)) was acquired from LGC standards (Teddington, UK). Ferulic acid-D3, digoxin (98% (w/w) and digoxin-D3 (97%) were acquired from Toronto Research Chemicals (Ontario, Canada). Quinic acid (98%), propranolol-D7 (100 μg mL−1 in MeOH with 5% 1 M HCl), propranolol HCl (1.0 mg mL−1 in MeOH), ammonium acetate (≥98%), Hank's balanced salt solution (HBSS) and MEM (Eagle's minimum essential medium) non-essential amino acid solution (100×) were obtained from Sigma-Aldrich (St Louis, MO, USA). DMEM (Dulbecco's Modified Eagle Medium; high glucose, no glutamine, no phenol red), heat-inactivated foetal calf serum (FCS), GlutaMAX™ and Trypsin-EDTA (0.05%, with phenol red) were acquired from Gibco (Thermo Scientific, Massachusetts, US). PenStrep (10
000 U mL−1 penicillin and 10
000 μg mL−1 streptomycin mix) was obtained from Life Technologies (California, US). Caco-2 cells (HTB-37™) were obtained from ATCC (Rockville, MD, USA). Formic acid (98–100%, Suprapur) was purchased from Merck (Darmstadt, Germany). Acetonitrile (ACN) and methanol (MeOH, ≥99.9%, LC-MS grade) were obtained from Fisher Scientific (Hampton, New Hampshire, USA). Ultrapure water (Purelab flex apparat) was acquired from ELGA Veolia (UK).
2.2. Materials
Cellstar® 12-well plates, ThinCert™ Cell Culture Inserts for 12-well plates, and 75 cm2 cell culture flasks were acquired from Greiner (Vilvoorde, Belgium). The EVE™ Automatic Cell Counter was purchased from NanoEnTek (South-Korea). The centrifuge used during handling of the Caco-2 cells was acquired from Eppendorf (Type 5702, Hamburg, Germany). A Sigma 1-15PK centrifuge (sample preparation) and centrifugal filters (modified nylon membrane, 0.2 μm, 500 μL sample capacity) were obtained respectively from Sigma Laborzentrifugen GmbH (Germany) and VWR (Radnor, Pennsylvania, USA). Trans epithelial resistance (TEER) values were measured with the Voltmètre-Ohmmètre Millicell-ERS from Merck (New Jersey, US). Cells were maintained in a C150 E2 CO2 incubator from Binder and a MaxQ Mini 4450 incubator from Thermo Scientific was used during the permeability experiment.
2.3. Cultivation of Caco-2 cells on ThinCerts
Caco-2 cells were cultured in 75 cm2 cell culture flasks containing 20 mL DMEM supplemented with 10% FCS and 1× nonessential amino acids (hereafter referred to as supplemented DMEM medium) and maintained in a humidified 5% CO2 incubator at 37 °C. The medium was changed every 3 days and the cells were passaged every 5–7 days at a 1
:
4 to 1
:
6 split ratio (depending on growth) using 0.05% trypsin-EDTA for detachment.
The cultivated Caco-2 cells were seeded on the ThinCerts when 70–90% confluent, between 21 and 29 days before the bidirectional transport experiment. First, the cells were rinsed with a 0.05% trypsin solution followed by an incubation of maximal 15 min (5% CO2, 37 °C) in fresh trypsin solution to detach the cells from the cell culture flask. The reaction was stopped by the addition of 10 mL supplemented DMEM medium. The content of the flask was transferred to a Falcon tube and cells were pelleted by centrifugation (7 min, 300 rcf). The pellet was resuspended in 6.5 mL supplemented DMEM medium with PenStrep and cell density and viability were calculated with the EVE cell counter after addition of 0.2% Trypan Blue. Cell suspensions were diluted to 0.6 × 106 viable cells per mL in supplemented DMEM medium with PenStrep. ThinCerts were pre-wetted with 100 μL supplemented DMEM medium with PenStrep at least two minutes before addition of 500 μL cell suspension and basolateral chambers were filled with 1.5 mL of the same medium. After six hours incubation at 37 °C, 5% CO2, the apical medium was replaced with 500 μL fresh supplemented DMEM medium with PenStrep to remove the non-adherent cells in order to reduce the risk of multilayer formation. Apical and basolateral media were replaced every second day and between 12 and 24 h before the permeability experiment.
2.4. Tested compounds
Chlorogenic acid, as parent compound, and quinic acid, one of the most prominent intestinal biotransformation products were selected as test compounds during the permeability experiment. Preliminary experiments using an in vitro gastrointestinal model have shown that despite quinic acid already being present in the small intestine after chlorogenic acid intake, quinic acid levels significantly rise in the colon due to microbiotic biotransformation, and therefore confirming the importance of studying the colonic absorption.17 The chlorogenic acid (10 μM and 50 μM) and quinic acid (10 μM) concentrations were chosen based on the work of Hubatsch et al.25 which states that concentrations of 10 μM or less should be used when studying possible active transport to prevent transport protein saturation. The 50 μM concentration-level of chlorogenic acid was included in the experimental setup to evaluate, if active transport was involved, if the transport was concentration-dependent and/or saturated.25 Furthermore, two positive control compounds were included in the experimental setup: (i) digoxin (10 μM) was used as positive control for the active P-glycoprotein (P-gp, MDR1) efflux mechanism and (ii) propranolol (50 μM) as a positive control for high passive permeability. Used concentrations were based on the available literature.20,26 Test solutions were prepared starting from standard solutions in MeOH using HBSS as dilution medium. The total volume of organic solvent did not exceed 1% to ensure the integrity of the cell monolayer.25 Chemical structures of all compounds included in the permeability assay are shown in Fig. 1.
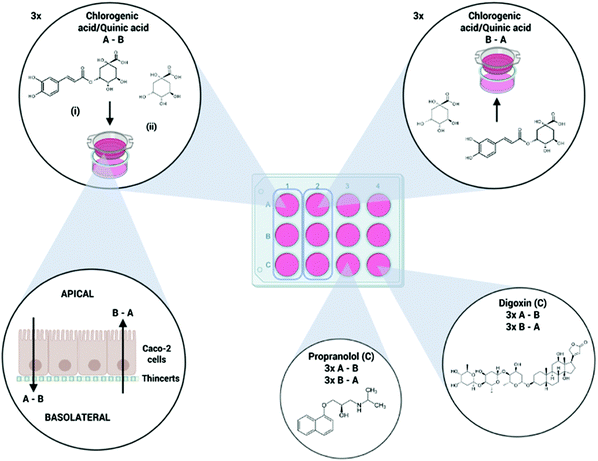 |
| Fig. 1 Overview of the experimental setup during the permeability assay with (i) chlorogenic acid and (ii) quinic acid in Caco-2 monolayers. Propranolol and digoxin were included as positive control (C) compounds. A–B: Apical to basolateral side transport. B–A: Basolateral to apical side transport. | |
2.5. Permeability experiment
The following protocol was based on the procedure described by Hubatsch et al.25 Before the experiment, the cell monolayer was washed with prewarmed HBSS to remove the residual medium. The medium was decanted from the ThinCerts and the filter supports were placed in a new 12 well plate containing fresh prewarmed HBSS (37 °C, 1.5 mL per well). 500 μL fresh HBSS was added to the apical side. The cells were incubated with gentle shaking (70 rpm) for 15 min at 37 °C in a humidified atmosphere.
The transportation experiments were carried out in both directions; from the apical to basolateral (A–B) and from basolateral to apical side (B–A), including three replicates for each compound and/or concentration level. An overview of the experimental setup is shown in Fig. 1. The preincubation medium was removed and replaced with test solutions. 400 μL prewarmed donor solutions was added to the apical side of the A–B ThinCerts, while the basolateral compartment contained 1200 μL prewarmed HBSS containing the same percentage of methanol as the donor solutions to prevent precipitation during the experiment. For the B–A wells, 1200 μL donor solution was added to the basolateral side while 400 μL HBSS with an equal methanol-percentage was added to the apical compartment. Well plates were incubated under gentle shaking (70 rpm), to minimize the effect of an unstirred water layer, at 37 °C in a humidified atmosphere. Samples, 600 μL for the A–B experiment and 200 μL for B–A experiment, were taken after 30, 60, 90 and 120 min from the receiving compartment and replaced with the same volume of fresh HBSS medium containing the same percentage of methanol as the donor solutions. Samples were immediately stored at −80 °C until analysis. Cell monolayer integrity of all wells was confirmed before and after the permeability experiment using the transepithelial electrical resistance (TEER) values.
2.6. LC-MS/MS analysis
2.6.1. Sample preparation.
20 μL internal standard solution (1 μg mL−1; see ESI†) was added to 200 μL sample aliquots followed by addition of 180 μL MeOH. Samples were vortexed for 30 s, transferred to a 0.2 μm nylon centrifugal filter and centrifuged for 5 min at 8000 rpm. The filtrates were transferred to an LC vial before analysis with liquid chromatography coupled to triple quadrupole mass spectrometry (LC-MS/MS).
2.6.2. Analysis.
Samples were analyzed using an Agilent 1290 Infinity II liquid chromatography instrument coupled to an Agilent 6495 triple quadrupole mass spectrometer (Agilent Technologies) with electrospray ionization (ESI) source. Chromatographic separation was performed on a Luna Omega PS C18 column (100 × 2.1 mm; 3 μm) from Phenomenex (Utrecht, the Netherlands). A multiple reaction monitoring (MRM) method was applied for all analytes. Overview of the ionization mode and MRM transitions of the analytes are shown in Table 1. Details on mobile phase composition, gradient elution and source parameters for the different analytes are described in ESI.†
Table 1 Overview of the used internal standard (IS), polarity and MRM transitions for the compounds included in the in vitro permeability assay (CE = collision energy). MRM transitions of the IS are mentioned between brackets
Compound
|
IS |
Polarity |
Precursor ion (m/z) |
Product ions (m/z) |
CE (V) |
QQuantifier, qqualifier. |
Chlorogenic acid |
Ferulic acid-D3 |
Negative |
353.1 |
191.0Q; 85.0q |
17; 52 |
(196.0) |
(178.0q; 151.9q; 133.9Q) |
(10; 10; 15) |
Quinic acid |
Ferulic acid-D3 |
Negative |
191.0 |
93.0q; 85.0Q; 43.2q |
25; 25; 40 |
(196.0) |
(178.0q; 151.9q; 133.9Q) |
(10; 10; 15) |
Propranolol |
Propranolol-D7 |
Positive |
260.1 |
183.0q; 116.1Q; 56.2q |
20; 20; 35 |
(267.2) |
(116.1q; 72.1q; 56.1Q) |
(20; 25;35) |
Digoxin |
Digoxin-D3 |
Positive |
798.3 |
651.2q; 112.9q; 96.8Q |
10; 40; 33 |
(801.3) |
(654.3q; 96.8Q) |
(10; 30) |
2.7.
P
app and efflux ratio calculations
Apparent permeability coefficient (Papp, cm s−1) was calculated using eqn (1). A is the area of the used ThinCerts (11.31 cm2), C0 is the dosing concentration of the tested compound expressed as μmol mL−1 and dQ/dt is the steady-state-flux (μmol s−1) which is the slope of the regression curve when plotting the cumulative amount of detected compound (mol) over time (s). The Papp (A–B) values of the tested compounds were divided by the Papp (A–B) value of propranolol obtained in the same permeability assay. The following Papp(A–B)target/Papp(A–B)PROP ratios relate to the fraction test compound absorbed, as propranolol is almost completely absorbed in vivo.20
Formula used to calculate Papp
| 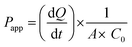 | (1) |
The efflux ratio was calculated by dividing the Papp of the B–A direction by the Papp of the A–B direction (eqn (2)). An efflux ratio greater or equal to two indicates the involvement of an active efflux transport mechanism.25
Efflux ratio formula
| 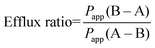 | (2) |
2.8. Validation of analytical methods
Validation of the LC-MS/MS methods was carried out over three days based on the guidelines provided by the European Medicines Agency.27 Performance parameters such as intra-day and inter-day precision and accuracy, linearity, calibration range, selectivity and carry-over effects were evaluated.
A nine-point multi-component calibration curve, with a concentration range from 5 ng mL−1 to 1000 ng mL−1, was prepared in HBSS. A broad calibration range was chosen since the expected concentrations of the assay-samples were unknown. The linearity-range was evaluated for each compound. Calibration curves were 1/x or 1/x2 weighted. The concentration of the lowest calibration curve was referred to as the lowest limit of quantification (LLOQ). The area of the LLOQ sample should be at least five times higher than the signal of the blank. Accuracy of all calibration levels had to be within 20% of the nominal value.
Four concentration-levels of quality control (QC) samples were prepared in HBSS following the sample preparation procedure described in section 2.6: LLOQ (5 ng mL−1), low QC (15 ng mL−1), mid QC (400 ng mL−1) and high QC (900 ng mL−1).
Carry-over effects were evaluated by injection of a solvent blank (HBSS without addition of standards or internal standards) after the highest calibration point in the worklist. The peak area of the blank sample should not exceed 20% of the peak area of the LLOQ sample and 5% of the internal standard peak. Selectivity was evaluated by injection of a solvent blank (HBSS, without addition of standards or internal standard). Peak areas in the solvent blank should not be more than 20% of the peak area at LLOQ level and <5% of the internal standard peak area.
The within- and between-day accuracy and precision were evaluated by analysis of 5 replicates of the 4 QC-levels over a 3-day period. Accuracy was evaluated as the deviation from the nominal spiked value (% bias). Precision was calculated as the residual standard deviation (RSD) of the mean quantified concentration. Acceptance criteria for both precision and accuracy were set at 20% for the LLOQ-level and 15% for low, mid and high QC-levels.
3. Results and discussion
3.1. Validation of analytical methods
A linear calibration curve was obtained for all compounds with a concentration range from 5 to 1000 ng mL−1 (Table 2, Fig. S1†). Table 2 summarizes the intra-day and inter-day accuracy and precision results. The results at LLOQ level were within the range of <20% bias and <20% RSD respectively for all compounds. Chlorogenic acid, quinic acid and propranolol met the criteria of <15% bias and <15% RSD respectively at the QC low, QC mid and QC high levels. Digoxin did not meet the accuracy criteria at the QC high level, with an intra- and inter-day accuracy of 20% and 18% respectively. However, concentrations of all digoxin permeability assay samples were below 200 ng mL−1, which made the method suitable for analysis of the permeability samples.
Table 2 Overview of the method validation data for all compounds: linear range, R2, intra- and interday accuracy and precision. Lower limit of quantification, LLOQ; quality control low, QCL; quality control mid, QCM and quality control high, QCH
Compound |
Linear range (ng mL−1) |
R
2
|
Accuracy (% bias) |
Precision (% RSD) |
Intra-day (n = 5) |
Inter-day (n = 15) |
Intra-day (n = 5) |
Inter-day (n = 15) |
LLOQ |
QCL |
QCM |
QCH |
LLOQ |
QCL |
QCM |
QCH |
LLOQ |
QCL |
QCM |
QCH |
LLOQ |
QCL |
QCM |
QCH |
Chlorogenic acid |
5–1000 |
0.995 |
12.2 |
14.5 |
7.8 |
12.4 |
12.2 |
14.5 |
7.0 |
12.4 |
4.6 |
3.0 |
4.8 |
3.9 |
5.3 |
4.8 |
7.2 |
4.7 |
Quinic acid |
5–1000 |
0.992 |
4.4 |
9.3 |
9.0 |
6.9 |
5.4 |
9.0 |
8.3 |
5.6 |
9.8 |
7.6 |
4.9 |
2.7 |
9.8 |
8.0 |
7.8 |
6.5 |
Propranolol |
5–1000 |
0.995 |
5.2 |
2.5 |
7.5 |
4.3 |
5.2 |
2.5 |
7.5 |
3.8 |
4.0 |
4.2 |
6.6 |
4.7 |
4.8 |
4.9 |
6.7 |
6.3 |
Digoxin |
5–1000 |
0.991 |
7.3 |
7.9 |
2.8 |
19.8 |
7.3 |
4.4 |
2.8 |
17.7 |
7.8 |
7.4 |
5.9 |
6.2 |
8.3 |
9.7 |
6.1 |
7.5 |
All compounds met the required carry-over EMA guidelines criteria as peak areas of blanks analyzed after the highest calibration level did not exceed 20% of the LLOQ standard peak area and 5% of the internal standard. Furthermore, the chromatographic methods of all compounds were in compliance with the EMA selectivity guidelines as no peaks with a peak area above 20% of the LLOQ and 5% of the peak area of the internal standard were detected in the blank samples.
3.2. Chlorogenic acid permeability experiment
3.2.1. Positive control compounds.
Positive control compounds were included in the experimental setup as between-experiments variations in Papp values could be observed using a Caco-2 cell experimental setup. These variations could result from differences in cell characteristics (age, passage number, etc.), cell culture conditions and/or varying experimental conditions.19,28–30 As suggested by Stockdale et al., the acquired Papp values of the tested compounds should be compared with the permeability values of the positive control compounds included in the same permeability assay. This methodology was also previously used by Obringer et al.,20 who divided the Papp (A–B) of the tested compounds by the measured Papp (A–B) of propranolol in the same assay. As propranolol is completely absorbed in vivo, the absorption rate of compounds with a ratio greater than 1 was equal to 100%. In this study, propranolol and digoxin were included as reference compounds for a high passive permeability and P-gp mediated efflux, respectively.20,29
Permeability coefficients of 5.45 × 10−6 cm s−1 (A–B) and 8.40 × 10−6 cm s−1 (B–A) were calculated for propranolol. These values can be used as reference values for high passive permeability as propranolol is almost completely absorbed in vivo.28 As depicted in Fig. 2 and Table S1,† no clear differences were observed for propranolol permeability in the A–B and B–A direction. This led to an efflux ratio of 1.5, which does not indicate the involvement of an active transport mechanism. This is in agreement with the findings of Zheng et al.26
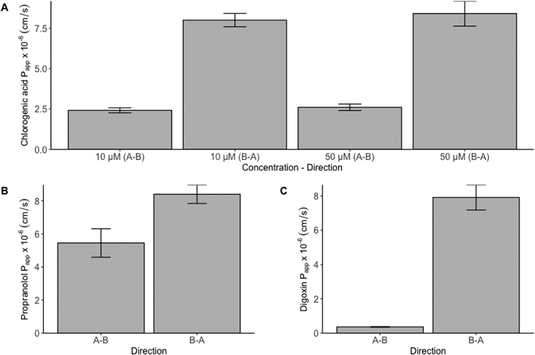 |
| Fig. 2 Overview of the Papp values, both in the apical to basolateral side (A–B) and basolateral to apical side direction (B–A), of chlorogenic acid (A), propranolol (B) and digoxin (C) obtained after the permeability experiment evaluating chlorogenic acid. | |
A clear difference in permeability between the A–B and B–A direction was observed for digoxin. In the A–B direction, a Papp of 0.36 × 10−6 cm s−1 was calculated, which is a factor 15 lower in comparison to propranolol, while the Papp in B–A direction was 7.91 × 10−6 cm s−1. As digoxin is known to be a substrate of the active efflux P-gp protein, a higher permeability coefficient from the basolateral to apical side was expected. Accordingly, the calculated efflux ratio of digoxin was 22. The results obtained for both propranolol and digoxin confirm the reliability of the assay.
3.2.2. Chlorogenic acid.
P
app (A–B) values of 2.42 × 10−6 cm s−1 and 2.61 × 10−6 cm s−1 were calculated for the 10 μM and 50 μM concentrations respectively, with corresponding Papp(A–B)CHL/Papp(A–B)PROP ratios of 44% and 48% which suggests a moderate intestinal absorption of chlorogenic acid. However, the data clearly show a higher apparent permeability coefficient in the basolateral to apical direction in comparison to the A–B direction (Fig. 2A, Table S1†). In the B–A direction, permeability coefficients of 8.01 × 10−6 cm s−1 (10 μM) and 8.41 × 10−6 cm s−1 (50 μM) were measured for chlorogenic acid which results in respective efflux ratios of 3.3 and 3.2 (Table S1†). The results, consistent for both applied concentrations, suggest the involvement of active transport from the basolateral to apical side, leading to an overall poor absorption of chlorogenic acid. However, the effect is less pronounced than for the positive control digoxin. Furthermore, no effect of the applied concentrations on the bidirectional permeability was observed which shows that the active transport proteins involved in the transport of chlorogenic acid was (were) not saturated.
The reported involvement of an active efflux in the absorption mechanism of chlorogenic acid in this study is in agreement with the findings of Erk et al.31 who reported an active efflux for chlorogenic acid using a pig jejunal mucosa in an Ussing chamber model. A 3.8-fold higher transport rate from basolateral to apical side was reported which corresponds to the presented efflux ratios in this work. Erk et al. proposed P-gp as the responsible protein for the active efflux as the secretion of chlorogenic acid was affected by the P-gp inhibitor sodiumazide (NaN3).31 Dupas et al. and Monente et al. studied the in vitro absorption of chlorogenic acid in Caco-2 cells in the apical to basolateral direction and reported a 0.14 and 0.3% recovery of the initial quantity respectively.9,32 The basolateral to apical transport was neglected in these studies and the involvement of an active efflux mechanism as observed in our experiments was not studied. Konishi et al. and Farrell et al. investigated the bidirectional in vitro transepithelial transport of chlorogenic acid using Caco-2 cells and did not report a higher permeation in the B–A direction. However, Konishi et al. used a concentration of 5 mM which is a factor 500 above the threshold of 10 μM described by Hubatsch et al. A saturation of the active transport proteins could occur and explain the deviating results.2,25,33
3.3. Quinic acid permeability experiment
3.3.1. Positive control compounds.
An overview of the positive control results can be found in Table S2† and Fig. 3. For propranolol, permeability values of 5.14 × 10−6 cm s−1 (A–B) and 5.22 × 10−6 cm s−1 (B–A) were obtained. The calculated Papp values of digoxin in A–B and B–A direction were 0.7 × 10−6 cm s−1 and 6.17 × 10−6 cm s−1 respectively, leading to an efflux ratio of 9.01. As mentioned in section 3.2.1, variations in permeability values could be observed between different experiments. Digoxin showed a lower efflux ratio in comparison to the chlorogenic acid experiment. This shows the importance of including positive control compounds in each experiment to ensure correct interpretation of the acquired results.
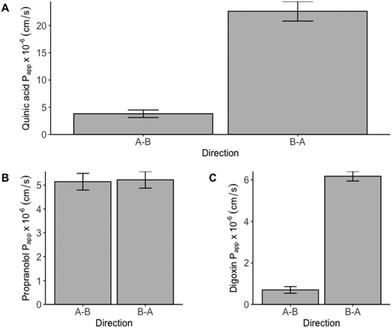 |
| Fig. 3 Overview of the Papp values, both in the apical to basolateral side (A–B) and basolateral to apical side direction (B–A), of quinic acid (A), propranolol (B) and digoxin (C) obtained after the permeability experiment evaluating quinic acid. | |
3.3.2. Quinic acid.
The mean Papp values of quinic acid are summarized in Table S2.† Quinic acid presented a Papp(A–B) of 3.8 × 10−6 cm s−1 with a corresponding Papp(A–B)QA/Papp(A–B)PROP ratio of 74%. An apparent permeability coefficient of 22.6 × 10−6 cm s−1 was detected for the basolateral to apical-direction, resulting in an efflux ratio of 5.9 (Fig. 3A, Table S2†). Given the obtained efflux ratio for quinic acid is above the cut-off value of two and the comparison with digoxin (efflux ratio of 9.01), the involvement of an active efflux mechanism for quinic acid is demonstrated. Although quinic acid demonstrated a high permeability (74% of the propranolol permeability coefficient), the overall intestinal absorption will be moderately low due to the involved active efflux mechanism.
High concentrations of quinic acid in human plasma and urine following coffee consumption have been described.34 However, no statements on the absorption of quinic acid can be made based on these data as (i) in vivo studies lead to a representation of the bioavailability of a certain compound including all ADME aspects and (ii) coffee contains multiple quinic acid containing hydroxycinnamic esters and free quinic acid. Thus, high concentrations of quinic acid in the plasma and/or urine are not necessarily linked to a high absorption of the compound. To our knowledge this is the first study to investigate the in vitro gastrointestinal absorption of quinic acid using a Caco-2 permeability assay and reporting the involvement of an active efflux transporter. The major efflux transporters located in the intestine are the ATP binding cassette (ABC)-transporters like P-gp, BCRP (breast cancer resistance protein) and MRP2 (multidrug resistance-associated protein 2).35 In order to identify the protein responsible for the active-efflux, additional experiments with suitable transport-inhibitors or Caco-2 Efflux Transporter Knockout Cells should be carried out.
In order to get a more comprehensive knowledge on the gastrointestinal fate, bioavailability and biological mechanism of chlorogenic acid (i) the concentration levels leading to saturation of the active efflux mechanism of chlorogenic acid and quinic acid should be determined in future experiments, and (ii) the in vitro intestinal absorption assay should be performed for the remaining microbiotic biotransformation products of chlorogenic acid. Farrell et al. studied the intestinal absorption of chlorogenic acid microbial biotransformation products caffeic acid and ferulic acid across a Caco-2 cell monolayer. Ferulic acid showed higher basolateral recoveries in comparison to caffeic acid suggesting methylation positively correlates with enhanced intestinal absorption. These results were confirmed by Monente et al. who reported similar results including for dihydrocaffeic acid and dihydroferulic acid. No efflux mechanisms were reported for caffeic acid and ferulic acid.33
4. Conclusions
This study investigated the intestinal absorption of chlorogenic acid and one of its most prominent intestinal biotransformation products, quinic acid, using a bidirectional Caco-2 permeability assay. The results demonstrate the presence of an active efflux transport for both chlorogenic acid and quinic acid leading to an overall low intestinal absorption of both compounds. To the best of our knowledge, this is the first study presenting the in vitro gastrointestinal absorption of quinic acid using a Caco-2 assay and reporting the involvement of an active efflux mechanism. This could be of interest for (i) studies investigating the health-promoting effects of chlorogenic acid and its biotransformation products and (ii) the optimal formulation and dosing of chlorogenic acid in food supplements in order to obtain beneficial health effects.
Author contributions
All authors provided input during the initial brainstorm sessions and contributed in preparing the manuscript. OM, JJ and IS contributed to the experimental design. Cultivation and maintenance of the Caco-2 cells was primarily done by JJ and IS together with SL. The in vitro permeability assays were performed by OM and JJ. Validation of the LC-MS/MS methods, LC-MS/MS analysis and interpretation of the data were done by OM and ALNVN. NH and SL contributed to the interpretation of the data and provided essential input to the manuscript.
Conflicts of interest
The authors declare they have no conflict of interest.
Acknowledgements
Olivier Mortelé acknowledges the Concerted Research Action (GOA) funded by the Special Fund for Research (BOF) of the University of Antwerp, 2015-2018.
References
- D. Wianowska and M. Gil, Recent advances in extraction and analysis procedures of natural chlorogenic acids, Phytochem. Rev., 2018, 18, 273–302 CrossRef
.
- Y. Konishi and S. Kobayashi, Transepithelial Transport of Chlorogenic Acid, Caffeic Acid, and Their Colonic Metabolites in Intestinal Caco-2 Cell Monolayers, J. Agric. Food Chem., 2004, 52, 2518–2526 CrossRef CAS PubMed
.
- M. D. dos Santos, M. C. Almeida, N. P. Lopes and G. de Souza, oacute, E. ria, iacute and P. lia, Evaluation of the Anti-inflammatory, Analgesic and Antipyretic Activities of the Natural Polyphenol Chlorogenic Acid, Biol. Pharm. Bull., 2006, 29, 2236–2240 CrossRef CAS PubMed
.
- G. M. Agudelo-Ochoa, I. C. Pulgarín-Zapata, C. M. Velásquez-Rodriguez, M. Duque-Ramírez, M. Naranjo-Cano, M. M. Quintero-Ortiz, O. J. Lara-Guzmán and K. Muñoz-Durango, Coffee Consumption Increases the Antioxidant Capacity of Plasma and Has No Effect on the Lipid Profile or Vascular Function in Healthy Adults in a Randomized Controlled Trial, J. Nutr., 2016, 146, 524–531 CrossRef CAS PubMed
.
- A. Hunyadi, A. Martins, T.-J. Hsieh, A. Seres and I. Zupkó, Chlorogenic Acid and Rutin Play a Major Role in the In Vivo Anti-Diabetic Activity of Morus alba Leaf Extract on Type II Diabetic Rats, PLoS One, 2012, 7, e50619 CrossRef CAS PubMed
.
- J. Santana-Galvez, L. Cisneros-Zevallos and D. A. Jacobo-Velazquez, Chlorogenic Acid: Recent Advances on Its Dual Role as a Food Additive and a Nutraceutical against Metabolic Syndrome, Molecules, 2017, 22, 358 CrossRef PubMed
.
- G. Li, D. Ma, Y. Zhang, W. Zheng and P. Wang, Coffee consumption and risk of colorectal cancer: a meta-analysis of observational studies, Public Health Nutr., 2013, 16, 346–357 CrossRef PubMed
.
- S. L. Schmit, H. S. Rennert, G. Rennert and S. B. Gruber, Coffee Consumption and the Risk of Colorectal Cancer, Cancer Epidemiol. Biomarkers Prev., 2016, 25, 634–639 CrossRef CAS PubMed
.
- C. Dupas, A. Marsset Baglieri, C. Ordonaud, D. Tome and M. N. Maillard, Chlorogenic acid is poorly absorbed, independently of the food matrix: A Caco-2 cells and rat chronic absorption study, Mol. Nutr. Food Res., 2006, 50, 1053–1060 CrossRef CAS PubMed
.
- R. W. Pero, H. Lund and T. Leanderson, Antioxidant metabolism induced by quinic acid. Increased urinary excretion of tryptophan and nicotinamide, Phytother. Res., 2009, 23, 335–346 CrossRef CAS PubMed
.
- R. W. Pero and H. Lund, Dietary quinic acid supplied as the nutritional supplement AIO + AC-11(R) leads to induction of micromolar levels of nicotinamide and tryptophan in the urine, Phytother. Res., 2011, 25, 851–857 CrossRef CAS PubMed
.
- L. Liu, Y. Liu, J. Zhao, X. Xing, C. Zhang and H. Meng, Neuroprotective Effects of D-(-)-Quinic Acid on Aluminum Chloride-Induced Dementia in Rats, J. Evidence-Based Complementary Altern. Med., 2020, 2020, 5602597–5602597 Search PubMed
.
- A. Stalmach, W. Mullen, D. Barron, K. Uchida, T. Yokota, C. Cavin, H. Steiling, G. Williamson and A. Crozier, Metabolite profiling of hydroxycinnamate derivatives in plasma and urine after the ingestion of coffee by humans: identification of biomarkers of coffee consumption, Drug Metab. Dispos., 2009, 37, 1749–1758 CrossRef CAS PubMed
.
- A. Stalmach, H. Steiling, G. Williamson and A. Crozier, Bioavailability of chlorogenic acids following acute ingestion of coffee by humans with an ileostomy, Arch. Biochem. Biophys., 2010, 501, 98–105 CrossRef CAS PubMed
.
- A. Farah, M. Monteiro, C. M. Donangelo and S. Lafay, Chlorogenic Acids from Green Coffee Extract are Highly Bioavailable in Humans, J. Nutr., 2008, 138, 2309–2315 CrossRef CAS PubMed
.
- M. P. Gonthier, M. A. Verny, C. Besson, C. Rémésy and A. Scalbert, Chlorogenic Acid Bioavailability Largely Depends on Its Metabolism by the Gut Microflora in Rats, J. Nutr., 2003, 133, 1853–1859 CrossRef CAS PubMed
.
- O. Mortelé, E. Iturrospe, A. Breynaert, E. Verdickt, B. B. Xavier, C. Lammens, S. Malhotra-Kumar, P. G. Jorens, L. Pieters, A. L. N. van Nuijs and N. Hermans, Optimization of an in vitro gut microbiome biotransformation platform with chlorogenic acid as model compound: From fecal sample to biotransformation product identification, J. Pharm. Biomed. Anal., 2019, 175, 112768 CrossRef PubMed
.
- M. P. Gonthier, C. Remesy, A. Scalbert, V. Cheynier, J. M. Souquet, K. Poutanen and A. M. Aura, Microbial metabolism of caffeic acid and its esters chlorogenic and caftaric acids by human faecal microbiota in vitro, Biomed. Pharmacother., 2006, 60, 536–540 CrossRef CAS PubMed
.
-
T. Lea, in The Impact of Food Bioactives on Health: in vitro and ex vivo models, ed. K. Verhoeckx, P. Cotter, I. Lopez-Exposito, C. Kleiveland, T. Lea, A. Mackie, T. Requena, D. Swiatecka and H. Wichers, Cham (CH), 2015, ch. 10, pp. 103–111, DOI:10.1007/978-3-319-16104-4_10
.
- C. Obringer, J. Manwaring, C. Goebel, N. J. Hewitt and H. Rothe, Suitability of the in vitro Caco-2 assay to predict the oral absorption of aromatic amine hair dyes, Toxicol. in Vitro, 2016, 32, 1–7 CrossRef CAS PubMed
.
- F. Foger, A. Kopf, B. Loretz, K. Albrecht and A. Bernkop-Schnurch, Correlation of in vitro and in vivo models for the oral absorption of peptide drugs, Amino Acids, 2008, 35, 233–241 CrossRef CAS PubMed
.
- C. Tannergren, A. Bergendal, H. Lennernäs and B. Abrahamsson, Toward an Increased Understanding of the Barriers to Colonic Drug Absorption in Humans: Implications for Early Controlled Release Candidate Assessment, Mol. Pharm., 2009, 6, 60–73 CrossRef CAS PubMed
.
- W. Rubas, M. E. M. Cromwell, Z. Shahrokh, J. Villagran, T. N. Nguyen, M. Wellton, T. H. Nguyen and R. J. Mrsny, Flux Measurements across Caco-2 Monolayers May Predict Transport in Human Large Intestinal Tissue, J. Pharm. Sci., 1996, 85, 165–169 CrossRef CAS PubMed
.
- J. Küblbeck, J. J. Hakkarainen, A. Petsalo, K.-S. Vellonen, A. Tolonen, P. Reponen, M. M. Forsberg and P. Honkakoski, Genetically Modified Caco-2 Cells With Improved Cytochrome P450 Metabolic Capacity, J. Pharm. Sci., 2016, 105, 941–949 CrossRef
.
- I. Hubatsch, E. G. Ragnarsson and P. Artursson, Determination of drug permeability and prediction of drug absorption in Caco-2 monolayers, Nat. Protoc., 2007, 2, 2111–2119 CrossRef CAS PubMed
.
- Y. Zheng, L. Z. Benet, H. Okochi and X. Chen, pH Dependent but not P-gp Dependent Bidirectional Transport Study of S-propranolol: The Importance of Passive Diffusion, Pharm. Res., 2015, 32, 2516–2526 CAS
.
-
EMA, Guideline on bioanalytical method validation, European Medicines Agency, Londen, 2011 Search PubMed
.
- M. C. Grès, B. Julian, M. Bourrié, V. Meunier, C. Roques, M. Berger, X. Boulenc, Y. Berger and G. Fabre, Correlation between oral drug absorption in humans, and apparent drug permeability in TC-7 cells, a human epithelial intestinal cell line: comparison with the parental Caco-2 cell line, Pharm. Res., 1998, 15, 726–733 CrossRef PubMed
.
- T. P. Stockdale, V. L. Challinor, R. P. Lehmann, J. J. De Voss and J. T. Blanchfield, Caco-2 Monolayer Permeability and Stability of Chamaelirium luteum (False Unicorn) Open-Chain Steroidal Saponins, ACS Omega, 2019, 4, 7658–7666 CrossRef CAS
.
- D. A. Volpe, Variability in Caco-2 and MDCK cell-based intestinal permeability assays, J. Pharm. Sci., 2008, 97, 712–725 CrossRef CAS PubMed
.
- T. Erk, J. Hauser, G. Williamson, M. Renouf, H. Steiling, F. Dionisi and E. Richling, Structure- and dose-absorption relationships of coffee polyphenols, Biofactors, 2014, 40, 103–112 CrossRef CAS PubMed
.
- C. Monente, I. A. Ludwig, A. Stalmach, M. P. de Pena, C. Cid and A. Crozier, In vitro studies on the stability in the proximal gastrointestinal tract and bioaccessibility in Caco-2 cells of chlorogenic acids from spent coffee grounds, Int. J. Food Sci. Nutr., 2015, 66, 657–664 CrossRef PubMed
.
- T. L. Farrell, L. Poquet, T. P. Dew, S. Barber and G. Williamson, Predicting phenolic acid absorption in Caco-2 cells: a theoretical permeability model and mechanistic study, Drug Metab. Dispos., 2012, 40, 397–406 CrossRef CAS PubMed
.
- T. Erk, G. Williamson, M. Renouf, C. Marmet, H. Steiling, F. Dionisi, D. Barron, R. Melcher and E. Richling, Dose-dependent absorption of chlorogenic acids in the small intestine assessed by coffee consumption in ileostomists, Mol. Nutr. Food Res., 2012, 56, 1488–1500 CrossRef CAS PubMed
.
- A. H. Schinkel and J. W. Jonker, Mammalian drug efflux transporters of the ATP binding cassette (ABC) family: an overview, Adv. Drug Delivery Rev., 2003, 55, 3–29 CrossRef CAS PubMed
.
Footnotes |
† Electronic supplementary information (ESI) available. See DOI: 10.1039/d0fo02629h |
‡ Shared last author. |
|
This journal is © The Royal Society of Chemistry 2021 |