DOI:
10.1039/D0FO01459A
(Paper)
Food Funct., 2021,
12, 278-290
Improvement of the hepatic lipid status in intrauterine growth retarded pigs by resveratrol is related to the inhibition of mitochondrial dysfunction, oxidative stress and inflammation†
Received
4th June 2020
, Accepted 23rd November 2020
First published on 10th December 2020
Abstract
Mitochondrial dysfunction, oxidative stress and inflammation are crucial contributors to liver damage and nonalcoholic fatty liver disease (NAFLD) in adulthood in offspring affected by intrauterine growth retardation (IUGR). Resveratrol (RSV) has been reported to treat and/or prevent hepatic diseases under various pathological conditions. However, the therapeutic and/or preventive effects of RSV on hepatic abnormality in IUGR adults have not been investigated until now. The effects of IUGR and RSV on the hepatic metabolic status, mitochondrial function, redox homeostasis and inflammation in pigs in adulthood were investigated. A total of 36 pairs of IUGR and normal birth weight piglets were orally fed with 80 mg RSV per kg body weight per d or vehicle (0.5% carboxymethylcellulose) for 7–21 d after birth. And then the offspring were fed with a basal diet supplemented with 300 mg RSV per kg feed or a basal diet from weaning to slaughter at 150 d. The plasma and liver samples were collected for subsequent analysis. RSV exerted beneficial effects on hepatic injury and metabolic alterations in IUGR pigs, which may be due to improved mitochondrial function and fatty acid oxidation by intensified mitochondrial biogenesis, enhanced antioxidant levels such as glutathione reductase and total superoxide dismutase activities, increased interleukin 10 gene expression and repolarization of macrophages. RSV alleviated hepatic lipid accumulation in IUGR pigs by improving mitochondrial function, redox status and inflammation, implying that it is a potential candidate for further development as an effective clinical treatment for NAFLD associated with IUGR.
1. Introduction
Intrauterine growth retardation (IUGR) is usually defined as the restricted growth and development of the embryo or its organs during gestation, as characterized by low-birth weight.1 This is a common complication of pregnancy, affecting 10–15% of pregnancies.2 Although the causes of IUGR are multifactorial, it consistently leads to deleterious health outcomes. In addition to increased perinatal morbidity and mortality, human epidemiological and animal experimental studies have demonstrated that a higher risk for nonalcoholic fatty liver disease (NAFLD) in adulthood is observed in individuals suffering from IUGR.3–7 Moreover, NAFLD found in IUGR worsens type 2 diabetes mellitus and may drive exaggerated incidence of cardiovascular–renal disease in these offspring.8
NAFLD, as characterized by excess lipid deposition in the liver, is one of the most prevalent chronic liver diseases globally that includes a spectrum of diseases ranging from simple fatty liver without hepatocellular damage to nonalcoholic steatohepatitis (NASH, fatty liver with hepatocellular injury and inflammation) with or without fibrosis that may eventually progress to cirrhosis.9–11 Simple fatty liver remains a benign outcome displaying slow progression over many years, while 10–20% of cases progress to NASH.10,12 It has been suggested that mitochondrial dysfunction, excess reactive oxygen species (ROS) over the antioxidant defense system (i.e., oxidative stress) and increased pro-inflammatory mediators such as tumor necrosis factor alpha (TNFα), interleukin (IL) 6 and IL1β (i.e., inflammation status) are related to hepatic lipid accumulation, all events of which may be involved in the progression of simple steatosis to NASH and severe fibrosis.11 Similarly, in different animal models such as rats and pigs, IUGR permanently increased lipid accumulation,4,5 impaired mitochondrial function,5,13 altered redox status,3,14 and induced inflammation3 in the liver of adult offspring, contributing to the occurrence and exacerbation of hepatic damage and dysfunction in adulthood. Moreover, in a rat model, maternal protein restriction-induced low-birth-weight offspring at 12 months of age showed hepatic fibrosis evidenced by greater collagen deposition, and higher mRNA expression of collagen type 1, α1 and profibrotic cytokine transforming growth factor β1, partly contributing to hepatic oxidative stress and inflammation.3 Considering the role of mitochondria, redox homeostasis and immune status in lipid balance, inhibition of oxidative stress, inflammation and mitochondrial dysfunction for enhancing fatty acid oxidation and reducing lipid accretion could be a promising strategy for preventing NASH and treating NAFLD in adult offspring, who experienced the process of IUGR.
Resveratrol (RSV, 3,5,4′-trihydroxystilbene), a natural polyphenol, can be extracted from many plants such as grapes, peanuts and berries, and has attracted a greater attention owing to its multiple beneficial health effects such as renoprotection,15 neuroprotection16 and hepatoprotection.17 Considerable studies conducted on two examination models (in vitro with HepG2 or primary hepatocytes, and in vivo using either Wistar rats or C57BL/6 mice treated with either high palmitate or a high-fat diet, respectively) have confirmed that RSV decreased fat accumulation, controlled mitochondrial biogenesis, diminished the secretion of pro-inflammatory mediators including IL6 and TNFα, reduced NADPH oxidase- and mitochondria-derived ROS production, and increased the activities of ROS-detoxifying enzymes such as superoxide dismutase (SOD) and glutathione peroxidase (GPX) in the liver or hepatocytes,18–22 suggesting that RSV prevented the development and progression of NAFLD caused by a bad diet. In addition, RSV has also been reported to alleviate hepatic damage under various pathological conditions such as streptozotocin-induced diabetes,23 acute carbon tetrachloride liver injury24 and primary biliary cholangitis,25 which can be partly attributed to its anti-oxidative and anti-inflammatory properties. A recent report on suckling IUGR piglets showed that RSV supplementation attenuates hepatic mitochondrial dysfunction by improving mitochondrial biogenesis and redox status, ultimately leading to a reduced triglyceride (TG) content in the liver.26 However, whether RSV could slow down the progression of NAFLD in IUGR adult offspring is unknown. Besides, pigs are widely used as biomedical models for the study of the metabolic syndrome of IUGR in humans due to the similar morphology, physiology, metabolism and proportional organ sizes between pigs and humans.5 Therefore, this study employed pigs as a model to explore the potential and preventive effects of RSV on hepatic metabolic status, mitochondrial function, oxidative stress and inflammation of IUGR offspring in adulthood.
2. Materials and methods
All animal procedures conformed to the Laboratory Animals-Standards of Welfare and Ethics (DB32/T 2911-2016) issued by Jiangsu Market Supervision and Administration Bureau. All experiments were approved by the Institutional Animal Care and Use Committee at Nanjing Agricultural University (SYXK-2017-0027).
2.1 Animals and experimental design
A total of 72 male newborn piglets from 36 sows with the same parity of the third, similar expected dates of confinement (<3 d) and litter sizes (11–13 piglets) at parturition were chosen for this experiment. In each litter, one IUGR male piglet (∼0.88 kg) and one normal birth weight (NBW) male piglet (∼1.72 kg) were selected based on their birth weight (BW) according to a previous study.26 Newborn piglets that had a BW that was close to the mean BW (within 0.5 SD) were classified as NBW piglets, whereas those with 2 SD lower BW belonged to the IUGR piglet category. All piglets were divided into 4 groups (6 replicates (pens) per group, 3 piglets per replicate): NBW piglets receiving vehicle (NBW-CON), NBW piglets receiving RSV (NBW-RSV), IUGR piglets receiving vehicle (IUGR-CON) and IUGR piglets receiving RSV (IUGR-RSV). Following birth, both NBW and IUGR offspring were cross fostered and nursed by ad libitum fed dams. During the suckling period, 36 pairs of IUGR and NBW piglets were orally treated with 80 mg RSV per kg body weight per d or vehicle by gavage from 7 to 21 days of age and remained with sows housed in lactation crates in environmentally controlled rooms. Resveratrol (purity 98%, from Zhejiang Yixin Pharmaceutical Co., Ltd, Yixin, China) was dissolved in 0.5% carboxymethylcellulose (Sinopharm Chemical Reagent Co., Ltd, Shanghai, China). All IUGR and NBW piglets in 4 groups were weaned at 21 days of age, accordingly transferred to the weaning unit, and fed with a basal diet supplemented with 300 mg RSV per kg of feed or a basal diet from weaning to slaughter at 150 days of age. Feed and water were provided ad libitum. The composition and nutrient amounts of the basal diet for sows and pigs are given in ESI Tables 1 and 2,† respectively. The duration and dosage of RSV were used in this study based on the previous studies.26–28
2.2 Sample collection
At 150 days of age, 1 pig per pen (6 pigs per group) was randomly selected. Heparinized blood samples were collected from the anterior vena cava after overnight fasting, and centrifuged at 2000g, 4 °C for 20 min to obtain plasma. The plasma samples were stored at −20 °C until analysis. Then, the pigs were euthanized by exsanguination after electrical stunning and liver samples were promptly collected and weighed. A fraction of liver samples from the left lobe were collected and fixed in 4% paraformaldehyde, while the remaining parts (about 20 g) were snap-frozen in liquid nitrogen and stored at −80 °C for further analysis.
2.3 Plasma parameters
The levels of glucose, TG, total cholesterol (TC), free fatty acids (FFA), alanine aminotransferase (ALT) and aspartate aminotransferase (AST) in the plasma were determined using the corresponding commercial kits according to the manufacturer's instruction (Nanjing Jiancheng Bioengineering Institute, Nanjing, China). A commercial enzyme-linked immunosorbent assay (ELISA) kit (CUSABIO Biotech, Wuhan, China) was used to measure the plasma insulin level. The sensitivity limit of insulin determination was 1 μIU mL−1, and the inter- and intra-assay coefficients of variation were less than 15%. The homeostasis model assessment of insulin resistance (HOMA-IR) was used to calculate the insulin resistance according to the following formula: HOMA − IR = fasting plasma insulin (μU mL−1) × fasting plasma glucose (mmol L−1)/22.5.29
2.4 Hepatic morphology
Liver samples of pigs were fixed in 4% paraformaldehyde and embedded in paraffin wax. Each paraffin-embedded sample was sectioned into 5 μm thick sections and hematoxylin and eosin (H&E) staining was performed as standard protocols. Images were collected using an optical binocular microscope (Olympus BX5; Olympus Optical Co. Ltd, Tokyo, Japan) equipped with a digital camera (Nikon H550L; Nikon, Tokyo, Japan).
2.5 Hepatic metabolites and the activities of enzymes related to lipid metabolism
The contents of TG, FFA, TC, and glycogen, and the activities of lipoprotein lipase (LPL), hepatic lipase (HL) and total lipase (TL) in the liver were measured using the corresponding commercial kits (Nanjing Jiancheng Bioengineering Institute, Nanjing, China) according to the protocols of the manufacturer. The amount of adenosine triphosphate (ATP) in the liver was determined using an ATP Content Assay Kit (Solarbio, Beijing, China) following the manufacturer's instructions.
2.6 Hepatic oxidative stress
In order to evaluate hepatic oxidative stress, the amounts of protein carbonyl (PC), malondialdehyde (MDA) and reduced glutathione (GSH), and the activities of total SOD (T-SOD), GPX and glutathione reductase (GR) were measured using commercially available kits from Nanjing Jiancheng Bioengineering Institute (Nanjing, China), according to the guidelines of the manufacturer.
2.7 Activities of the electron transfer chain (ETC) complexes
The activities of hepatic mitochondrial ETC complexes including I, II, III, IV and V were determined using commercial kits (SinoBestBio, Shanghai, China) following the manufacturer's instructions.
2.8 qRT-PCR analysis
The mRNA abundance of target and reference (β-actin) genes in the liver were analyzed as previously described.18 Briefly, total RNA was extracted from the liver using TRIzol Reagent (TaKaRa, Dalian, China) following the protocols of the manufacturer. The RNA integrity was checked on 1% agarose gel with ethidium bromide staining. The RNA concentration and purity were determined from OD260/280 readings (ratio >1.8) using a spectrophotometer (NanoDrop, 2000c; Thermo Scientific, USA). After this, total RNA was reverse-transcribed into cDNA using the PrimeScriptTM RT Reagent Kit (TaKaRa, Dalian, China) following the guidelines of the manufacturer. Primer sequences used for qRT-PCR are listed in ESI Table 3.† Real-time PCR analysis was performed on a QuantStudio®5real-time PCR Design & Analysis system (Applied Biosystems, USA) with a ChamQ SYBR qPCR Master Mix Kit (Vazyme, Nanjing, China). After normalization against β-actin gene expression, the relative mRNA amount of the target genes was calculated using the 2−ΔΔCt method.30
2.9 Mitochondrial DNA (mtDNA) content
Total genomic DNA was isolated from the snap-frozen liver using a universal Genomic DNA Extraction Kit (TSINKE, Beijing, China). The concentration of DNA was quantified and diluted to the same concentration for further analysis. The relative mtDNA content was measured by co-amplifying the mt D-loop and the nuclear-encoded β-actin gene using the qRT-PCR assay. Primer sequences are listed in ESI Table 3.† PCR amplification was performed under the same conditions as described above. The relative quantification values were calculated according to the 2−ΔΔCt method.30
2.10 Statistical analysis
Data were analyzed by ANOVA using a 2 × 2 factorial arrangement of treatments with the GLM procedure (SPSS 20.0; IBM-SPSS Inc., Chicago, IL, USA), and individual pigs served as the experimental unit for all analyses. The statistical model included the effects of RSV and BW and their interactions. When the interaction was significant, the data were reanalyzed by one-way analysis of variance and Tukey's post hoc test. Statistical differences were determined at P < 0.05, and a trend was considered at 0.05 < P < 0.10. The measurements were expressed as means with their standard errors unless otherwise stated.
3. Results
3.1 Resveratrol improves plasma FFA levels and insulin resistance in IUGR pigs
The plasma insulin content (Fig. 1E) and HOMA-IR (Fig. 1F) in IUGR pigs were higher (P < 0.05) than those in NBW pigs. Pigs exposed to RSV treatment had reduced FFA (P < 0.05, Fig. 1C) and insulin (P < 0.05) levels in the plasma as well as decreased HOMA-IR (P < 0.05). In the plasma, a relationship between RSV and BW is observed with respect to TG (P = 0.081, Fig. 1A), glucose (P < 0.05, Fig. 1D), FFA (P < 0.05), insulin (P < 0.05) and HOMA-IR (P < 0.05). Administration of RSV to IUGR pigs alleviated increased HOMA-IR, and higher FFA and insulin levels (P < 0.05). However, the concentration of TC in the plasma was not altered in the 4 groups (P > 0.05, Fig. 1B).
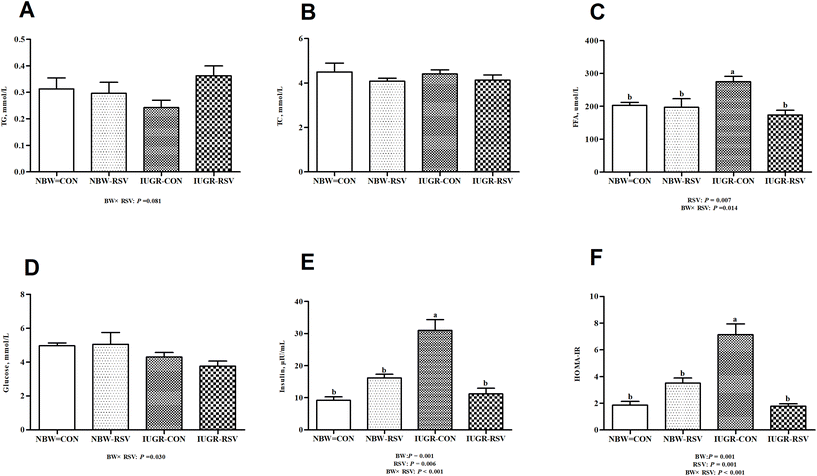 |
| Fig. 1 Resveratrol (RSV) improves free fatty acids (FFA) levels in plasma and insulin resistance in intrauterine growth retarded pigs. (A) TG, triglyceride; (B) TC, total cholesterol; (C) FFA; (D) glucose; (E) insulin; (F) HOMA-IR, homeostasis model assessment of insulin resistance. NBW-CON, normal birth weight pigs treated with vehicle; NBW-RSV, normal birth weight pigs treated with resveratrol; IUGR-CON, intrauterine growth retarded pigs treated with vehicle; IUGR-RSV, intrauterine growth retarded pigs treated with resveratrol; BW, birth weight. Results are presented as means and standard errors, n = 6. Means with different superscripts are different at P < 0.05. | |
3.2 Resveratrol alleviates hepatic injury in IUGR pigs
IUGR pigs had increased ALT (P = 0.060, Fig. 2B) levels in the plasma and reduced absolute weight of the liver (P < 0.05, Fig. 2C) compared with the NBW. Resveratrol decreased the absolute weight of the liver of pigs (P < 0.05). A relationship was observed between RSV and BW with respect to the plasma AST level (P < 0.05, Fig. 2A). Both RSV and BW had no effect on the relative weight of the liver of pigs (P > 0.05, Fig. 2D). As shown in Fig. 2E, the NBW-CON and NBW-RSV groups had a normal cell morphology, and the vacuolation of hepatocytes and the disorganization of parenchyma shown in IUGR-CON were not observed in the IUGR-RSV group.
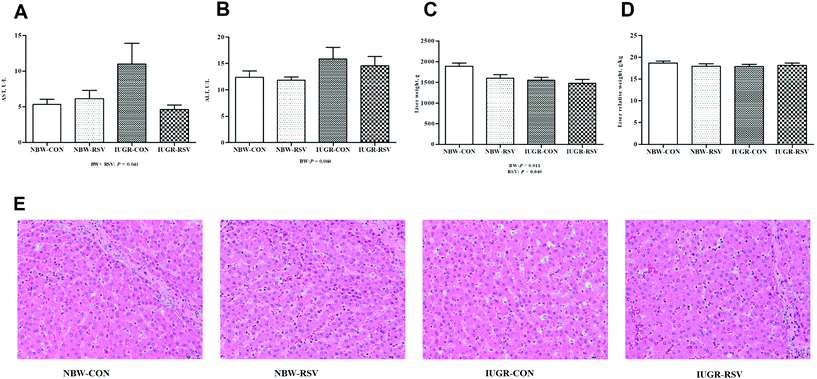 |
| Fig. 2 Resveratrol (RSV) alleviates hepatic injury in intrauterine growth retarded pigs. (A) AST, aspartate aminotransferase; (B) ALT, alanine aminotransferase; (C) liver weight; (D) liver relative weight; (E) representative HE images, scale bar = 50 μm; NBW-CON, normal birth weight pigs treated with vehicle; NBW-RSV, normal birth weight pigs treated with resveratrol; IUGR-CON, intrauterine growth retarded pigs treated with vehicle; IUGR-RSV, intrauterine growth retarded pigs treated with resveratrol; BW, birth weight. Results are presented as means and standard errors, n = 6. Means with different superscripts are different at P < 0.05. | |
3.3 Resveratrol affects hepatic lipid levels in IUGR pigs
In order to evaluate the hepatic metabolic status, lipid parameters and glycogen content were measured. The increased concentrations of TG (P < 0.05, Fig. 3A) and FFA (P < 0.05, Fig. 3C) were found in the liver of IUGR pigs when compared with the NBW. In contrast, RSV fed to pigs resulted in the lower levels of TG (P < 0.05) and FFA (P < 0.05) in the liver relative to the vehicle treatment. A relationship was observed between RSV and BW with respect to hepatic glycogen (P = 0.060, Fig. 3D) and FFA (P < 0.05) contents. Expectedly, RSV suppressed the increased FFA levels in the liver of IUGR pigs (P < 0.05). In the liver, the TC content was not changed by RSV and BW (P > 0.05, Fig. 3B).
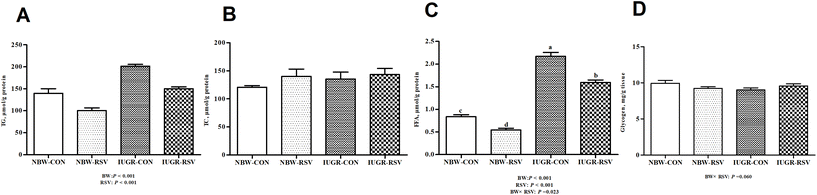 |
| Fig. 3 Resveratrol (RSV) affects hepatic lipid levels in intrauterine growth retarded pigs. (A) TG, triglyceride; (B) TC, total cholesterol; (C) FFA, free fatty acids; (D) glycogen. NBW-CON, normal birth weight pigs treated with vehicle; NBW-RSV, normal birth weight pigs treated with resveratrol; IUGR-CON, intrauterine growth retarded pigs treated with vehicle; IUGR-RSV, intrauterine growth retarded pigs treated with resveratrol; BW, birth weight. Results are presented as means and standard errors, n = 6. Means with different superscripts are different at P < 0.05. | |
3.4 Resveratrol enhances hepatic fatty acid β-oxidation to reduce lipid accumulation in IUGR pigs
Compared with the NBW, IUGR reduced HL (P < 0.05, Fig. 4B) activity, upregulated (P < 0.05, Fig. 4D) the mRNA expression of liver fatty acid binding proteins 1 (L-FABP1), and downregulated (P < 0.05, Fig. 4D) the mRNA expression of peroxisome proliferator activated receptor alpha (PPARα) and carnitine palmitoyltransferase 1 alpha (CPT1α) in the liver of pigs. Pigs fed with RSV had higher (P < 0.05) gene expression of PPARα, hormone-sensitive lipase (HSL) and CPT1α and exhibited lower (P < 0.05) gene expression of sterol regulatory element binding protein 1c (SREBP1c) and L-FABP1 in the liver. A relationship was observed between RSV and BW with respect to HL activity (P < 0.05) and gene expression of microsomal triglyceride transfer protein (P = 0.053), fatty acid transport proteins 1 (P < 0.05) and L-FABP1 (P = 0.091) in the liver. Both RSV and BW did not affect the activities of LPL (Fig. 4A) and TL (Fig. 4C), and the mRNA expression of acetyl-CoA carboxylase (ACC), fatty acid synthase (FAS), stearoyl-CoA desaturase 1 (SCD1), cluster of differentiation 36 and LPL in the liver of pigs (P > 0.05).
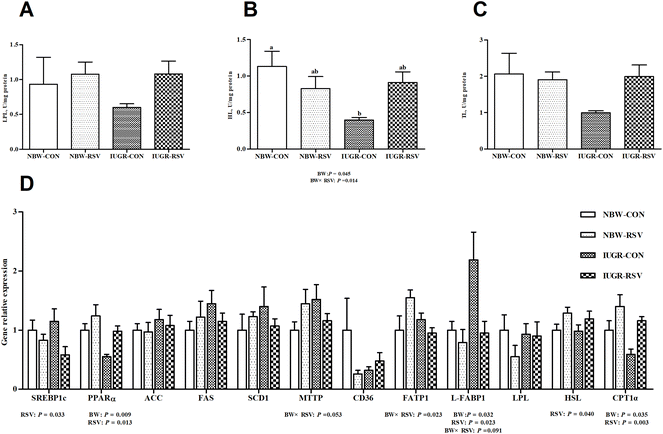 |
| Fig. 4 Resveratrol (RSV) increases hepatic fatty acid β-oxidation to reduce lipid accumulation in intrauterine growth retarded pigs. (A) LPL, lipoprotein lipase, n = 6; (B) HL, hepatic lipase, n = 6; (C) TL, total lipase, n = 6; (D) gene expression related to lipid metabolism, n = 5; SREBP1c, sterol regulatory element binding protein 1c; PPARα, peroxisome proliferator activated receptor alpha; ACC, acetyl-CoA carboxylase; FAS, fatty acid synthase; SCD1, stearoyl-CoA desaturase 1; MTTP, microsomal triglyceride transfer protein; CD36, cluster of differentiation 36; FATP1, fatty acid transport protein 1; L-FABP1, liver fatty acid binding protein 1; HSL, hormone-sensitive lipase; CPT1α, carnitine palmitoyltransferase 1 alpha; NBW-CON, normal birth weight pigs treated with vehicle; NBW-RSV, normal birth weight pigs treated with resveratrol; IUGR-CON, intrauterine growth retarded pigs treated with vehicle; IUGR-RSV, intrauterine growth retarded pigs treated with resveratrol; BW, birth weight. Results are presented as means and standard errors. Means with different superscripts are different at P < 0.05. | |
3.5 Resveratrol improves hepatic mitochondrial dysfunction in IUGR pigs
Table 1 shows that the activities of complex I (P = 0.060) and III (P < 0.05), and the levels of ATP (P < 0.05) and mtDNA (P < 0.05) were lower in the liver of IUGR pigs compared with NBW pigs. Resveratrol treatment increased the activities of complex II (P < 0.05), III (P < 0.05) and V (P = 0.097), and the levels of ATP (P < 0.05) and mtDNA (P < 0.05) in the liver of pigs. In Fig. 5, IUGR inhibited the gene expression related to mitochondrial biogenesis (sirtuin 1 (SIRT1, P = 0.088), nuclear respiratory factor 1 (NRF1, P = 0.097), estrogen-related receptor alpha (ERRα, P < 0.05), polymerase gamma (POLG, P < 0.05)) and energy metabolism (Cytochrome c oxidase IV (COXIV, P = 0.072), ATP synthase alpha subunit (ATP5A1, P = 0.090), ATP synthase beta polypeptide (ATP5B1, P < 0.05), ATP synthase F0 complex subunit C1 (ATP5G1, P < 0.05) and ubiquinol-Cytochrome c reductase binding protein (P = 0.098)). Fortunately, RSV increased the mRNA expression of SIRT1 (P < 0.05), peroxisome proliferation activated receptor gamma coactivator-1 alpha (PGC1α, P < 0.05), NRF1 (P < 0.05), ERRα (P < 0.05), mitochondrial transcription factor A (TFAM, P < 0.05), POLG (P < 0.05), NADH dehydrogenase (ubiquinone) 1 alpha subcomplex 4 (P = 0.051), NADH dehydrogenase (ubiquinone) 1 alpha subcomplex 13 (P = 0.055), NADH dehydrogenase (ubiquinone) 1 beta subcomplex 1 (NDUFB1, P = 0.096), succinate dehydrogenase complex flavoprotein subunit A (P < 0.05), succinate dehydrogenase complex iron sulfur subunit B (P = 0.059), COXIV (P < 0.05), COXV (P < 0.05), ATP5A1 (P < 0.05), ATP5B1 (P < 0.05), ATP5G1 (P < 0.05), and Cytochrome C (CtyC, P < 0.05) in the liver of pigs. A relationship was observed between RSV and BW with respect to the contents of ATP (P < 0.05) and mtDNA (P < 0.05), and the mRNA expression of NDUFB1 (P = 0.071) and CytC (P < 0.05) in the liver. RSV alleviated the decreased mtDNA number, ATP production and CtyC gene expression in the liver of IUGR pigs (P < 0.05). Complex IV activity, NADH dehydrogenase (ubiquinone) 1 alpha subcomplex 1 and NADH dehydrogenase (ubiquinone) 1 alpha subcomplex 6 gene expression in the liver were not altered among 4 groups (P > 0.05).
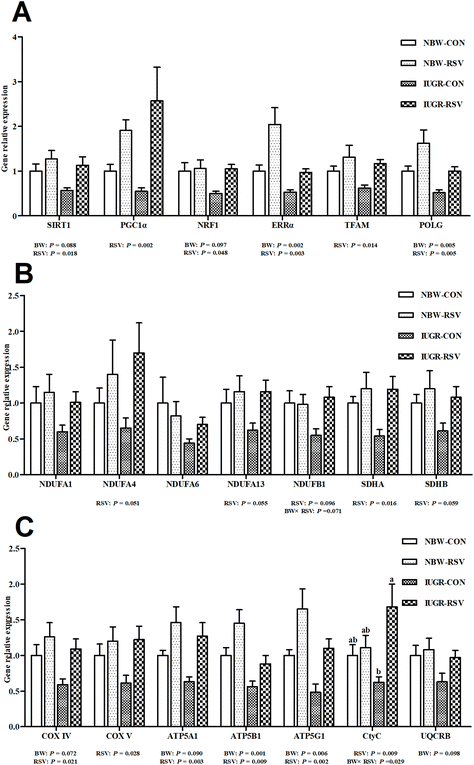 |
| Fig. 5 Resveratrol (RSV) upregulates the transcriptional expression of genes related to hepatic mitochondrial biogenesis and energy metabolism in intrauterine growth retarded pigs. (A) Gene expression related to mitochondrial biogenesis; (B and C) gene expression related to energy metabolism; SIRT1, sirtuin 1; PGC-1α, peroxisome proliferation activated receptor gamma coactivator-1 alpha; NRF1, nuclear respiratory factor 1; ERRα, estrogen-related receptor alpha; TFAM, mitochondrial transcription factor A; ERRα, estrogen-related receptor alpha; POLG, polymerase gamma; NDUFA1, NADH dehydrogenase (ubiquinone) 1 alpha subcomplex 1; NDUFA4, NADH dehydrogenase (ubiquinone) 1 alpha subcomplex 4; NDUFA6, NADH dehydrogenase (ubiquinone) 1 alpha subcomplex 6; NDUFA13, NADH dehydrogenase (ubiquinone) 1 alpha subcomplex 13; NDUFB1, NADH dehydrogenase (ubiquinone) 1 beta subcomplex 1; SDHA, succinate dehydrogenase complex flavoprotein subunit A; SDHB, succinate dehydrogenase complex iron sulfur subunit B; COX IV, Cytochrome c oxidase IV; COX V, Cytochrome c oxidase V; ATP5A1, ATP synthase alpha subunit; ATP5B, ATP synthase beta polypeptide; ATP5G1, ATP synthase F0 complex subunit C1; CytC, Cytochrome C; UQCRB, ubiquinol-Cytochrome c reductase binding protein; NBW-CON, normal birth weight pigs treated with vehicle; NBW-RSV, normal birth weight pigs treated with resveratrol; IUGR-CON, intrauterine growth retarded pigs treated with vehicle; IUGR-RSV, intrauterine growth retarded pigs treated with resveratrol; BW, birth weight. Results are presented as means and standard errors, n = 5. Means with different superscripts are different at P < 0.05. | |
Table 1 Effects of RSV and IUGR on electron transfer chain complex activities, adenosine triphosphate and mitochondrial DNA content in the liver of pigs
Items |
NBW-CON |
NBW-RSV |
IUGR-CON |
IUGR-RSV |
SEM |
P-value |
BW |
RSV |
BW × RSV |
RSV, resveratrol; IUGR, intrauterine growth retardation; NBW-CON, normal birth weight pigs treated with vehicle; NBW-RSV, normal birth weight pigs treated with resveratrol; IUGR-CON, intrauterine growth retarded pigs treated with vehicle; IUGR-RSV, intrauterine growth retarded pigs treated with resveratrol; SEM, pooled standard errors; BW, birth weight. Results are presented as means and their pooled standard errors, n = 6. Within the same row, means with different superscripts are different at p < 0.05. |
Complex I, nmol per min per mg protein |
506.96 |
583.66 |
663.99 |
992.46 |
73.77 |
0.060 |
0.121 |
0.307 |
Complex II, nmol per min per mg protein |
12.52 |
28.60 |
6.77 |
32.74 |
3.74 |
0.901 |
0.004 |
0.449 |
Complex III, nmol per min per mg protein |
153.51 |
278.08 |
97.07 |
168.90 |
17.53 |
0.002 |
<0.001 |
0.276 |
Complex IV, nmol per min per mg protein |
4.07 |
4.17 |
3.31 |
4.52 |
0.35 |
0.783 |
0.380 |
0.454 |
Complex V, nmol per min per mg protein |
12.05 |
19.75 |
12.11 |
20.14 |
2.26 |
0.961 |
0.097 |
0.970 |
Adenosine triphosphate, nmol per g tissue |
60.45b |
77.55a |
31.94c |
74.09ab |
4.19 |
0.001 |
<0.001 |
0.006 |
Mitochondrial DNA content, fold change |
1.00a |
0.97a |
0.47b |
0.93a |
0.06 |
0.002 |
0.017 |
0.007 |
3.6 Resveratrol maintains optimal redox status in IUGR pigs
IUGR pigs exhibited higher PC (P < 0.05, Fig. 6A) and MDA (P < 0.05, Fig. 6B) contents, and lower GR (P < 0.05, Fig. 6F) activity in the liver compared with NBW pigs. Compared with pigs fed with the vehicle, pigs treated with RSV had a lower MDA (P < 0.05) level, increased T-SOD (P = 0.096, Fig. 6D) and GR (P = 0.072) activities in the liver. At transcriptional levels (Fig. 6G), IUGR downregulated the mRNA expression of nuclear factor erythroid-derived 2-like 2 (Nrf2) (P < 0.05), Kelch-like ECH-associated protein 1 (Keap1) (P < 0.05), heme oxygenase 1 (HO1) (P = 0.088), glutamate–cysteine ligase catalytic subunit (P < 0.05), and GR (P < 0.05) in the liver of pigs; RSV upregulated the mRNA expression of HO1 (P < 0.05), glutamate–cysteine ligase modifier subunit (GCLM, P < 0.05), GPX1 (P = 0.065), GPX4 (P < 0.05), and GR (P < 0.05) in the liver of pigs. A relationship was observed between RSV and BW with respect to the MDA content (P < 0.05), PC level (P < 0.05), GR activity (P < 0.05), and the mRNA expression of Nrf2 (P = 0.085), HO1 (P = 0.066), SOD1 (P = 0.060), GCLM (P = 0.081) and GPX4 (P = 0.056) in the liver of pigs. As expected, RSV normalized the increased MDA content and diminished GR activity in the liver of IUGR pigs (P < 0.05). In the liver, the GSH level (Fig. 6C), GPX activity (Fig. 6E), and SOD2 mRNA expression (Fig. 6G) were not altered by BW and RSV (P > 0.05).
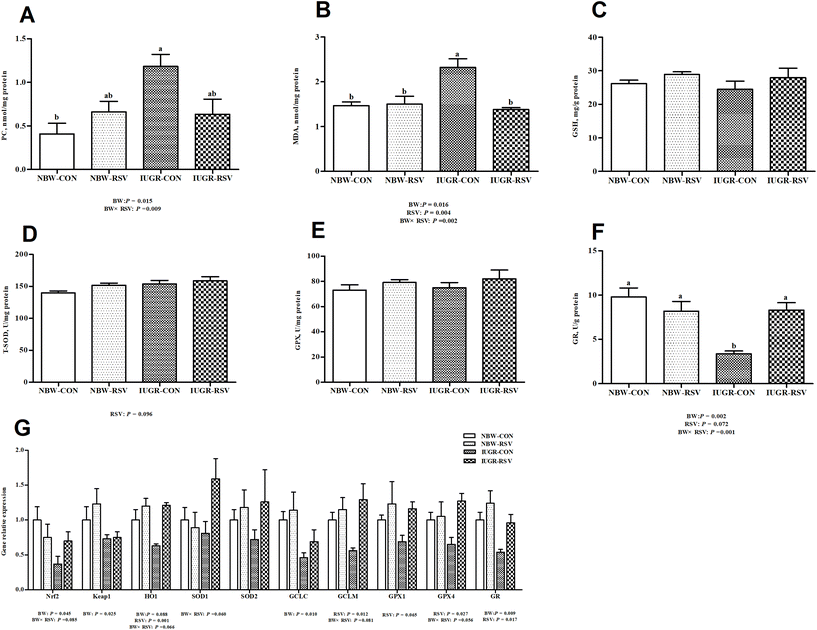 |
| Fig. 6 Resveratrol (RSV) maintains an optimal redox status in intrauterine growth retarded pigs. (A) PC, protein carbonyl, n = 6; (B) MDA, malondialdehyde, n = 6; (C) GSH, reduced glutathione, n = 6; (D) T-SOD, total superoxide dismutase, n = 6; (E) GPX, glutathione peroxidase, n = 6; (F) GR, glutathione reductase, n = 6; (G) gene expression involved in redox status, n = 5. Nrf2, nuclear factor erythroid-derived 2-like 2; Keap1, Kelch-like ECH-associated protein 1; HO1, heme oxygenase 1; GCLC, glutamate–cysteine ligase catalytic subunit; GCLM, glutamate–cysteine ligase modifier subunit; NBW-CON, normal birth weight pigs treated with vehicle; NBW-RSV, normal birth weight pigs treated with resveratrol; IUGR-CON, intrauterine growth retarded pigs treated with vehicle; IUGR-RSV, intrauterine growth retarded pigs treated with resveratrol; BW, birth weight. Results are presented as means and standard errors. Means with different superscripts are different at P < 0.05. | |
3.7 Resveratrol regulates hepatic inflammation gene expression in IUGR pigs
As shown in Fig. 7, compared with the NBW, IUGR increased the gene expression of toll-like receptor 4 (TLR4, P < 0.05), IL6 (P = 0.052), TNFα (P < 0.05), and IL1β (P = 0.071) in the liver of pigs. Interestingly, RSV reduced the mRNA expression of monocyte chemotactic protein 1 (MCP1, P = 0.083) and nuclear factor-κB p65 (P = 0.065), and increased IL10 (P = 0.093) gene expression in the liver of pigs. A relationship was observed between RSV and BW with respect to integrin alpha X (CD11c) (P < 0.05), adhesion G protein-coupled receptor E1 (P = 0.072), myeloid differentiation factor 88 (P < 0.05), and p65 (P = 0.069) mRNA expression in the liver. Compared with the IUGR-CON group, hepatic CD11c mRNA expression in the IUGR-RSV group was lower. The gene expression of hepatic tumor necrosis factor receptor-associated factor 6 in pigs did not differ between the BW and RSV-exposed groups (P > 0.05).
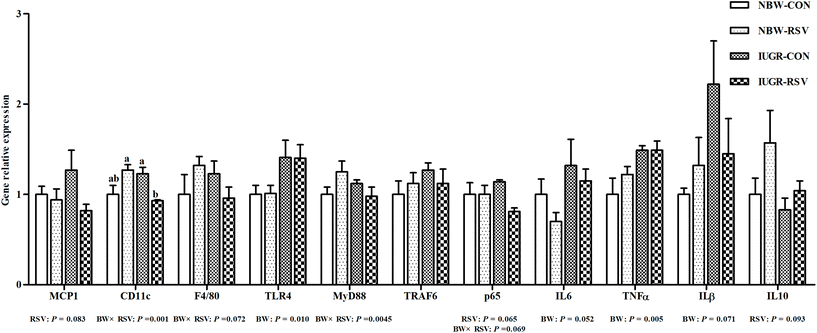 |
| Fig. 7 Resveratrol (RSV) regulates hepatic inflammation gene expression in intrauterine growth retarded pigs. MCP1, monocyte chemotactic protein 1; CD11c, integrin alpha X; F4/80, adhesion G protein-coupled receptor E1; TLR4, toll-like receptor 4; MyD88, myeloid differentiation factor 88; TRAF6, tumor necrosis factor receptor-associated factor 6; p65, nuclear factor-κB; TNFα, tumor necrosis factor alpha; IL6, interleukin 6; IL1β, interleukin 1β; IL10, interleukin 10; NBW-CON, normal birth weight pigs treated with vehicle; NBW-RSV, normal birth weight pigs treated with resveratrol; IUGR-CON, intrauterine growth retarded pigs treated with vehicle; IUGR-RSV, intrauterine growth retarded pigs treated with resveratrol; BW, birth weight. Results are presented as means and standard errors, n = 5. Means with different superscripts are different at P < 0.05. | |
4. Discussion
Accumulating evidence demonstrated that an abnormal intrauterine milieu can have long-lasting effects on the offspring and increases the risk of NAFLD, which endangers public health. Data for both children and adults suggest that low-birth weight induced by IUGR is associated with an increased risk of developing NAFLD.31 In the present study, the results in a pig model corroborated the previous studies and showed that IUGR led to hepatic injury and dysfunction in adult offspring evidenced by increased plasma ALT activity, aberrant hepatic histology, hepatic lipid accretion, oxidative damage, pro-inflammatory changes and mitochondrial dysfunction in offspring. Interestingly, RSV alleviated liver injury and hepatic lipid accretion in IUGR pigs by several actions including increased mitochondrial fatty acid oxidation and biogenesis, enhanced antioxidant enzyme activities, increased anti-inflammatory cytokine production and repolarization of macrophages. Besides, in this study, we found that IUGR pigs exhibited hyperinsulinemia with normal fasting glucose levels, increased plasma FFA levels and higher HOMA-IR, indicating that abnormal metabolism occurred in IUGR pigs at 150 days of age, which may be related to whole-body insulin resistance. The phenomenon was also attenuated by RSV, which may be partly associated with the beneficial effects of RSV on hepatic injury and lipid deposition in IUGR pigs.
Abnormal lipid accumulation is an important feature of NAFLD and occurs due to an increased free fatty acid input correlated with its inefficient β-oxidation, esterification, or both,17 ultimately leading to progress to NASH and liver failure. The increased hepatic lipid content in IUGR adult pigs may be related to continued downregulation of PPARα, which upregulates the gene expression involved in fatty acid oxidation to reduce hepatic lipid storage.32 In a rat model, pups from dams subjected to 50% food-restriction from pregnancy day 10 to 21 showed a decrease in PPARα at 1 day of age and 9 months of age and exhibited upregulation of SREBP1c and FAS at 9 months of age, resulting in an elevated TG content.4 Similar results observed in a pig model showed that IUGR adult offspring had higher TG levels in the liver partly due to downregulated expression of hepatic CPT1 which is the rate-limiting enzyme that determines fatty acid oxidation.5 In this study, reduced PPARα and its target gene (i.e., CPT1α) expression, and increased L-FABP1 gene expression were found in the liver of IUGR pigs at 150 days of age. L-FABP1 is known to be critical for fatty acid uptake and intracellular transport and also has an important role in regulating lipid metabolism.33 Besides, IUGR significantly reduced hepatic HL activity, which catalyzes the hydrolysis of triacylglycerol in circulating lipoproteins such as chylomicron and very low-density lipoprotein,34 providing FFA and glycerol for tissue storage or utilization. Thus, lipid accretion in the liver of IUGR pigs at 150 days of age could be attributed to impaired fatty acid oxidation capacity and increased FFA uptake directly from the circulation by L-FABP1 rather than HL. In addition to the above adverse results, RSV also improved SREBP1c and HSL gene expression. SREBP1c regulates the induction of genes for enzymes (e.g., FAS, SCD1 and ACC) involved in the biosynthesis of fatty acids and TG. However, RSV did not affect hepatic lipogenesis enzyme gene expression, suggesting that RSV had no effects on hepatic de novo fatty acid synthesis in the liver of pigs. HSL is an intracellular neutral lipase catalyzing the hydrolysis of hepatocellular TG and other lipids,35 leading to FFA generation for utilization. In view of the above-mentioned facts, we concluded that RSV reduced lipid accumulation in the liver through enhanced lipolysis and fatty acid β-oxidation as well as inhibited FFA uptake, suggesting that RSV may prevent and or treat hepatic lipid accretion in IUGR offspring in adulthood. Similar results were found in the application of RSV to suckling IUGR piglets.26 In addition, the anti-oxidative and anti-inflammatory effects of RSV on the liver of IUGR pigs may also be beneficial for intensifying mitochondrial fatty acid β-oxidation and reducing lipid accumulation. A previous study also showed that inhibited intestinal TG absorption by RSV may contribute to the improvement of fatty liver induced by a high-fat diet in rats.36 Whether RSV could suppress dietary lipid absorption in IUGR pigs needs to be further studied.
Mitochondria are the primary site not only for fatty acid β-oxidation, but also for ROS production, which makes them susceptible to oxidative stress. Mitochondrial dysfunction would promote mitochondrial ROS formation, further exacerbating hepatic injury and the deterioration of lipid metabolism. The close relationship between mitochondrial dysfunction and NAFLD has been reviewed in the previous research.37 IUGR affects mitochondrial oxidative metabolism in the liver in part by suppressing the protein components of ETC and mitochondrial biogenesis, which have been demonstrated in suckling26 and adult5 pigs. Consistent with these results, we also found that the contents of mtDNA and ATP, complexes activities, the gene expression related to mitochondrial biogenesis and energy metabolism were adversely affected in IUGR pigs. These results suggested that IUGR has long-lasting adverse effects on hepatic mitochondrial function, which consequently leads to hepatic injury and lipid accumulation in pigs. Fortunately, these impairments were attenuated by RSV in the liver. These results indicated that RSV improved hepatic mitochondrial function, which may be a contributor to reduced lipid contents and injury in the liver of IUGR pigs. The protective effects of RSV on mitochondria in different experimental models have been reviewed in detail by others.38,39 The protective effects of RSV on the hepatic mitochondrial function of suckling IUGR piglets have been reported.26 However, it is the first time that the role of RSV in the liver of IUGR adult pigs has been investigated. We should consider the role of the SIRT1-PGC1α pathway with regard to the effects of IUGR and RSV on mitochondrial function. SIRT1, a deacetylase and an important modulator of energy metabolism, can regulate mitochondrial biogenesis through PGC1α.40 PGC1α is a potent coactivator of the central transcription factor NRF1 in regulating the transcription of the TFAM gene, which controls the sole promoter of mitochondrial coding genes and replication of mtDNA.41 The regulation of SIRT1 on the downstream key transcription factors is dependent on its deacetylation activity. Therefore, although the expression of genes related to the SIRT1-PGC1 pathway was determined in the present study, the post-translational and translational mechanisms of the SIRT1-PGC1α pathway about the role of RSV in the liver of IUGR pigs need to be further explored.
The development and progression of NAFLD are associated with excessive free radical generation and the disruption of redox status, which are commonly defined as oxidative stress. Excess free radical can damage mitochondrial function and induce cell death, consequently leading to hepatic lipid accretion and liver injury. Accumulation of ROS and impairment of the antioxidant defense system were reported in hepatic tissues of mice exposed to a high-fat diet.18,42 Similar results were observed in the liver tissues of adult rats3,14 and piglets43,44 after they were exposed to IUGR. In line with these observations, we also found decreased GR activity, and increased contents of MDA and PC (a marker of lipid peroxidation and protein oxidation, respectively, and oxidative stress) in the liver of adult pigs after subjection to IUGR, indicating that hepatic oxidative stress permanently occurred and may be an important contributor to the development of NAFLD in adults associated with IUGR. In the present study, the reduced GR mRNA expression in the liver of IUGR pigs may explain the lower GR activity. Hepatic oxidative stress in IUGR pigs may be related to the suppressed expression of Nrf2 and its target genes such as HO1, GCLC and GR, which have been demonstrated to play a beneficial role in resisting oxidative stress and avoiding cell damage.45 Expectedly, RSV attenuated increased MDA content and inhibited GR activity in the liver of IUGR pigs. Moreover, RSV enhanced T-SOD activity, and upregulated the gene expression of HO1, GCLM, GPX1, GPX4 and GR in the liver. However, RSV had no effect on Nrf2 expression, suggesting that RSV regulated the expression of genes related to antioxidants independent of the Nrf2 transcriptional expression. The detailed molecular mechanism needs to be investigated in the future. Many studies have demonstrated that RSV significantly reduces the level of factors associated with oxidative stress, contributing to the attenuation of hepatocyte destruction and NAFLD development induced by diet in rodents, which have been reviewed in detail by Charytoniuk et al.17 A recent research in finishing pigs on the beneficial effects of RSV on muscular antioxidant capacity had also been reported.28 It should be noted that, although increased mitochondrial fatty acid oxidation by RSV can result in an increase in oxidative products (i.e., ROS),46 RSV still reduced the hepatic MDA content of IUGR pigs. Thus, the alleviation of hepatic OS by RSV was mainly due to enhanced antioxidant enzyme activities rather than suppressed ROS production and may exert a beneficial role in the prevention and treatment of NAFLD associated with IUGR in adult offspring.
Increased pro-inflammatory mediators have been reported to implicate in the pathogenesis of NAFLD/NASH in IUGR offspring.8 Inflammation is associated with ROS generation, which could promote mitochondrial dysfunction, lipid accumulation and liver injury. In the present study, IUGR increased hepatic IL6, IL1β and TNFα gene expression of pigs at 150 days of age, indicating that IUGR induced hepatic inflammation in adult pups. Similar findings in a rat model showed that hepatic proteins of TNFα and IL6 were upregulated in IUGR offspring at 12 months.3 In this study, hepatic inflammation of IUGR pigs may be associated with the upregulation of TLR4 mRNA. As a well-known pattern recognition receptor, TLR4 is activated by the corresponding ligands and then the pro-inflammatory cytokine (e.g., TNFα and IL6) production is stimulated. Evidence in rodents,47 in hepatocytes,48 and in patients49 suggested that the increase in hepatic TLR 4 is associated with the progression from NAFLD to NASH. Thus, in the present study, upregulation of TLR4 led to hepatic inflammation, which may play a critical role in the development of NAFLD associated with IUGR. Interestingly, RSV downregulated MCP1 and p65 mRNA levels, and upregulated IL10 gene expression in the liver of IUGR and NBW pigs. Moreover, RSV reduced hepatic CD11c gene expression in IUGR pigs rather than in NBW pigs. It is well known that hepatic inflammation is associated with macrophage polarization. During inflammation, macrophages can be activated and divided into 2 phenotypes: pro-inflammatory M1 macrophages and anti-inflammatory M2 macrophages.50 M1 macrophages can produce chemokines such as MCP1 and pro-inflammatory cytokines such as TNFα and IL6; M2 macrophages can generate the anti-inflammatory IL10 cytokine.51,52 CD11c is a well-known marker of M1 macrophages.53 A previous study demonstrated that overexpression of IL10 could restrain pro-inflammatory responses and ameliorate obesity and diabetes.54 Similar results were found in obese mice fed with a high-fat diet, which showed that RSV attenuated renal inflammation in part by facilitating macrophage phenotypic switch from pro-inflammatory M1 to anti-inflammatory M2.15 Although gene expression of pro-inflammatory cytokines was not downregulated by RSV, these results could conclude that RSV may counteract IUGR-induced inflammation in the liver partly by the increase in IL10 gene expression and repolarization of liver tissue macrophages from an M1 to an M2 phenotype, which may prevent the development of NAFLD in IUGR offspring.
5. Conclusions
In summary, the results indicated that RSV exerted beneficial effects on hepatic damage, lipid accumulation, mitochondrial dysfunction, oxidative stress and inflammation caused by IUGR in pigs, suggesting that RSV may be a promising hepatoprotective agent for the treatment of NAFLD in humans associated with IUGR.
Conflicts of interest
The authors declare that they have no conflict of interest.
Acknowledgements
This study was supported by the Fundamental Research Funds for the Central Universities (Grants No. KJQN 201935), the National Natural Science Foundation of China (Grants No. 31802101, 31772634, and 31802094), and the Natural Science Foundation of Jiangsu Province (Grants No. BK20180531).
References
- S. Longo, A. Borghesi, C. Tzialla and M. Stronati, IUGR and infections, Early Hum. Dev., 2014, 90(Suppl 1), S42–S44 CrossRef.
- C. S. Rashid, A. Bansal and R. A. Simmons, Oxidative Stress, Intrauterine Growth Restriction, and Developmental Programming of Type 2 Diabetes, Physiology, 2018, 33, 348–359 CrossRef CAS.
- J. L. Tarry-Adkins, D. S. Fernandez-Twinn, I. P. Hargreaves, V. Neergheen, C. E. Aiken, M. S. Martin-Gronert, J. M. McConnell and S. E. Ozanne, Coenzyme Q10 prevents hepatic fibrosis, inflammation, and oxidative stress in a male rat model of poor maternal nutrition and accelerated postnatal growth, Am. J. Clin. Nutr., 2016, 103, 579–588 CrossRef CAS.
- T. R. Magee, G. Han, B. Cherian, O. Khorram, M. G. Ross and M. Desai, Down-regulation of transcription factor peroxisome proliferator-activated receptor in programmed hepatic lipid dysregulation and inflammation in intrauterine growth-restricted offspring, Am. J. Obstet. Gynecol., 2008, 199, 271.e1–271.e5 CrossRef.
- L. Shen, M. Gan, S. Zhang, J. Ma, G. Tang, Y. Jiang, M. Li, J. Wang, X. Li, L. Che and L. Zhu, Transcriptome Analyses Reveal Adult Metabolic Syndrome With Intrauterine Growth Restriction in Pig Models, Front. Genet., 2018, 9, 291 CrossRef.
- M. Pruis, P. van Ewijk, V. Schrauwen-Hinderling and T. Plösch, Lipotoxicity and the role of maternal nutrition, Acta Physiol., 2014, 210, 296–306 CrossRef CAS.
- A. Fraser, S. Ebrahim, G. D. Smith and D. A. Lawlor, The associations between birthweight and adult markers of liver damage and function, Paediatr. Perinat. Epidemiol., 2008, 22, 12–21 Search PubMed.
- F. T. Spradley, J. A. Smith, B. T. Alexander and C. D. Anderson, Developmental origins of nonalcoholic fatty liver disease as a risk factor for exaggerated metabolic and cardiovascular-renal disease, Am. J. Physiol. Endocrinol. Metab., 2018, 315, E795–E814 CrossRef CAS.
- R. M. Williamson, J. F. Price, S. Glancy, E. Perry, L. D. Nee, P. C. Hayes, B. M. Frier, L. A. Van Look, G. I. Johnston and R. M. Reynolds, Prevalence of and Risk Factors for Hepatic Steatosis and Nonalcoholic Fatty Liver Disease in People With Type 2 Diabetes: the Edinburgh Type 2 Diabetes Study, Diabetes Care, 2011, 34, 1139–1144 CrossRef.
- M. Li, C. M. Reynolds, S. A. Segovia, C. Gray and M. H. Vickers, Developmental Programming of Nonalcoholic Fatty Liver Disease: The Effect of Early Life Nutrition on Susceptibility and Disease Severity in Later Life, Biomed Res. Int., 2015, 2015, 437107 Search PubMed.
- A. Alisi, N. Panera, C. Agostoni and V. Nobili, Intrauterine Growth Retardation and Nonalcoholic Fatty Liver Disease in Children, Int. J. Endocrinol., 2011, 2011, 269853 Search PubMed.
- G. Vernon, A. Baranova and Z. M. Younossi, Systematic review: the epidemiology and natural history of non-alcoholic fatty liver disease and non-alcoholic steatohepatitis in adults, Aliment. Pharmacol. Ther., 2011, 34, 274–285 CrossRef CAS.
- I. E. Peterside, M. A. Selak and R. A. Simmons, Impaired oxidative phosphorylation in hepatic mitochondria in growth-retarded rats, Am. J. Physiol. Endocrinol. Metab., 2003, 285, E1258–E1266 CrossRef CAS.
- E. L. Raab, P. M. Vuguin, D. A. Stoffers and R. A. Simmons, Neonatal exendin-4 treatment reduces oxidative stress and prevents hepatic insulin resistance in intrauterine growth-retarded rats, Am. J. Physiol.: Regul., Integr. Comp. Physiol., 2009, 297, R1785–R1794 CrossRef CAS.
- K. Cheng, Z. Song, Y. Chen, S. Li, Y. Zhang, H. Zhang, L. Zhang, C. Wang and T. Wang, Resveratrol protects against renal damage via attenuation of inflammation and oxidative stress in high-fat-diet-induced obese mice, Inflammation, 2019, 42, 937–945 CrossRef CAS.
- P. Maher, R. Dargusch, L. Bodai, P. E. Gerard, J. M. Purcell and J. L. Marsh, ERK activation by the polyphenols fisetin and resveratrol provides neuroprotection in multiple models of Huntington's disease, Hum. Mol. Genet., 2011, 20, 261–270 CrossRef CAS.
- T. Charytoniuk, K. Drygalski, K. Konstantynowicznowicka, K. Berk and A. Chabowski, Alternative treatment methods attenuate the development of NAFLD: a review of resveratrol molecular mechanisms and clinical trials, Nutrition, 2017, 34, 108–117 CrossRef CAS.
- K. Cheng, Z. Song, H. Zhang, S. Li, C. Wang, L. Zhang and T. Wang, The therapeutic effects of resveratrol on hepatic steatosis in high-fat diet-induced obese mice by improving oxidative stress, inflammation and lipid-related gene transcriptional expression, Med. Mol. Morphol., 2019, 52, 187–197 CrossRef CAS.
- M. Lagouge, C. Argmann, Z. Gerhart-Hines, H. Meziane, C. Lerin, F. Daussin, N. Messadeq, J. Milne, P. Lambert, P. Elliott, B. Geny, M. Laakso, P. Puigserver and J. Auwerx, Resveratrol improves mitochondrial function and protects against metabolic disease by activating SIRT1 and PGC-1alpha, Cell, 2006, 127, 1109–1122 CrossRef CAS.
- S. H. Jin, J. H. Yang, B. Y. Shin, K. Seo, S. M. Shin, I. J. Cho and S. H. Ki, Resveratrol inhibits LXRα-dependent hepatic lipogenesis through novel antioxidant Sestrin2 gene induction, Toxicol. Appl. Pharmacol., 2013, 271, 95–105 CrossRef CAS.
- J. Shang, L. L. Chen, F. X. Xiao, H. Sun, H. C. Ding and H. Xiao, Resveratrol improves non-alcoholic fatty liver disease by activating AMP-activated protein kinase, Acta Pharmacol. Sin., 2008, 29, 698–706 CrossRef CAS.
- M. M. Poulsen, J. Larsen, S. Hamilton-Dutoit, B. F. Clasen, N. Jessen, S. K. Paulsen, T. N. Kjær, B. Richelsen and S. B. Pedersen, Resveratrol up-regulates hepatic uncoupling protein 2 and prevents development of nonalcoholic fatty liver disease in rats fed a high-fat diet, Nutr. Res., 2012, 32, 701–708 CrossRef CAS.
- H. S. Aktaş, Y. Ozel, S. Ahmad, H. H. Pençe, B. Ayaz-Adakul, I. Kudas, S. Tetik, T. Şekerler, C. Canbey-Göret, L. Kabasakal and H. K. Elcioglu, Protective effects of resveratrol on hepatic ischemia reperfusion injury in streptozotocin-induced diabetic rats, Mol. Cell. Biochem., 2019, 460, 217–224 CrossRef.
- C. C. Chan, K. C. Lee, Y. H. Huang, C. K. Chou, H. C. Lin and F. Y. Lee, Regulation by resveratrol of the cellular factors mediating liver damage and regeneration after acute toxic liver injury, J. Gastroenterol. Hepatol., 2014, 29, 603–613 CrossRef CAS.
- Y. Li, Y. Xi, G. Tao, G. Xu, Z. Yang, X. Fu, Y. Liang, J. Qian, Y. Cui and T. Jiang, Sirtuin 1 activation alleviates primary biliary cholangitis via the blocking of the NF-κB signaling pathway, Int. Immunopharmacol., 2020, 83, 106386 CrossRef CAS.
- H. Zhang, Y. Li, W. Su, Z. Ying, L. Zhou, L. Zhang and T. Wang, Resveratrol attenuates mitochondrial dysfunction in the liver of intrauterine growth retarded suckling piglets by improving mitochondrial biogenesis and redox status, Mol. Nutr. Food Res., 2017, 61, 1600653 CrossRef.
- Z. Gan, W. Wei, Y. Li, J. Wu, Y. Zhao, L. Zhang, T. Wang and X. Zhong, Curcumin and Resveratrol Regulate Intestinal Bacteria and Alleviate Intestinal Inflammation in Weaned Piglets, Molecules, 2019, 24, 1220 CrossRef.
- C. Zhang, J. Luo, B. Yu, P. Zheng, Z. Huang, X. Mao, J. He, J. Yu, J. Chen and D. Chen, Dietary resveratrol supplementation improves meat quality of finishing pigs through changing muscle fiber characteristics and antioxidative status, Meat Sci., 2015, 102, 15–21 CrossRef CAS.
- D. R. Matthews, J. P. Hosker, A. S. Rudenski, B. A. Naylor, D. F. Treacher and R. C. Turner, Homeostasis model assessment: insulin resistance and β-cell function from fasting plasma glucose and insulin concentrations in man, Diabetologia, 1985, 28, 412–419 CrossRef CAS.
- K. J. Livak and T. D. Schmittgen, Analysis of relative gene expression data using real-time quantitative PCR and the 2(-Delta Delta C(T)) Method, Methods, 2001, 25, 402–408 CrossRef CAS.
- K. P. Newton, H. S. Feldman, C. D. Chambers, L. Wilson, C. Behling, J. M. Clark, J. P. Molleston, N. Chalasani, A. J. Sanyal, M. H. Fishbein, J. E. Lavine and J. B. Schwimmer, Low and High Birth Weights Are Risk Factors for Nonalcoholic Fatty Liver Disease in Children, J. Pediatr., 2017, 187, 141–146 CrossRef.
- R. Meng, Z. Pei, A. Zhang, Y. Zhou, X. Cai, B. Chen, G. Liu, W. Mai, J. Wei and Y. Dong, AMPK activation enhances PPARα activity to inhibit cardiac hypertrophy via ERK1/2 MAPK signaling pathway, Arch. Biochem. Biophys., 2011, 511, 1–7 CrossRef CAS.
- G. Wang, H. L. Bonkovsky, A. de Lemos and F. J. Burczynski, Recent insights into the biological functions of liver fatty acid binding protein 1, J. Lipid Res., 2015, 56, 2238–2247 CrossRef CAS.
- H. Han, D. Dai, W. Wang, J. Zhu, Z. Zhu, L. Lu and R. Zhang, Impact of serum levels of lipoprotein lipase, hepatic lipase, and endothelial lipase on the progression of coronary artery disease, J. Intern. Med., 2019, 2, 16–20 Search PubMed.
- M. Sekiya, J. Osuga, N. Yahagi, H. Okazaki, Y. Tamura, M. Igarashi, S. Takase, K. Harada, S. Okazaki, Y. Iizuka, K. Ohashi, H. Yagyu, M. Okazaki, T. Gotoda, R. Nagai, T. Kadowaki, H. Shimano, N. Yamada and S. Ishibashi, Hormone-sensitive lipase is involved in hepatic cholesteryl ester hydrolysis, J. Lipid Res., 2008, 49, 1829–1838 CrossRef CAS.
- E. F. Khaleel, G. A. Abdel-Aleem and D. G. Mostafa, Resveratrol improves high-fat diet induced fatty liver and insulin resistance by concomitantly inhibiting proteolytic cleavage of sterol regulatory element-binding proteins, free fatty acid oxidation, and intestinal triglyceride absorption, Can. J. Physiol. Pharmacol., 2018, 96, 145–157 CrossRef CAS.
- K. Begriche, J. Massart, M. A. Robin, F. Bonnet and B. Fromenty, Mitochondrial adaptations and dysfunctions in nonalcoholic fatty liver disease, Hepatology, 2013, 58, 1497–1507 CrossRef CAS.
- M. R. de Oliveira, S. F. Nabavi, A. Manayi, M. Daglia, Z. Hajheydari and S. M. Nabavi, Resveratrol and the mitochondria: From triggering the intrinsic apoptotic pathway to inducing mitochondrial biogenesis, a mechanistic view, Biochim. Biophys. Acta, 2016, 1860, 727–745 CrossRef CAS.
- Z. Ungvari, W. E. Sonntag, R. de Cabo, J. A. Baur and A. Csiszar, Mitochondrial Protection by Resveratrol, Exercise Sport Sci. Rev., 2011, 39, 128–132 CrossRef.
- P. Mukhopadhyay, B. Horváth, M. Rajesh, Z. V. Varga, K. Gariani, D. Ryu, Z. Cao, E. Holovac, O. Park, Z. Zhou, M. J. Xu, W. Wang, G. Godlewski, J. Paloczi, B. T. Nemeth, Y. Persidsky, L. Liaudet, G. Haskó, P. Bai, A. H. Boulares, J. Auwerx, B. Gao and P. Pacher, PARP inhibition protects against alcoholic and non-alcoholic steatohepatitis, J. Hepatol., 2017, 66, 589–600 CrossRef CAS.
- N. G. Larsson, J. Wang, H. Wilhelmsson, A. Oldfors, P. Rustin, M. Lewandoski, G. S. Barsh and D. A. Clayton, Mitochondrial transcription factor A is necessary for mtDNA maintenance and embryogenesis in mice, Nat. Genet., 1998, 18, 231–236 CrossRef CAS.
- H. Qin, X. Zhang, F. Ye and L. Zhong, High-fat diet-induced changes in liver thioredoxin and thioredoxin reductase as a novel feature of insulin resistance, FEBS Open Bio, 2014, 4, 928–935 CrossRef CAS.
- H. Zhang, Y. Chen, Y. Li, L. Yang, J. Wang and T. Wang, Medium-chain TAG attenuate hepatic oxidative damage in intra-uterine growth-retarded weanling piglets by improving the metabolic efficiency of the glutathione redox cycle, Br. J. Nutr., 2014, 112, 876–885 CrossRef CAS.
- H. Zhang, W. Su, Z. Ying, Y. Chen, L. Zhou, Y. Li, J. Zhang, L. Zhang and T. Wang, N-acetylcysteine attenuates intrauterine growth retardation-induced hepatic damage in suckling piglets by improving glutathione synthesis and cellular homeostasis, Eur. J. Nutr., 2018, 57, 327–338 CrossRef CAS.
- K. Cheng, Z. Song, S. Li, E. Yan, H. Zhang, L. Zhang, C. Wang and T. Wang, Effects of resveratrol on intestinal oxidative status and inflammation in heat-stressed rats, J. Therm. Biol., 2019, 85, 102415 CrossRef CAS.
- N. E. Sunny, E. J. Parks, J. D. Browning and S. C. Burgess, Excessive hepatic mitochondrial TCA cycle and gluconeogenesis in humans with nonalcoholic fatty liver disease, Cell Metab., 2011, 14, 804–810 CrossRef CAS.
- Z. J. Xu, J. G. Fan, X. P. Wang and G. L. Wang, Upregulating expressions of hepatic lipopolysaccharide receptors in nonalcoholic steatohepatitic rats, Chin. J. Hepatol., 2006, 14, 49–52 CAS.
- L. P. Han, B. Sun, C. J. Li, Y. Xie and L. M. Chen, Effect of celastrol on toll-like receptor 4-mediated inflammatory response in free fatty acid-induced HepG2 cells, Int. J. Mol. Med., 2018, 42, 2053–2061 CAS.
- T. Sharifnia, J. Antoun, T. G. Verriere, G. Suarez, J. Wattacheril, K. T. Wilson, R. M. Peek Jr., N. N. Abumrad and C. R. Flynn, Hepatic TLR4 signaling in obese NAFLD, Am. J. Physiol.: Gastrointest. Liver Physiol., 2015, 309, G270–G278 CrossRef CAS.
- W. Ren, Y. Xia, S. Chen, G. Wu, F. W. Bazer, B. Zhou, B. Tan, G. Zhu, J. Deng and Y. Yin, Glutamine Metabolism in Macrophages: A Novel Target for Obesity/Type 2 Diabetes, Adv. Nutr., 2019, 10, 321–330 CrossRef.
- A. Mantovani, A. Sica, S. Sozzani, P. Allavena, A. Vecchi and M. Locati, The chemokine system in diverse forms of macrophage activation and polarization, Trends Immunol., 2004, 25, 677–686 CrossRef CAS.
- S. Gordon and P. R. Taylor, Monocyte and macrophage heterogeneity, Nat. Rev. Immunol., 2005, 5, 953–964 CrossRef CAS.
- B. Kim, C. Farruggia, C. S. Ku, T. X. Pham, Y. Yang, M. Bae, C. J. Wegner, N. J. Farrell, E. Harness, Y. K. Park, S. I. Koo and J. Y. Lee, Astaxanthin inhibits inflammation and fibrosis in the liver and adipose tissue of mouse models of diet-induced obesity and nonalcoholic steatohepatitis, J. Nutr. Biochem., 2017, 43, 27–35 CrossRef CAS.
- M. Nakata, S. Yamamoto, T. Okada, D. Gantulga, H. Okano, K. Ozawa and T. Yada, IL-10 gene transfer upregulates arcuate POMC and ameliorates hyperphagia, obesity and diabetes by substituting for leptin, Int. J. Obes., 2016, 40, 425–433 CrossRef CAS.
Footnote |
† Electronic supplementary information (ESI) available. See DOI: 10.1039/d0fo01459a |
|
This journal is © The Royal Society of Chemistry 2021 |