DOI:
10.1039/D0FO02180F
(Paper)
Food Funct., 2021,
12, 119-132
Effects of Hsian-tsao (Mesona procumbens Hemsl.) extracts and its polysaccharides on the promotion of wound healing under diabetes-like conditions†
Received
18th August 2020
, Accepted 9th November 2020
First published on 23rd November 2020
Abstract
The aim of the study was to evaluate the effects of Hsian-tsao (Mesona procumbens Hemsl.) and its polysaccharides on impaired wound healing in diabetes. The results indicate that ethanol extracts of Hsian-tsao (EE) and crude polysaccharides from water extracts of Hsian-tsao (WEP) had strong inhibitory effects on methylglyoxal (MG)-induced glycation and reactive oxygen species (ROS) production. EE and WEP also decreased MG-induced inflammation-related factors in RAW 264.7 macrophages and restored MG-impaired wound-healing factors in 3T3-L1 fibroblasts. Furthermore, EE and WEP were found to dose-dependently enhance the MG-impaired phagocytosis of Staphylococcus aureus and Pseudomonas aeruginosa by macrophages. Excitingly, EE and WEP significantly enhanced wound healing on the dorsal skin through regulation of macrophage inflammatory protein-2 (MIP-2), metalloproteinase-9 (MMP-9), and tissue inhibitor of metalloproteinase-1 (TIMP-1) protein expressions in diabetic mice, as evidenced by the percentage reduction in wound surface area and the results of histopathologic scoring analysis. In conclusion, these results suggest that Hsian-tsao extract and its polysaccharides might be utilized in alternative natural therapy to promote wound healing in diabetic individuals.
Introduction
Diabetes is an important health problem worldwide. Diabetic individuals often have infectious diseases, which may accompany chronic wounds and result in a very large medical burden.1 Therefore, developing an effective therapy to improve impaired wound healing is important for the care of diabetic infections. Studies have indicated that the underlying mechanism of impaired diabetic wound healing is closely related to reactive oxygen species burst, protein glycation, and immune imbalance induced by glycative stress, such as dicarbonyls (e.g., methylglyoxal, MG), which may cause dysfunction in important cells involved in hemostasis, inflammation, proliferation, and remodeling during the wound-healing process such as fibroblasts and macrophages.2–6 Fibroblast and macrophage dysfunction can lead to chronic inflammation, impaired angiogenesis and poor tissue remodeling that deteriorate wounds. In this regard, increased inflammation-related cytokines and chemokines [e.g., interleukin-8 (IL-8), macrophage inflammatory protein-2 (MIP-2) and monocyte chemoattractant protein-1 (MCP-1)], decreased growth factors [e.g., vascular endothelial growth factor (VEGF)], and impaired homeostasis of tissue inhibitor metalloproteinases (TIMPs) and metalloproteinases (MMPs) are of great concern.7–10 In recent years, naturally occurring components have received much attention for their application in wound dressings.11 Thus, it is worth assessing the effect of natural substances on chronic wounds in diabetes, which will be an important basis for the development of alternative therapies to improve impaired diabetic wound healing.
Hsian-tsao (Mesona procumbens Hemsl.) is a common material used to prepare functional beverages in Chinese society. Several lines of evidence indicate that Hsian-tsao has strong antioxidant and anti-inflammatory properties.12–14 In this respect, phenolics (e.g., kaempferol and caffeic acid), triterpenoids (e.g., oleanolic acid and ursolic acid) and polysaccharides (e.g., Hsian-tsao leaf gum, a naturally occurring polymer) are regarded as the major functional ingredients in Hsian-tsao.11,14 Since the antioxidative and/or anti-inflammatory actions of phenolics, triterpenoids, and polysaccharides have the potential to accelerate wound healing,11,15,16 Hsian-tsao may be able to enhance wound healing in diabetic individuals. Therefore, the aim of the present study was to assess the effects of Hsian-tsao extracts and their polysaccharides on impaired diabetic wound healing. To this end, diabetes-like experimental models of MG-induced cells and streptozotocin (STZ)-induced mice were used in this study.
Materials and methods
Sample preparation
Hsian-tsao (Mesona procumbens Hemsl.) purchased from a local market in Kansai Town (Hsinchu, Taiwan) was air dried and subjected to liquid extraction with 50% ethanol solution for 24 h. The yield was further freeze dried to obtain ethanol extracts of Hsian-tsao (EE).
To extract crude polysaccharides from Hsian-tsao, air-dried Hsian-tsao was subjected to liquid extraction by water (1
:
10) at 121 °C for 20 minutes. After filtration, 95% ethanol solution was added to the yield in a proportion of 1
:
4, and then the mixture was vortexed and centrifuged at 900g for 40 minutes. The supernatant was then removed, and the precipitate was dried at 37 °C in an oven. The final dried matter obtained was crude polysaccharides from water extracts of Hsian-tsao (WEP).
Physical properties and structure analysis
The functional group and polysaccharide fouling of WEP were analyzed by Fourier transform infrared spectrometer (FTIR; PerkinElmer Spectrum one, Waltham, MA, USA), X-ray diffraction (XRD; Rigaku MiniFlex II, Japan), and thermal field emission scanning electron microscope (FE-SEM; JEOL JSM-7800F, Japan), respectively. The thermodynamic properties and the stability of WEP were analyzed used differential scanning calorimetry (DSC; PerkinElmer DSC-6000, Waltham, MA, USA) and thermogravimetric analysis (TGA; PerkinElmer TGA-4000, Waltham, MA, USA), respectively.
Cells and treatment
RAW 264.7 cells (BCRC no. 60001) and 3T3-L1 fibroblasts (BCRC no. 60159) were obtained from Bioresource Collection and Research Center (BCRC, Food Industry Research and Development Institute, Hsinchu, Taiwan), and the cells were cultured in DMEM medium (Gibco BRL, Grand Island, NY) supplemented with 10% v/v fetal bovine serum (FBS), 4.5 g L−1 glucose, 1% PS antibiotic solution (100 units mL−1 penicillin and 100 μg mL−1 streptomycin), 4 mM L-glutamine, and 1.5 g L−1 sodium bicarbonate in a 5% CO2 incubator at 37 °C.
To establish a cell model of diabetic skin ulcers, RAW 264.7 cells and 3T3-L1 fibroblasts were cultured in medium containing 25 mM glucose and 100–500 μM MG as previously reported, which is similar to the clinical glucose and MG concentrations in diabetic individuals,17,18 for 48 h. After culture treatment, the cell viability of RAW 264.7 cells and 3T3-L1 fibroblasts was tested using the MTT assay, and 100 μM or lower doses of MG that did not significantly affect cell viability were chosen for the following tests (Fig. S1†).
To determine the dose of EE and WEP samples to use in cell treatments, RAW 264.7 cells and 3T3-L1 fibroblasts were cultured in medium containing 0–200 μg mL−1 of EE or WEP for 24–72 h, and the experimental parameters (dose of EE and WEP samples and culture time) that did not significantly affect cell viability were chosen for the following tests (Fig. S2 and S3†).
Animals and treatments
The STZ-induced diabetic mouse model has already been used for studying diabetes-impaired wound healing;19 therefore, this model was used in the present study to evaluate the effect of EE and WEP on diabetes-impaired wound healing.
Eight-week-old male C57BL/6 mice obtained from the BioLASCO Experimental Animal Center (Taipei, Taiwan) were used for diabetic animal experiments. This animal test was approved by the Institutional Animal Care and Use Committee of the National Chung Hsing University, Taichung, Taiwan (IACUC Approval no: 103-115).
All mice were fed with Lab Diet 5001 and were maintained under standard laboratory conditions for one week. Thereafter, the mice were divided into a normal group (n = 20) and a diabetes mellitus (DM) group (n = 30). The mice in the diabetic group were subjected to a daily i.p. injection of streptozotocin (STZ; w/v in 0.05 M citrate buffer, pH 4.5) at a dose of 65 mg per kg b.w. for 7 consecutive days. At the same time, the mice in the normal group were subjected to an i.p. injection of citrate buffer. After the 7-day treatment, the blood glucose level of all fasted mice was measured using Accu-Chek Active (Roche, Germany), and the levels of fasting blood glucose were recorded as the reference value to confirm diabetes induction (Fig. S4†).
To establish a wound-healing animal model, the mice were anesthetized with avertin (2,2,2-tribromoethanol and 2-methyl-2-butanol), and two symmetrical wounds were induced on the dorsal skin of mice by an 8 mm disposable biopsy punch (Integra® Miltex®/33-37). Thereafter, the wounds on the mice were treated with 100 μL of saline, EE or WEP using a sprayer, and the treated wounds were covered with a 3 M Tegaderm™ Waterproof dressing to prevent infection. Detailed information on the experimental treatments for mice is provided in Table 1. The wounds were treated with samples every three days, and the change in wound size was confirmed by the recorded photos and quantified by ImageJ software (LOCI, University of Wisconsin, WI, USA). During the experimental period, the body weight and food and water intake of the mice were recorded every three days (Tables S1 to S3†). At the termination of the experiments, all mice were sacrificed, and their dorsal skins were then removed for further histopathological and immunohistochemical analyses.
Table 1 The experimental mouse treatments
Categorya |
Group (n = 10) |
Wound position on mouse skin |
Left |
Right |
Wound treatment |
EE: ethanol extracts of Hsian-tsao; WEP: crude polysaccharides from water extracts of Hsian-tsao.
|
Diabetic mice |
1 |
Saline |
Aminoguanidine (10 μg mL−1) |
2 |
EE (25 μg mL−1) |
WEP (25 μg mL−1) |
3 |
EE (50 μg mL−1) |
WEP (50 μg mL−1) |
Normal mice |
4 |
Saline |
EE (25 μg mL−1) |
5 |
Saline |
WEP (25 μg mL−1) |
Analysis of crude polysaccharides and β-1,3-glucan
Then, 0.1 g of WEP extract was added to 1 mL of NaOH (1 N) and heated at 80 °C until completely dissolved. The solution was then diluted with deionized water to 1000-fold. One milliliter of the 1000-fold dilution was added to 1 mL of phenol solution (5%) and 5 mL of concentrated sulfuric acid, vortexed evenly, and then put in an ice bath for 2 minutes. The absorbance of the polysaccharide was determined using a FLUO star galaxy spectrophotometer (BMG Labtechnologies, Offenburg, Germany) with a wavelength of 488 nm. A standard curve of glucose (0, 10, 25, 37.5 and 50 μL mL−1) was developed to quantify the polysaccharides in the samples. The concentration of WEP reported for the subsequent experiments is the concentration of the polysaccharide in WEP, not the concentration of WEP.
The β-1,3-glucan content of WEP was measured by a fluorescence method with aniline blue staining as previously reported.20,21 The fluorescence of the polysaccharide was determined using a FLUO star galaxy spectrophotometer (BMG Labtechnologies, Offenburg, Germany) with an excitation wavelength of 398 nm and an emission wavelength of 502 nm. A standard curve of curdlan (0, 5, 10, 25, 30, and 50 μL mL−1) was developed to quantify the β-1,3-glucan content in samples.
Anti-glycation assay
The antiglycation effect of the EE and WEP samples was evaluated using the MG-BSA model as previously reported.21 Briefly, 0.5 mL of EE or WEP samples (10–200 μg mL−1), aminoguanidine (AG) or phosphate buffered saline (PBS) was mixed with bovine serum albumin (BSA, 20 mg mL−1) and MG (20 mM), and the mixture was heated at 100 °C for 40 min. Thereafter, the fluorescence of an aliquot of the mixture was measured at excitation and emission wavelengths of 355 and 405 nm, respectively. The antiglycation effect as determined by the fluorescence intensity was calculated by the following formula: [fluorescence (sample)/fluorescence (control)] × 100.
Measurement of ROS
The reactive oxygen species (ROS) within cells was estimated using the 2′-7 dichlorofluorescin diacetate (DCFH-DA) method described by Wu et al.22 Briefly, RAW 264.7 cells were untreated (control) or treated with various concentrations (0–50 μg mL−1) of EE, WEP or AG under MG (10 μM) conditions for 48 h. At the end of the treatment, the cell medium was replaced with PBS containing 10 μM DCFH-DA and then maintained in the incubator for 30 minutes. Thereafter, the cells were washed twice with PBS to remove excess dye, and then 1% Triton X-100 was added to lyse the cells. Subsequently, the cell lysates were subjected to fluorescence analysis using a FLUO star galaxy spectrophotometer (BMG Labtechnologies, Offenburg, Germany) with an excitation wavelength of 485 nm and an emission wavelength of 520 nm. The ROS within cells administered different treatments was quantified by the fluorescence intensity as calculated by the following formula: [fluorescence (sample)/fluorescence (control)] × 100.
Analysis of inflammation-related factors
The inflammation-related factors of RAW 264.7 cells, including IL-8, macrophage MIP-2 and MCP-1, were quantified by western blot analysis as described by Lin et al.23 Briefly, cells were untreated (control) or treated with various concentrations (0–25 μg mL−1) of EE, WEP or AG under MG (100 μM) conditions for 48 h. At the end of the treatment, the proteins were extracted from cells and subjected to western blot analysis. Anti-IL-8 antibody (Abcam; Cambridge, UK), anti-MCP-1 antibody (Novus Biologicals; Littleton, CO, USA) and anti-MIP-2 antibody (GeneTex; Irvine, CA, USA) were used in this assay. Finally, VisionWorks LS 6.3.3 (UVP, Upland, CA, USA) was used to quantify the intensities of the bands corresponding to the target proteins, and the signal intensities were then normalized to the loading controls.
Analysis of wound-healing factors
Wound-healing factors in 3T3-L1 fibroblasts, including MMP-2, MMP-9, TIMP-1, and VEGF, were quantified by western blot analysis as described by Lin et al.22 Briefly, the cells were untreated (control) or treated with various concentrations (0–25 μg mL−1) of EE, WEP or AG under MG (100 μM) conditions for 48 h. At the end of the treatment, the proteins were extracted from cells and subjected to western blot analysis. Anti-MMP-2 antibody, anti-MMP-9 antibody, anti-TIMP-1 antibody (Abcam; Cambridge, UK), and anti-VEGF antibody (Novus Biologicals; Littleton, CO, USA) were used in this assay. Finally, VisionWorks LS 6.3.3 (UVP, Upland, CA, USA) was used to quantify the intensities of bands corresponding to the target proteins, and the signal intensities were then normalized to the loading controls.
Analysis of phagocytosis of bacterial pathogens
Staphylococcus aureus and Pseudomonas aeruginosa have been demonstrated to be the most common bacteria in skin ulcers;24 therefore, we used these two bacteria to develop a cell model of macrophage-driven phagocytosis. Briefly, the cells were cultured in black 96-well plates and left untreated (control) or treated with various concentrations (0–25 μg mL−1) of EE or WEP under MG (100 μM) conditions for 48 h. Subsequently, deactivated S. aureus or P. aeruginosa (labeled with CellTracker Green purchased from Life Technologies, Eugene, Oregon, USA) was added to the culture medium (5 × 104 CFU mL−1). After 1 h of incubation, the culture medium was removed, and the cells were washed with ice-cold PBS twice. Thereafter, the fluorescence of RAW 264.7 macrophages was measured by a FLUO star galaxy spectrophotometer (BMG Labtechnologies, Offenburg, Germany) with an excitation wavelength of 492 nm and an emission wavelength of 517 nm. The ability of RAW 264.7 cells to protect against bacterial pathogens was quantified by the fluorescence intensity as calculated by the following formula: [fluorescence (sample)/fluorescence (control)] × 100.
Histopathological analysis of skin
The dorsal skin of mice was removed, fixed in formalin, and embedded in paraffin. Thereafter, the sections were prepared and stained with hematoxylin and eosin (H & E) for histopathological analysis conducted by a professional pathologist. The histopathological scores for skin wound healing were calculated using a previously reported standard.25,26
Immunohistochemical staining
Immunohistochemical (IHC) staining was performed according to the previous report.19 Briefly, paraffin-embedded wound tissue sections were treated with 3% H2O2 and proteinase K (0.5 mg mL−1) for 10 min and incubated in a blocking buffer. Then, the primary antibodies (MMP-2, MMP-9, TIMP-1) were used for the indirect detection of target proteins and incubated in diluted 3,3-diaminobenzidine tetrahydrochloride hydrate (DAB-4HCl) for color development.
Statistical analysis
The results shown in the present study are expressed as the mean ± SD or SEM. Statistical comparisons were made using one-way analysis of variance (ANOVA) followed by Duncan's multiple comparison test. P < 0.05 was considered statistically significant.
Results
Contents of polysaccharides and β-1,3-glucan in WEP
According to Table 2, the yield of crude polysaccharides from water extracts of Hsian-tsao (WEP) was approximately 2.3%. The contents of polysaccharides and β-1,3-glucan in WEP were 301.7 and 68.9 mg g−1 of extract, respectively.
Table 2 Yield of crude polysaccharides from water extracts of Hsian-tsao (WEP) and the contents of polysaccharides and β-1,3-glucan in WEP
Extractsa |
Yield (%) |
Polysaccharides (mg g−1 extract) |
β-1,3-Glucan (mg g−1 extract) |
Extracted from the grinding powder of hot-air dried Hsian-tsao. The results represent the mean ± SD of n = 3.
|
WEP |
2.3 ± 0.1 |
301.7 ± 4.8 |
68.9 ± 5.8 |
Physical properties of WEP
To determine functional group characteristics of WEP. The WEP powder was in the detection range of 4000–400 cm−1 using FTIR spectrometer and the functional groups were identified. As shown in Fig. 1A, the broadband at 3434.00 cm−1 was standing for hydroxyl group (O–H). The carbonyl (C
O) functional group was confirmed by a peak at 1626.24 cm−1. The absorptions at both 1400.59 and 1076.47 were represented of carbon–oxygen bond (C–O). In addition, the crystalline type of WEP was assessed by XRD. The result showed that no obvious absorption peaks appeared at the range of 2θ (°) from 20° to 80°, indicating the WEP had an amorphous characteristic without sharp peaks (Fig. 1B). The polysaccharide fouling and structural topography of WEP were measured by FE-SEM. As shown in Fig. 1C, rough texture of the WEP surface was observed. Besides, the thermal stability properties of WEP were evaluated by TGA and DSC analysis. The TGA profile showed that WEP decomposition in the temperature at 310 °C with a weight loss of 26.55% (Fig. 1D). Furthermore, the DSC curve showed an endothermic peak between onset temperature (To = 96.54 °C) and crystallization temperature (Tc = 165.85 °C) during the heating process. The peak temperature (Tp) of WEP crystallization was found at 118.93 °C (Fig. 1E).
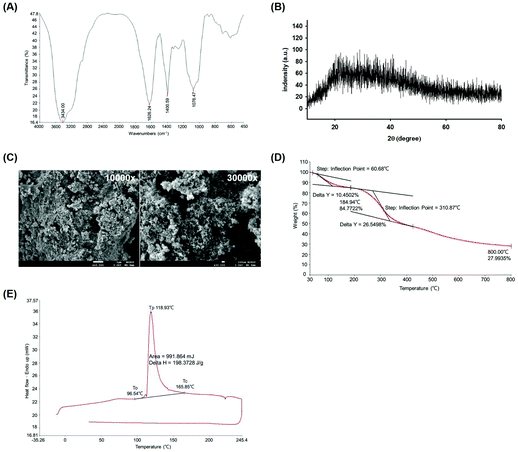 |
| Fig. 1 Physical properties and structure analysis of WEP. Charts of (A) FTIR, (B) XRD, (C) FE-SEM, (D) TGA, and (E) DSC were displayed. FE-SEM of the WEP at magnifications of 10 000× and 30 000×, respectively. WEP, crude polysaccharides from water extracts of Hsian-tsao; To, onset temperature; Tc, crystallization temperature; Tp, the peak temperature of crystallization; Delta H, crystallization enthalpy. | |
EE and WEP inhibited MG-induced protein glycation
As shown in Fig. 2A and B, 1 mg mL−1 AG, a typical anti-glycation agent, significantly inhibited MG-induced protein glycation by approximately 100% (p < 0.05). EE at different concentrations (10–200 μg mL−1) were also found to inhibit MG-induced protein glycation by approximately 50% (p < 0.05) (Fig. 2A). Similarly, 10 μg mL−1 and 25–200 μg mL−1 WEP inhibited MG-induced protein glycation by approximately 50% and 70%, respectively (p < 0.05) (Fig. 2B).
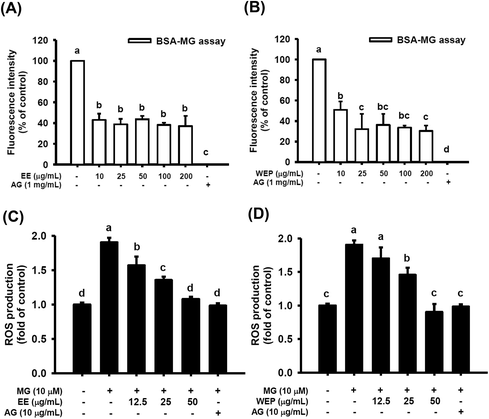 |
| Fig. 2 Effects of EE and WEP on MG-induced protein glycation (A and B) and ROS production (C and D). The results represent the mean ± SD of n = 3. Groups with different superscript letters are significantly different (p < 0.05). EE, ethanol extracts of Hsian-tsao; WEP, crude polysaccharides from water extracts of Hsian-tsao; AG, aminoguanidine. | |
EE and WEP inhibited MG-induced ROS production
Fig. 2C and D show that 10 μM MG significantly induced ROS production in RAW 264.7 cells (p < 0.05), and ROS production was significantly diminished after intervention with AG (10 μg mL−1) (p < 0.05). In addition, EE (12.5–50 μg mL−1) dose-dependently inhibited MG-induced ROS production in RAW 264.7 cells (p < 0.05) (Fig. 2C). WEP (12.5–50 μg mL−1) also inhibited MG-induced ROS production in RAW 264.7 cells (p < 0.05, excluding cells treated with 12.5 μg mL−1 WEP) (Fig. 2D).
EE and WEP decreased the expression of inflammation-related factors in MG-induced RAW 264.7 cells
IL-8, MIP-2, and MCP-1 have been demonstrated to be important modulators secreted by immune cells in inflammatory foci at wound or infection sites.27,28 As shown in Fig. 3, compared with the control condition, MG treatment (100 μM) significantly increased the protein expression of IL-8, MIP-2, and MCP-1 in RAW 264.7 cells (p < 0.05), and the expression of these factors was significantly decreased by intervention with AG (10 μg mL−1) (p < 0.05). EE and WEP (12.5–25 μg mL−1) were also found to reduce the MG-induced protein expression of IL-8, MIP-2, and MCP-1 (p < 0.05, excluding cells treated with 12.5 μg mL−1 EE in the IL-8 analysis) (Fig. 3). Compared with EE treatment, WEP treatment showed greater inhibitory effects on these MG-induced inflammation-related factors (Fig. 3).
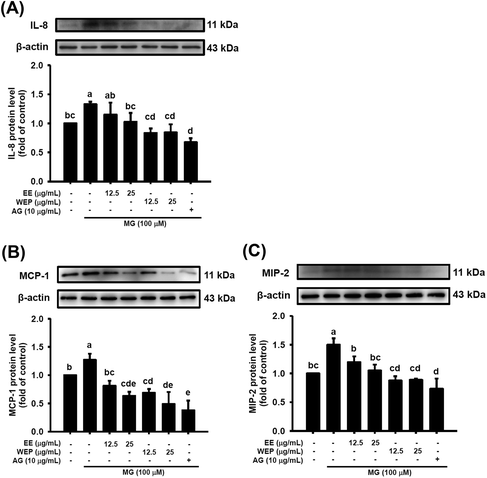 |
| Fig. 3 Effects of EE and WEP on the protein expression of (A) IL-8, (B) MCP-1, and (C) MIP-2 in MG-induced RAW 264.7 cells. The relative density of the target protein was compared with that of the control. The results represent the mean ± SD of n = 3. Groups with different superscript letters are significantly different (p < 0.05). EE, ethanol extracts of Hsian-tsao; WEP, crude polysaccharides from water extracts of Hsian-tsao; AG, aminoguanidine. | |
EE and WEP modulated the expression of wound-healing factors in MG-induced 3T3-L1 fibroblasts
The dysregulation of MMPs/TIMP-1 and VEGF can lead to the degradation of matrix proteins and impaired angiogenesis, respectively, which is regarded as the major cause of impaired wound healing in diabetic individuals. Fig. 4 shows that MG treatment (100 μM) significantly increased the protein expression of MMP-2 and MMP-9 and decreased the protein expression of TIMP-1 and VEGF in 3T3-L1 fibroblasts (p < 0.05), which was significantly abrogated after intervention with AG (10 μg mL−1) (p < 0.05). Furthermore, EE treatment significantly decreased the MG-induced protein expression of MMP-2 and MMP-9 (Fig. 4A and B) and increased the MG-impaired protein expression of TIMP-1 (Fig. 4C) in a dose-dependent manner. However, EE treatment did not restore the MG-impaired protein expression of VEGF (Fig. 4D). WEP treatment was found to dose-dependently abolish the MG-induced protein expression of MMP-2 and MMP-9 and the MG-impaired protein expression of TIMP-1 and VEGF (p < 0.05) (Fig. 4). Compared with EE treatment, WEP treatment showed increased inhibitory effects on these MG-induced and MG-impaired wound-healing factors (Fig. 4). Compared with normal controls, treatment with AG (10 μg mL−1) or WEP (25 μg mL−1) significantly decreased the protein expression of MMP-2 (p < 0.05) (Fig. 4B) and increased the protein expression of TIMP-1 and VEGF (p < 0.05) (Fig. 4C and D).
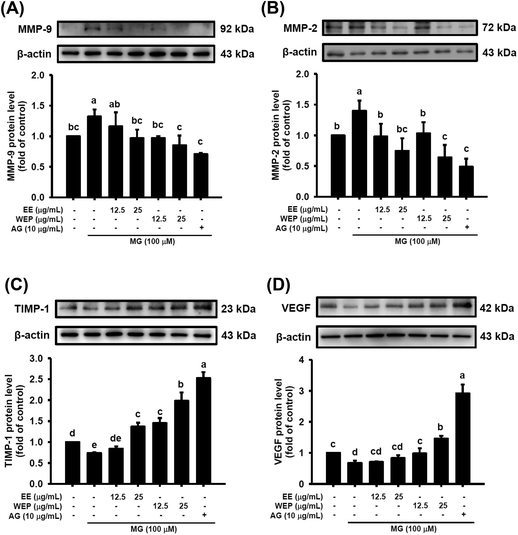 |
| Fig. 4 Effects of EE and WEP on the protein expression of (A) MMP-9, (B) MMP-2, (C) TIMP-1, and (D) VEGF in MG-induced 3 T3-L1 fibroblasts. The relative density of the target protein was compared with that of the control. The results represent the mean ± SD of n = 3. Groups with different superscript letters are significantly different (p < 0.05). EE, ethanol extracts of Hsian-tsao; WEP, crude polysaccharides from water extracts of Hsian-tsao; AG, aminoguanidine. | |
EE and WEP modulated the macrophage-driven phagocytosis of bacteria
As shown in Fig. 5, MG treatment (100 μM) did not affect the macrophage-driven phagocytosis of S. aureus and P. aeruginosa. AG treatment (10 μg mL−1) was found to enhance the phagocytosis of S. aureus in MG-treated macrophages (p < 0.05) (Fig. 5A) but to impair the phagocytosis of P. aeruginosa in MG-treated macrophages (p < 0.05) (Fig. 5B). Furthermore, EE and WEP dose-dependently enhanced the phagocytosis of S. aureus and P. aeruginosa in MG-treated macrophages (p < 0.05) (Fig. 5). Compared with EE treatment (25 μg mL−1), WEP treatment (25 μg mL−1) showed increased the phagocytosis of S. aureus in MG-treated macrophages (Fig. 5A).
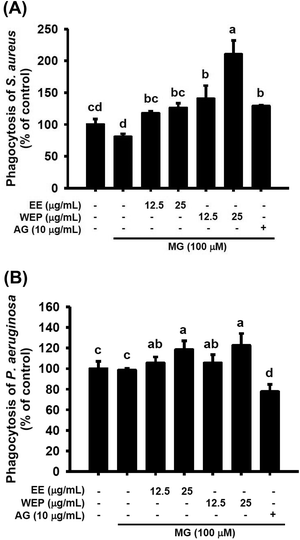 |
| Fig. 5 Effects of EE and WEP on the phagocytosis of (A) Staphylococcus aureus and (B) Pseudomonas aeruginosa by MG-induced RAW 264.7 cells. The results are expressed as the percentage of the control. Data show the mean ± SD of n = 3. Groups with different superscript letters are significantly different (p < 0.05). EE, ethanol extracts of Hsian-tsao; WEP, crude polysaccharides from water extracts of Hsian-tsao; AG, aminoguanidine. | |
EE and WEP promoted wound healing on the skin of normal and diabetic mice
Table 3 shows that the percent wound surface area of EE- or WEP-treated wounds was smaller than that of untreated wounds on the dorsal skin of normal mice 6 days post-wounding (p < 0.05). In the DM groups, the percent wound surface area of AG-treated wounds was smaller than that of untreated wounds on the dorsal skin of diabetic mice 15 days post-wounding (p < 0.05) (Table 3 and Fig. 6). The area of EE- or WEP-treated wounds was also smaller than that of untreated wounds on the dorsal skin of diabetic mice 9 days post-wounding (p < 0.05) (Table 3 and Fig. 6).
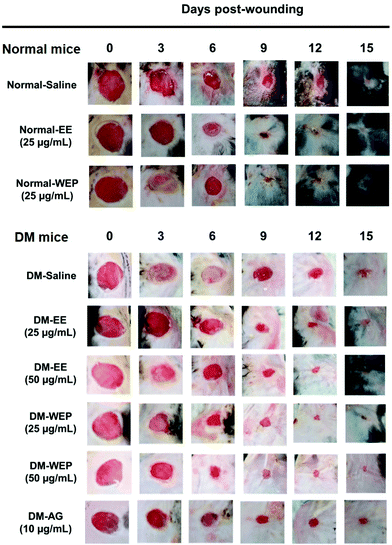 |
| Fig. 6 Effects of EE and WEP on wound healing acceleration in mice. Wounds were created on the skin of normal and diabetic mice. Wound healing effects were observed after EE and WEP treatments on post-wounding days 0, 3, 6, 9, 12, and 15, respectively. EE, ethanol extracts of Hsian-tsao; WEP, crude polysaccharides from water extracts of Hsian-tsao; AG, aminoguanidine. | |
Table 3 Wound-healing effects of EE and WEP on the skin of normal and diabetic mice
Treatment |
Days post-wounding |
0 |
3 |
6 |
9 |
12 |
15 |
Percent wound surface area |
Results represent the mean ± SEM of n = 8. Groups with different alphabetic uppercase letters (N groups) or lowercase letters (DM groups) are significantly different from one another at the same point (p < 0.05). The wound size was measured by ImageJ software. N: normal; EE: ethanol extracts of Hsian-tsao; WEP: crude polysaccharides from water extracts of Hsian-tsao; DM: diabetes; AG: aminoguanidine.
|
N-salinea |
100.0 ± 0.0A |
77.0 ± 4.7A |
44.5 ± 3.4A |
19.7 ± 1.0A |
11.2 ± 0.7A |
6.2 ± 0.8A |
N-EE (25 μg mL−1) |
100.0 ± 0.0A |
77.2 ± 2.9A |
36.3 ± 3.3B |
12.4 ± 1.0B |
7.0 ± 0.8B |
2.4 ± 0.3B |
N-WEP (25 μg mL−1) |
100.0 ± 0.0A |
65.5 ± 2.7A |
40.3 ± 1.9B |
10.5 ± 1.1B |
5.0 ± 0.9B |
1.5 ± 0.4B |
DM-saline |
100.0 ± 0.0a |
83.0 ± 2.4ab |
49.5 ± 2.6a |
31.6 ± 2.8a |
15.8 ± 1.5a |
9.4 ± 1.1a |
DM-EE (25 μg mL−1) |
100.0 ± 0.0a |
84.6 ± 4.2a |
51.1 ± 1.9a |
21.7 ± 2.1bc |
10.8 ± 0.9b |
5.7 ± 0.5b |
DM-EE (50 μg mL−1) |
100.0 ± 0.0a |
70.6 ± 3.8bc |
42.1 ± 2.4ab |
23.0 ± 1.8bc |
11.1 ± 0.4b |
3.8 ± 0.65bc |
DM-WEP (25 μg mL−1) |
100.0 ± 0.0a |
78.0 ± 3.2ab |
40.1 ± 3.5ab |
18.3 ± 2.3c |
9.2 ± 1.2bc |
4.9 ± 0.6b |
DM-WEP (50 μg mL−1) |
100.0 ± 0.0a |
75.6 ± 5.6ab |
40.3 ± 4.3ab |
18.7 ± 2.1c |
9.3 ± 1.0b |
5.6 ± 0.7b |
DM-AG (10 μg mL−1) |
100.0 ± 0.0a |
76.8 ± 2.8ab |
49.8 ± 4.7a |
26.5 ± 2.1ab |
14.7 ± 1.4a |
4.6 ± 1.1bc |
Table 4 and Fig. 7 show the results of the histopathological analysis of the EE- or WEP-treated skin wounds of normal and diabetic mice. According to the results, the degree of crust and epidermis formation, inflammation, angiogenesis, granulation and re-epithelialization of the skin tissues was not significantly different between untreated normal mice and normal mice treated with EE or WEP (Table 4). In the DM groups, the degree of crust and epidermis formation in the skin tissues was not significantly different between untreated mice and mice treated with AG. The degree of inflammation and angiogenesis of the skin tissues of AG-treated diabetic mice was significantly lower than that of untreated diabetic mice (p < 0.05). The degree of granulation and re-epithelialization of the skin tissues of AG-treated diabetic mice was significantly higher than that of untreated diabetic mice (p < 0.05) (Table 4). The degree of crust and epidermis formation of the skin tissues of diabetic mice treated with EE or WEP (50 μg mL−1) was found to be lower than that of untreated diabetic mice. In addition, the degree of inflammation and angiogenesis of the skin tissues of diabetic mice treated with EE or WEP (25 and 50 μg mL−1) was found to be lower than that of untreated diabetic mice. The degree of granulation and re-epithelialization of the skin tissues of EE- or WEP-treated diabetic mice was also found to be significantly higher than that of untreated diabetic mice (p < 0.05) (Table 4). In addition, the findings of the immunohistochemical analysis for MIP-2, MMP-9, and TIMP-1 also supported the positive effects of EE and WEP on impaired diabetic wound healing (Fig. 8–10).
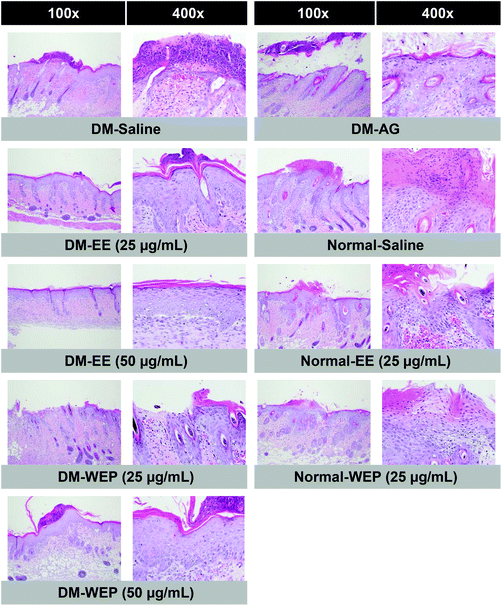 |
| Fig. 7 Histopathological findings of skin wounds from different Hsian-tsao extracts-treated diabetic or normal mice on the 15th day post-wounding. Skin wounds showed focal epidermal crust, inflammation, angiogenesis, granulation layer and re-epithelialization as evidence by H&E staining (100× and 400×). | |
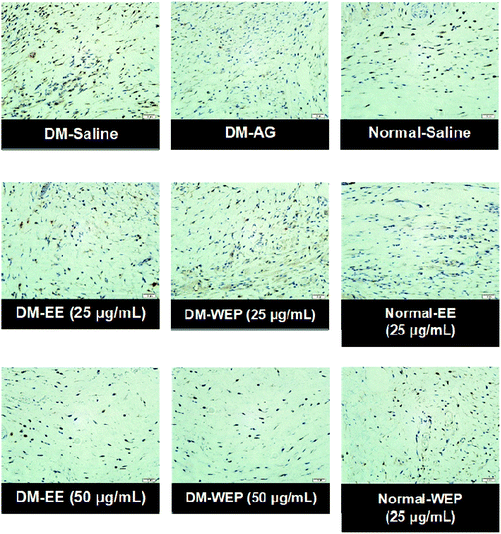 |
| Fig. 8 Representative immunohistochemical MIP-2 staining of wound sections from different Hsian-tsao extract-treated diabetic or normal mice on the 15th day post-wounding. | |
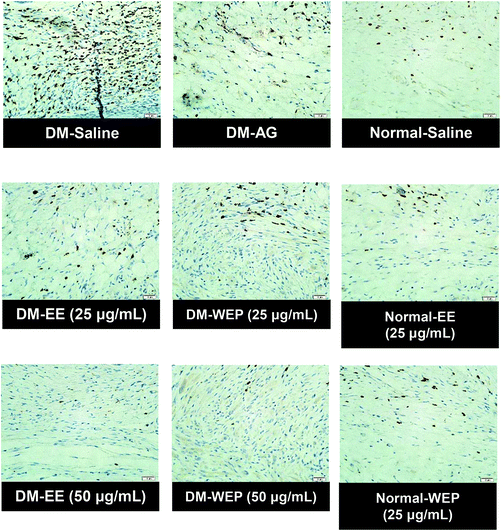 |
| Fig. 9 Representative immunohistochemical MMP-9 staining of wound sections from different Hsian-tsao extract-treated diabetic or normal mice on the 15th day post-wounding. | |
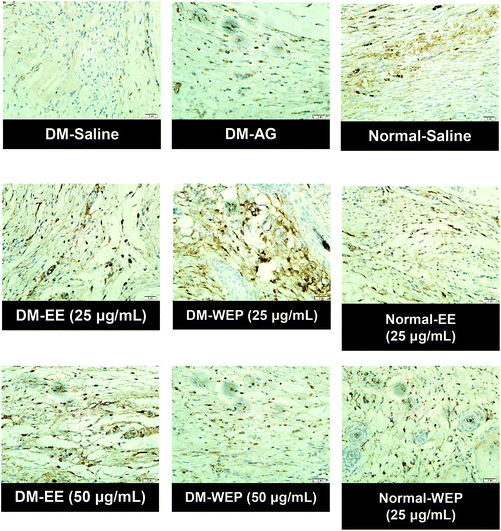 |
| Fig. 10 Representative immunohistochemical TIMP-1 staining of wound sections from different Hsian-tsao extract-treated diabetic or normal mice on the 15th day post-wounding. | |
Table 4 Histopathological scores of EE- and WEP-treated wounds on the skin of normal and diabetic mice
Treatment |
Histopathology |
Crust, epidermisb |
Inflammationb |
Angiogenesisc |
Granulationc |
Re-epithelializationc |
Results represent the mean ± SEM for n = 8. Groups with different alphabetic uppercase letters (N groups) or lowercase letters (DM groups) are significantly different from one another at the same point (p < 0.05). N: normal; EE: ethanol extracts of Hsian-tsao; WEP: crude polysaccharides from water extracts of Hsian-tsao; DM: diabetes; AG: aminoguanidine.
Degree of lesions was graded from one to five depending on severity: 1 = minimal (<1%); 2 = slight (1–25%); 3 = moderate (26–50%); 4 = moderate/severe (51–75%); 5 = severe/high (76–100%).
Degree of lesions was graded from one to four according to the method of Altavilla et al., 2001.
|
N-salinea |
0.8 ± 0.3A |
1.3 ± 0.3A |
1.5 ± 0.4A |
3.0 ± 0.3A |
2.6 ± 0.2A |
N-EE (25 μg mL−1) |
0.3 ± 0.2A |
0.5 ± 0.3A |
0.5 ± 0.3A |
3.6 ± 0.2A |
2.9 ± 0.1A |
N-WEP (25 μg mL−1) |
0.5 ± 0.3A |
0.5 ± 0.3A |
0.8 ± 0.5A |
3.8 ± 0.2A |
2.8 ± 0.2A |
DM-saline |
1.5 ± 0.6a |
3.1 ± 0.4a |
3.1 ± 0.1a |
2.1 ± 0.2a |
2.0 ± 0.3a |
DM-EE (25 μg mL−1) |
0.8 ± 0.3ab |
0.5 ± 0.4c |
0.4 ± 0.4bc |
3.9 ± 0.1bc |
3.0 ± 0.0b |
DM-EE (50 μg mL−1) |
0.0 ± 0.0b |
0.0 ± 0.0c |
0.0 ± 0.0c |
4.0 ± 0.0c |
3.0 ± 0.0b |
DM-WEP (25 μg mL−1) |
0.5 ± 0.4ab |
0.5 ± 0.3c |
0.6 ± 0.4bc |
3.8 ± 0.2bc |
2.8 ± 0.2b |
DM-WEP (50 μg mL−1) |
0.0 ± 0.0b |
0.0 ± 0.0c |
0.0 ± 0.0c |
4.0 ± 0.0c |
3.0 ± 0.0b |
DM-AG (10 μg mL−1) |
0.9 ± 0.4ab |
1.9 ± 0.5b |
1.1 ± 0.5b |
3.4 ± 0.3b |
2.8 ± 0.2b |
Discussion
Chronic wounds are a serious problem in diabetes care. Therefore, it is important to develop an effective therapy to promote wound healing in diabetic individuals. In diabetes, hyperglycemia-related glycative stress induced by dicarbonyls, especially MG, can induce the production of ROS and the formation of advanced glycation end products (AGEs), which have been demonstrated to amplify proinflammatory responses, thereby prolonging injury and impairing wound healing.2–6,29 Similar to previous findings,30 EE and WEP had marked inhibitory effects on MG-induced glycation (i.e., AGE formation) and ROS production (Fig. 2), suggesting that Hsian-tsao extracts and their polysaccharides may attenuate glycative stress-induced inflammation.
In terms of the inflammation involved in the wound-healing process, the actions of cytokines and chemokines secreted by macrophages are commonly discussed.4,7 In this regard, IL-8, MCP-1, and MIP-2 have been shown to modulate tissue lesions and post-infectious inflammation.27,28 These chemokines can remove damaged tissues and evoke inflammatory responses against pathogen infection. However, the overload of these chemokines is usually found in wounds in diabetic individuals, which may increase tissue permeability and leucocyte infiltration, thereby leading to chronic inflammation in peripheral normal skin tissue and finally delayed wound healing.4,7 In the present study, we first found that MG significantly induced the protein expression of IL-8, MCP-1, and MIP-2 in RAW264.7 macrophages (Fig. 3). This phenomenon resembled the high amounts of IL-8 and MCP-1 in the wounds of diabetic mice.31 As expected, EE and WEP showed strong inhibitory effects on the MG-induced protein expression of IL-8, MCP-1, and MIP-2 (Fig. 3); the biological actions of specific phenolics and polysaccharides within Hsian-tsao may play important roles in this process.12–14
Fibroblasts have been demonstrated to play an important role in tissue repair in the wound-healing microenvironment.6 Many studies have indicated that the wound-healing factors secreted by fibroblasts, including MMPs, TIMP-1, and VEGF, are in a state of imbalance within diabetic individuals. This imbalance significantly interferes with tissue remodeling, including angiogenesis, granulation and re-epithelialization during the wound-healing process, thereby resulting in diabetic chronic wounds.10,32,33 The present study first showed that MG significantly impaired the homeostasis of TIMP-1 and MMPs and decreased VEGF expression (Fig. 4), suggesting that MG may be a pivotal factor in the deterioration of diabetic chronic wounds. Excitingly, treatment with EE or WEP could restore the impaired homeostasis of TIMP-1 and MMPs and the lower VEGF levels in MG-induced 3T3-L1 fibroblasts, suggesting that Hsian-tsao extracts and their polysaccharides could promote tissue remodeling in diabetic wounds.
Bacterial infection is commonly found to impair wound healing in diabetes because glycative stress can damage the action of phagocytosis driven by macrophages and then increase the susceptibility of infection.34,35 In the present study, we found that MG treatment slightly impaired the macrophage-driven phagocytosis of S. aureus and P. aeruginosa (Fig. 5). Notably, treatment with EE or WEP can enhance macrophage-driven phagocytosis (Fig. 5), suggesting that Hsian-tsao has potential to inhibit bacterial infection, which may assist the improvement of impaired wound healing.
As for wound healing, hemostasis occurs first, and then, the xenobiotics in wounds are removed by inflammation. Thereafter, the crust, which is composed of dead cells and necrotic tissue, forms, and granulation and angiogenesis driven by fibroblasts then occurs under the crust; finally, the regenerated tissue is remodeled into the normal tissue.4 Our data indicated that impaired wound healing occurs in diabetic mice (Table 3), as indicated by increased crust, poor granulation, angiogenesis, and poor re-epithelialization (Table 4). Excitingly, treatment with EE or WEP was first found to promote wound healing in diabetic mice (Table 3 and Fig. 6), as evidenced by decreased crust and enhanced granulation, angiogenesis, and re-epithelialization (Table 4 and Fig. 7). In this regard, the actions of the phenolics and polysaccharides within Hsian-tsao may make a strong contribution to the overall effect.12–14
Aminoguanidine (AG), a typical antiglycation agent,22 was used as the positive control in the present study. AG was shown to restore MG-induced glycation, ROS, and cell damage (Fig. 2–4). However, AG significantly weakened the macrophage-driven phagocytosis of P. aeruginosa but not that of S. aureus (Fig. 5). This may be related to the inhibitory effect of AG on inducible nitric oxide synthase (iNOS) because iNOS can modulate the action of macrophage-driven phagocytosis.36
Conclusions
Hsian-tsao extracts and their polysaccharides display marked inhibitory effects on glycative stress-induced glycation, ROS production, and macrophage and fibroblast dysfunction. In addition, they significantly improve the slow healing of wounds in diabetes. Therefore, Hsian-tsao intervention can be considered an alternative therapy for chronic diabetic wounds.
Abbreviations
AGEs | Advanced glycation end products |
ROS | Reactive oxygen species |
EE | Ethanol extracts of Hsian-tsao |
WEP | Crude polysaccharides from water extracts of Hsian-tsao |
MG | Methylglyoxal |
AG | Aminoguanidine |
IL-8 | Interleukin-8 |
MIP-2 | Macrophage inflammatory protein-2 |
MCP-1 | Monocyte chemoattractant protein-1 |
MMP | Metalloproteinase |
TIMP-1 | Tissue inhibitor of metalloproteinase-1 |
VEGF | Vascular endothelial growth factor |
STZ | Streptozotocin |
BSA | Bovine serum albumin |
FBS | Fetal bovine serum |
H & E | Hematoxylin and eosin |
ANOVA | Analysis of variance |
Conflicts of interest
The authors declare no conflicts of interest regarding the publication of this article.
Acknowledgements
This research work was supported by the part by the grants 105AS-3.2.2-FD-Z1(1) and 106AS-3.2.2-FD-Z1(1) from Council of Agriculture, Taiwan.
References
- S. K. Nethi, S. Das, C. R. Patra and S. Mukherjee, Recent advances in inorganic nanomaterials for wound-healing applications, Biomater. Sci., 2019, 7, 2652–2674 RSC.
- J. Majtan, Methylglyoxal-a potential risk factor of manuka honey in healing of diabetic ulcers, J. Evidence-Based Complementary Altern. Med., 2011, 2011, 295494 Search PubMed.
- H. Li, M. O'Meara, X. Zhang, K. Zhang, B. Seyoum, Z. Yi, R. J. Kaufman, T. J. Monks and J. M. Wang, Ameliorating methylglyoxal-induced progenitor cell dysfunction for tissue repair in diabetes, Diabetes, 2019, 68, 1287–1302 CrossRef CAS.
- S. Guo and L. A. Dipietro, Factors affecting wound healing, J. Dent. Res., 2010, 89, 219–229 CrossRef CAS.
- E. Gianino, C. Miller and J. Gilmore, Smart wound dressings for diabetic chronic wounds, Bioengineering, 2018, 5, 51 CrossRef CAS.
- P. Bainbridge, Wound healing and the role of fibroblasts, J. Wound Care, 2013, 22, 407–408 CrossRef CAS.
- A. Ridiandries, J. T. M. Tan and C. A. Bursill, The role of chemokines in wound healing, Int. J. Mol. Sci., 2018, 19, 3217 CrossRef.
- U. A. Okonkwo and L. A. DiPietro, Diabetes and wound angiogenesis, Int. J. Mol. Sci., 2017, 18, 1419 CrossRef.
- O. Ochoa, F. M. Torres and P. K. Shireman, Chemokines and diabetic wound healing, Vascular, 2007, 15, 350–355 CrossRef.
- R. Gary Sibbald and K. Y. Woo, The biology of chronic foot ulcers in persons with diabetes, Diabetes Metab. Res. Rev., 2008, 24(Suppl 1), S25–S30 CrossRef.
- M. Naseri-Nosar and Z. M. Ziora, Wound dressings from naturally-occurring polymers: a review on homopolysaccharide-based composites, Carbohydr. Polym., 2018, 189, 379–398 CrossRef CAS.
- G. C. Yen and C. Y. Hung, Effects of alkaline and heat treatment on antioxidative activity and total phenolics of extracts from Hsian-tsao (Mesona procumbens Hemsl.), Food Res. Int., 2000, 33, 487–492 CrossRef CAS.
- L. S. Lai, S. T. Chou and W. W. Chao, Studies on the antioxidative activities of Hsian-tsao (Mesona procumbens Hemsl) leaf gum, J. Agric. Food Chem., 2001, 49, 963–968 CrossRef CAS.
- H. C. Huang, S. H. Chuang, Y. C. Wu and P. M. Chao, Hypolipidaemic function of Hsian-tsao tea (Mesona procumbens Hemsl.): working mechanisms and active components, J. Funct. Foods, 2016, 26, 217–227 CrossRef CAS.
- Y. Song, R. Zeng, L. Hu, K. G. Maffucci, X. Ren and Y. Qu,
In vivo wound healing and in vitro antioxidant activities of Bletilla striata phenolic extracts, Biomed. Pharmacother., 2017, 93, 451–461 CrossRef CAS.
- L. C. Agra, J. N. Ferro, F. T. Barbosa and E. Barreto, Triterpenes with healing activity: a systematic review, J. Dermatol. Treat., 2015, 26, 465–470 CrossRef.
- A. Lapolla, R. Reitano, R. Seraglia, G. Sartore, E. Ragazzi and P. Traldi, Evaluation of advanced glycation end products and carbonyl compounds in patients with different conditions of oxidative stress, Mol. Nutr. Food Res., 2005, 49, 685–690 CrossRef CAS.
- A. Lapolla, R. Flamini, A. Dalla Vedova, A. Senesi, R. Reitano, D. Fedele, E. Basso, R. Seraglia and P. Traldi, Glyoxal and methylglyoxal levels in diabetic patients: quantitative determination by a new GC/MS method, Clin. Chem. Lab. Med., 2003, 41, 1166–1173 CAS.
- C. H. Wu, A. Z. Chen and G. C. Yen, Protective effects of glycyrrhizic acid and 18β-glycyrrhetinic acid against cisplatin-induced nephrotoxicity in BALB/c mice, J. Agric. Food Chem., 2015, 63, 1200–1209 CrossRef CAS.
- S. H. Young, W. J. Dong and R. R. Jacobs, Observation of a partially opened triple-helix conformation in 1–>3-beta-glucan by fluorescence resonance energy transfer spectroscopy, J. Biol. Chem., 2000, 275, 11874–11879 CrossRef CAS.
- Y. T. Ko and Y. L. Lin, 1,3-beta-glucan quantification by a fluorescence microassay and analysis of its distribution in foods, J. Agric. Food Chem., 2004, 52, 3313–3318 CrossRef CAS.
- C. H. Wu, H. W. Huang, J. A. Lin, S. M. Huang and G. C. Yen, The proglycation effect of caffeic acid leads to the elevation of oxidative stress and inflammation in monocytes, macrophages and vascular endothelial cells, J. Nutr. Biochem., 2011, 22, 585–594 CrossRef CAS.
- J. A. Lin, C. H. Wu and G. C. Yen, Methylglyoxal displays colorectal cancer-promoting properties in the murine models of azoxymethane and CT26 isografts, Free Radicals Biol. Med., 2018, 115, 436–446 CrossRef CAS.
- L. J. Bessa, P. Fazii, M. Di Giulio and L. Cellini, Bacterial isolates from infected wounds and their antibiotic susceptibility pattern: some remarks about wound infection, Int. Wound J., 2015, 12, 47–52 CrossRef.
- D. Altavilla, A. Saitta, D. Cucinotta, M. Galeano, B. Deodato, M. Colonna, V. Torre, G. Russo, A. Sardella, G. Urna, G. M. Campo, V. Cavallari, G. Squadrito and F. Squadrito, Inhibition of lipid peroxidation restores impaired vascular endothelial growth factor expression and stimulates wound healing and angiogenesis in the genetically diabetic mouse, Diabetes, 2001, 50, 667–674 CrossRef CAS.
- D. Altavilla, M. Galeano, A. Bitto, L. Minutoli, G. Squadrito, P. Seminara, F. S. Venuti, V. Torre, M. Calo, M. Colonna, P. Lo Cascio, G. Giugliano, N. Scuderi, C. Mioni, S. Leone and F. Squadrito, Lipid peroxidation inhibition by raxofelast improves angiogenesis and wound healing in experimental burn wounds, Shock, 2005, 24, 85–91 CrossRef CAS.
- J. E. Park and A. Barbul, Understanding the role of immune regulation in wound healing, Am. J. Surg., 2004, 187, 11S–16S CrossRef CAS.
- K. Ley, C. Laudanna, M. I. Cybulsky and S. Nourshargh, Getting to the site of inflammation: the leukocyte adhesion cascade updated, Nat. Rev. Immunol., 2007, 7, 678–689 CrossRef CAS.
- Y. Guo, C. Lin, P. Xu, S. Wu, X. Fu, W. Xia and M. Yao, AGEs induced autophagy impairs cutaneous wound healing via stimulating macrophage polarization to M1 in diabetes, Sci. Rep., 2016, 6, 36416 CrossRef CAS.
- S. Adisakwattana, T. Thilavech and C. Chusak,
Mesona Chinensis Benth extract prevents AGE formation and protein oxidation against fructose-induced protein glycation in vitro, BMC Complementary Altern. Med., 2014, 14, 130 CrossRef.
- E. C. Leal, E. Carvalho, A. Tellechea, A. Kafanas, F. Tecilazich, C. Kearney, S. Kuchibhotla, M. E. Auster, E. Kokkotou, D. J. Mooney, F. W. LoGerfo, L. Pradhan-Nabzdyk and A. Veves, Substance P promotes wound healing in diabetes by modulating inflammation and macrophage phenotype, Am. J. Pathol., 2015, 185, 1638–1648 CrossRef CAS.
- Z. Zeng, W. D. Huang, Q. Gao, M. L. Su, Y. F. Yang, Z. C. Liu and B. H. Zhu, Arnebin-1 promotes angiogenesis by inducing eNOS, VEGF and HIF-1alpha expression through the PI3K-dependent pathway, Int. J. Mol. Med., 2015, 36, 685–697 CrossRef CAS.
- R. Lobmann, C. Zemlin, M. Motzkau, K. Reschke and H. Lehnert, Expression of matrix metalloproteinases and growth factors in diabetic foot wounds treated with a protease absorbent dressing, J. Diabetes Complications, 2006, 20, 329–335 CrossRef.
- J. G. Kiselar, X. Wang, G. R. Dubyak, C. El Sanadi, S. K. Ghosh, K. Lundberg and W. M. Williams, Modification of beta-defensin-2
by dicarbonyls methylglyoxal and glyoxal inhibits antibacterial and chemotactic function in vitro, PLoS One, 2015, 10, e0130533 CrossRef.
- S. Khanna, S. Biswas, Y. Shang, E. Collard, A. Azad, C. Kauh, V. Bhasker, G. M. Gordillo, C. K. Sen and S. Roy, Macrophage dysfunction impairs resolution of inflammation in the wounds of diabetic mice, PLoS One, 2010, 5, e9539 CrossRef.
- T. P. Misko, W. M. Moore, T. P. Kasten, G. A. Nickols, J. A. Corbett, R. G. Tilton, M. L. McDaniel, J. R. Williamson and M. G. Currie, Selective inhibition of the inducible nitric oxide synthase by aminoguanidine, Eur. J. Pharmacol., 1993, 233, 119–125 CrossRef CAS.
Footnotes |
† Electronic supplementary information (ESI) available. See DOI: 10.1039/d0fo02180f |
‡ These authors contributed equally to this work. |
|
This journal is © The Royal Society of Chemistry 2021 |
Click here to see how this site uses Cookies. View our privacy policy here.